Advanced applications of DNA nanostructures dominated by DNA origami in antitumor drug delivery
- 1Clinical Medical Laboratory Center, Jining First People’s Hospital, Shandong First Medical University, Jining, Shandong, China
- 2College of Traditional Chinese Medicine, Shandong University of Traditional Chinese Medicine, Jinan, Shandong, China
DNA origami is a cutting-edge DNA self-assembly technique that neatly folds DNA strands and creates specific structures based on the complementary base pairing principle. These innovative DNA origami nanostructures provide numerous benefits, including lower biotoxicity, increased stability, and superior adaptability, making them an excellent choice for transporting anti-tumor agents. Furthermore, they can considerably reduce side effects and improve therapy success by offering precise, targeted, and multifunctional drug delivery system. This comprehensive review looks into the principles and design strategies of DNA origami, providing valuable insights into this technology’s latest research achievements and development trends in the field of anti-tumor drug delivery. Additionally, we review the key function and major benefits of DNA origami in cancer treatment, some of these approaches also involve aspects related to DNA tetrahedra, aiming to provide novel ideas and effective solutions to address drug delivery challenges in cancer therapy.
1 Introduction
DNA origami is a new nanotechnology that utilizes numerous techniques to precisely fold DNA molecules into a variety of forms, yielding unique DNA nanostructures with enhanced properties. These structures have extensive applications in biomedical sciences, particularly in drug delivery. Cancer is a disease that develops when, in response to specific factors, normal cells undergo certain transformations that allow them to proliferate without limit and form malignant tumors. The biological mechanisms involved are complex, and malignancy is severe, with a poor prognosis. Currently, there are several types of anti-tumor medications available, including chemotherapy drugs, nucleic acid drugs, and protein drugs. Chemotherapy drugs, which are potent medications, indiscriminately attack both normal and cancerous cells within the body, frequently causing side effects such as bone marrow suppression, cardiac toxicity, and serious digestive and skin rea ctions, all of which significantly harm the patient’s health. While nucleic acid and protein-based biological preparations typically have low cytotoxicity, they are unstable within the body and can quickly be decomposed by endogenous hydrolytic enzymes, as well as potential immunogenicity, limiting their efficacy. Since DNA origami nanostructures (DONs) possess excellent editability, biocompatibility, and in vivo stability, they can be utilized to create DNA nanostructures with a variety of activities and anti-tumor medications. This enables drugs to access cancer cells with greater stability and precision, boosting treatment efficacy and lowering toxicity. Consequently, studying ways to employ DNA origami to address drug delivery issues has become a hot spot in medical research. This review will comprehensively analyze the most recent research advances and development trends of DNA origami in delivering anti-tumor drugs, exploring its role and benefits in cancer treatment, with the aim of providing new ideas and directions for addressing drug delivery issues in tumor therapy more effectively.
2 DNA origami for drug delivery design
2.1 Overview of the DNA origami principle
A DNA molecule is a polymer consisting of two continuous single strands of deoxyribonucleotides that run parallel and in opposite directions. Each strand contains four types of bases, namely, adenine (A), guanine (G), thymine (T), and cytosine (C). The nucleotides combine to complementary pairs A-T and C-G, creating stable links between the strands and giving foundation to DNA’s double helix shape. Because of the selectivity and stability of base pairing, DNA molecules may be folded and assembled, which can then be manipulated by changing the sequence arrangement and template shape to construct complex three-dimensional structures. Using this DNA base pairing theory, professor Rothemund from the California Institute of Technology introduced the concept of DNA origami in 2006 (Rothemund, 2006). By designing and arranging DNA sequences, he successfully created artificial shapes such as triangles and stars. DNA origami is a unique bottom-up approach to constructing nanoscale structures made from DNA in a self-assembling manner. Through nucleic acid sequence hybridization, a long single-stranded DNA, typically the genome of M13 phage, is used as a scaffold, and hundreds of short single-stranded DNAs, or staple strands, are used to form pre-designed nanostructures with specific sizes and shapes. The short DNA strands act like staples on a bookbind, crossing multiple binding domains on the DNA scaffold to create the appropriate 2D or 3D structure. This causes the scaffold folding and twisting from its initial linear configuration into the desired nano-pattern. Finally, the short and long DNA strands are heated in an alkaline solution (Typically, it is a buffer solution consisting of tris acetate buffer with a pH of around 8.), automatically and stably binding to form the initially designed structures. DNA origami presents a promising technique for creating intricate, functional nanostructures for applications in various fields, including medicine and electronics (Jiang et al., 2019; Dey et al., 2021).
To produce a DONs, the initial step usually involves designing the sequence via programmatic software, such ascaDNAno (Douglas et al., 2009), ATHENA (Hyungmin et al., 2020), and Adenita (de Llano et al., 2020), widely utilized nowadays. Once the template strand and auxiliary folding strands are designed, they are mixed in a specific ratio for annealing, followed by subsequent functional modifications and purification of the resulting structure. Over the last 2 decades, scientists have created an array of 2D or 3D DNA folding structures (Figure 1).
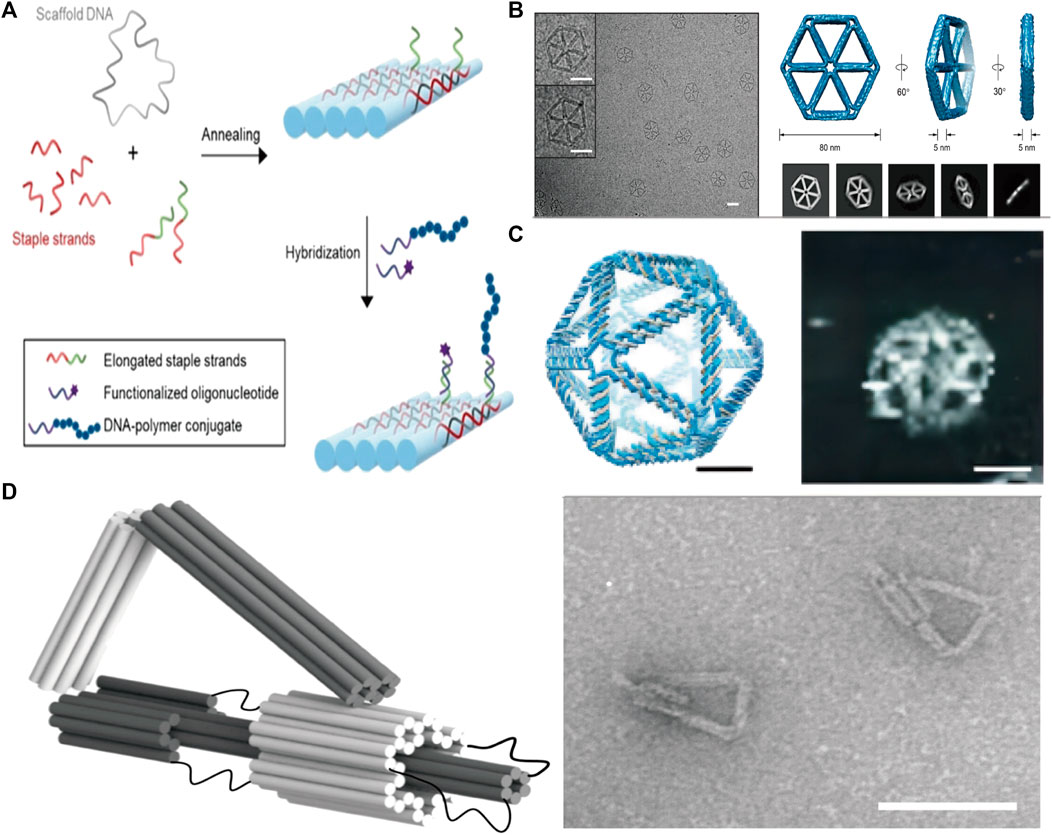
FIGURE 1. (A) Schematic diagram of the principle of DNA origami. Reproduced with permission (Hannewald et al., 2021) Copyright © 2021, John Wiley and Sons. (B) 2D wireframe hexagonal DNA nanostructure made using DNA origami. Reproduced with permission (Wang et al., 2022b) under copyright Creative Commons Attribution 4.0 International License (CC-BY license) (C) 3D wireframe DNA nanostructures in the shape of an icosahedron are formed using DNA origami techniques. Reproduced with permission (Veneziano et al., 2016) Copyright © 2016, The American Association for the Advancement of Science (D) A DNA nano-component with flexible motion capability made using DNA origami. Reproduced with permission (Marras et al., 2015) under copyright Creative Commons Attribution 4.0 International License (CC-BY license).
DNA origami technology is programmable, allowing for the design and manufacturing of DNA nanostructures with features and functions that may be tuned to individual requirements. Drug delivery, sensors, and nanocircuits are just a few examples of the many applications that could benefit greatly from such structures.
2.2 Strategies to construct DONs for drug delivery
Loading drugs onto DONs is a critical step in optimizing drug delivery. The stable structure and specific targeting properties of DONs make them ideal nanocarriers for efficient drug transportation. To load drugs onto DONs, an appropriate method must be selected based on the drug’s characteristics. This can be accomplished by the use of techniques like as intercalation, ligand recruitment, chain modification, hybridization, or encapsulation (Madhanagopal et al., 2018). For instance, the binding of doxorubicin (DOX) to either the G-C base pairs or grooves of the A-T rich regions in the double-stranded DNA can be used to combine DOX with DONs and generate a stable complex (Perez-Arnaiz et al., 2014). For larger-molecule drugs, their corresponding ligands can be chemically linked to DONs to achieve loading functionality. Alternatively, drugs can be covalently connected to component DNA chains and assembled as part of the structure (Liu et al., 2012; Chen et al., 2016; Schaffert et al., 2016). For nucleic acid drugs like cytosine-phosphorothioate-guanine (CpG) and small interfering RNA (siRNA), adding a complementary single-strand extension onto the DONs is suitable (Perez-Arnaiz et al., 2014). In general, selecting the appropriate drug-loading method is crucial in harnessing the full potential of DONs as a nanocarrier for optimal drug delivery results.
Enhancing drug delivery targeting is a critical strategy for developing efficient DON-based drug delivery systems. Traditional anti-tumor drugs, such as chemotherapy agents, frequently induce significant toxic side effects by attacking both malignant and healthy cells. However, designing and fabricating nanocarriers with specific targeting can release drugs only when necessary, reducing the harm to healthy cells. Special DONs can considerably improve targeting efficiency. Rectangular, triangular, or tubular-shaped DONs have shown a preference for accumulating in the kidneys. In particular, rectangular DONs have demonstrated a stronger kidney-protective effect similar to N-acetyl cysteine, which assists in clearing reactive oxygen species (ROS) from the kidneys (Jiang et al., 2018; Wang, 2019). The precise arrangement of interleukin-33 (IL-33) nanoarrays on rectangular DONs enables preferential delivery of IL-33 to the kidneys. Nano-rafts loaded with IL-33 exhibit a preference for accumulating in the kidneys, where they can stay for up to 48 h and continuously release IL-33. This leads to a rapid increase of type 2 innate lymphoid cells (ILC-2) and regulatory T cells (Tregs), facilitating the alleviation of ischemic acute kidney injury (AKI) (Li et al., 2021). Moreover, anchoring cell-specific antibodies, aptamers, peptides, ligands, or receptor-specific proteins on the surface of DONs can enable drug-loaded nanostructures to reach specific regions. For instance, triangular DONs modified with folate have been shown to enhance the targeting of M1 macrophages while helping the transition of pro-inflammatory M1 macrophages to anti-inflammatory M2 macrophages. This results in a reduction of reactive oxygen species (ROS) and nitric oxide (NO) levels, thereby promoting a better anti-inflammatory response (Ma et al., 2022b).
Improving drug stability is crucial for new biologics used against tumors, such as nucleic acid drugs, which are highly susceptible to hydrolytic enzymes, leading to poor stability in vivo. However, DONs as a shell can provide protection against environmental and physiological factors, boosting drug stability and prolonging its lifespan. For example, Lacroix et. alcombining human serum albumin with DONs can slow down the degradation rate of DNA structures in vivo, helping to stabilize siRNAs’ efficacy in cells (Lacroix et al., 2017). Ponnuswamy et al. employed oligolysine conjugated to PEG to shield DNA nanostructures, thus mitigating nucleolytic degradation (Ponnuswamy et al., 2017). Agarwal et al. utilized cationic poly (ethylene glycol)-polylysine block copolymer micelles as a robust and cost-effective strategy for safeguarding DONs (Agarwal et al., 2017). Gerling et al. enhanced the resistance of DNA origami structures against nucleases by inducing thymine dimer formation through ultraviolet irradiation. Some studies have indicated that encapsulating DNA origami within protective silica shells via sol-gel chemistry contributes to the stability of DONs (Liu et al., 2018b; Nguyen et al., 2019; Wassermann et al., 2023). Similarly, it has been suggested that mineralization of DONs surfaces with calcium phosphate enhances resistance against degradation by nuclease (Wu et al., 2021a).
Moreover, some studies have shown that packaging proteins and peptides on the surface of DONs (Cho et al., 2014; Auvinen et al., 2017; Wang et al., 2020; Seitz et al., 2022) and increasing cross-linking quantity (Xin et al., 2022) can effectively prevent nucleases from degrading it, hence increasing nuclease resistance, regulating drug release rate, and improving DONs stability. Additionally, as shown by the findings of Rajendran et al., reinforcing linkages can enhance the thermal stability of DONs, which is important for maintaining stable release of thermosensitive anti-tumor drugs in vivo (Rajendran et al., 2021) (Figure 2).
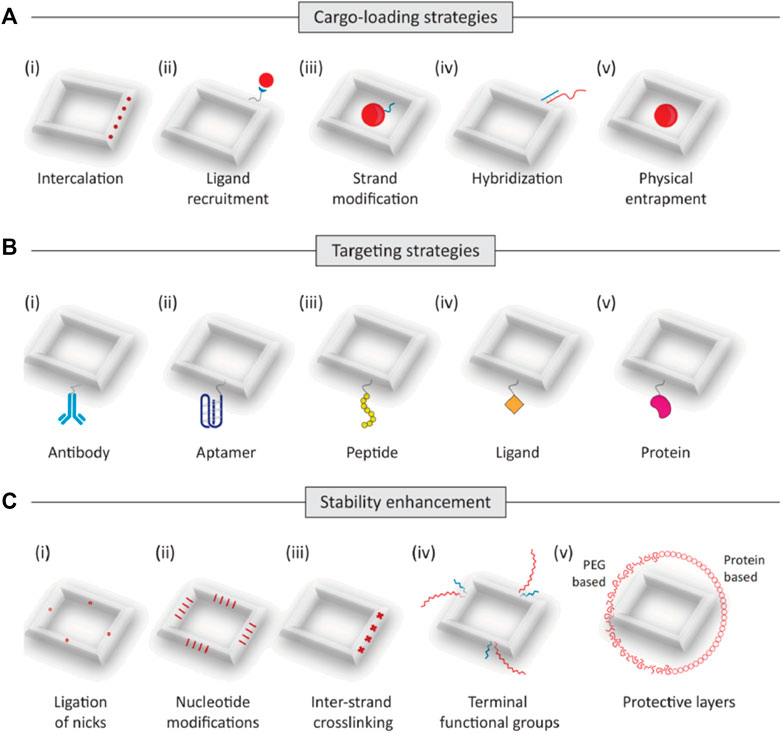
FIGURE 2. Trategies to construct DONs for drug delivery. (A) The main strategies of drug loading for DONs(as shown in i-v): intercalation, ligand recruitment, trand modification, hybridization, and entrapment. (B) Targeting strategies. Drug-loaded DONs can be designed to reach specified locations by using cell-specific antibodies, aptamers, peptides, ligands, or receptor-specific proteins (as shown in i-v). (C) Strategies to improve biostability. Modifications to improve the stability of DNA nanostructures include ligation of nicks, (ii) nucleotide modifications, (iii)inter-strand crosslinking, (iv) functional groups such as hexaethylene glycol and hexane diol, and (v) polyethylene glycol (PEG)-based or protein-based protective layers.
3 Anti-tumor drug delivery dased on DNA origami
3.1 Targeted drug delivery
Using the DNA origami technique to construct nanostructures can also endow them with more functions beyond basic drug delivery. For instance, several medications, such as a combination of anti-tumor medications and adjuvants or different types of anti-tumor drugs, can be loaded onto DONs, including, to achieve comprehensive treatment and improve therapeutic efficacy while reducing side effects. Furthermore, employing DONs to load multi-functional nanocarriers can simultaneously support drug delivery, imaging, and therapy, which has significant implications for improving treatment outcomes (Lu et al., 2019; Zeng et al., 2021).
Targeted therapy is a sort of treatment that uses medications or other substances to identify and attack specific types of cancer cells while causing minimal damage to normal cells. Clinical practice has revealed that many drugs often suffer from shortcomings, such as multiple target points, high cell toxicity, and poor in vivo stability, which frequently lead to various side effects and poor therapeutic outcomes (Li et al., 2022). However, with the construction of suitable DNA nanostructures using DNA origami, drugs can be loaded onto the DNA folding vehicle. This reduces in vivo loss, delivers precisely to target cells, accurately kills specific targets without hurting normal cells, and diminishes toxic side effects while enhancing efficacy. Many studies have shown that DNA origami particles loaded with drugs can achieve targeted drug delivery, particularly for chemotherapeutic drugs, nucleic acid drugs, protein, and peptide drugs (Udomprasert and Kangsamaksin, 2017; Zhang et al., 2023).
DNA origami allows DNA molecules to be folded into various nanoscale structures, such as nanotubes and nanoboxes, which has notable benefits for targeted drug delivery. For instance, these nanoscale structures are highly controllable. By adjusting the folding sequence and length, the shape and size of the nanostructure can be precisely controlled, enabling accurate targeting and delivery of drugs (Yan et al., 2015). Additionally, DNA origami exhibits excellent selectivity and can target specific cells or tissues by designing DNA sequences with specific affinities, thus allowing for precise targeting of drugs (Li et al., 2013). Furthermore, DNA origami has a high drug-carrying capacity, efficiently encapsulating and releasing multiple types of drugs by adjusting spatial structure and size. Finally, using naturally occurring DNA molecules in the body results in extremely biocompatible nanostructures, reducing adverse impacts on the human body (Yan et al., 2015; Kumar et al., 2016).
As a result, DNA origami has become widely used in targeted drug delivery, effectively improving the efficiency and accuracy of drug delivery while also opening up new avenues for drug treatment research. Research has indicated that the process of DONs internalization by tumor cells involves the mechanism of cell membrane engulfment and the formation of endocytic vesicles. The internalization process of DONs includes four primary steps, adsorption, endocytosis, early endosome formation, and late endosome formation. During adsorption, DONs interact with scavenger receptors on the cell membrane surface, tightly adhering to the cell membrane. The cell membrane then undergoes stretching movements and wraps around DONs, forming an endocytic vesicle. Subsequently, the endocytic vesicle forms small vesicles on the cell membrane to create an early endosome, which fuses with the endoplasmic reticulum, transforming into a late endosome or lysosome, and then gets decomposed (Wang et al., 2018) (Figure 3). In fact, the uptake and intracellular localization mechanisms will be highly structure and coating dependent, different types of DONs, along with their distinct modifications, have a substantial impact on cellular uptake (Balakrishnan et al., 2019; Chiu et al., 2019). Currently, there is no consensus regarding the cellular uptake process of DONs, and further research is needed to shed light on this matter.
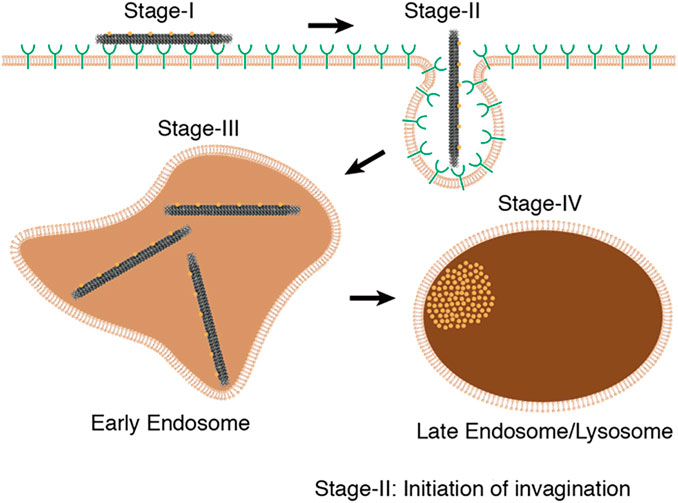
FIGURE 3. The internalization process of DONs includes four primary steps, including adsorption, endocytosis, early endosome formation, and late endosome formation. Reproduced with permission (Wang et al., 2018) Copyright © 2018, American Chemical Society.
3.1.1 Chemotherapy drugs
Chemotherapy is a widely used drug treatment method in cancer therapy, with drugs such as doxorubicin, daunorubicin, metal complexes, fluorouracil, vincristine, paclitaxel, and others commonly utilized. These small molecule drugs enter cells and interfere with the normal cell cycle, promoting cell death and killing cancer cells; however, they also cause multiple and severe side effects due to their broad action targets and strong cytotoxicity against both normal and cancer cells.
DOX is a type of anthracycline drug, commonly used in chemotherapy. It achieves its therapeutic effect by primarily promoting cancer cell apoptosis through the inhibition of DNA synthesis. DOX has a strong affinity for binding to DNA and can be stably loaded onto DONs (Ijas et al., 2021). This composite structure of DONs-DOX exhibits high efficacy in targeting cancer cells and inducing stronger cytotoxicity than free DOX. Additionally, it promotes cancer cell apoptosis while limiting normal cell damage, reducing toxicity and side effects. Zhao et al. investigated the use of DONs-DOX to intervene in three different breast cancer cell lines, MDA-MB-231, MDA-MB-468, and MCF-7. The results showed that DONs-DOX exhibited higher cytotoxicity and a lower intracellular clearance rate compared to free DOX, thereby demonstrating its effectiveness in promoting the death of breast cancer cells (Zhao et al., 2012). Palazzolo et al. utilized compact short-tube DONs to load DOX liposomes and investigated their efficacy in breast cancer cell lines and mouse models. DONs-DOX exhibited high stability, biocompatibility, and improved anti-tumor efficacy and biological distribution of DOX in tumor-bearing mice. Additionally, it was observed to reduce bone marrow toxicity and evade immune system recognition (Palazzolo et al., 2019). Zhang et al. loaded DOX into triangular DONs and demonstrated significant anti-tumor efficacy in a mouse model of breast cancer without any observed systemic toxicity (Zhang et al., 2014) (Figure 4). Han et al. modified DONs with MUC1 adaptors to enhance the intracellular uptake efficiency of MCF-7 breast cancer cells while reducing the uptake efficiency of normal cells. DONs-DOX was found to demonstrate higher systemic safety and lower systemic toxicity compared to free DOX, making it more effective in inhibiting breast cancer cell proliferation (Han et al., 2018). Moreover, it exhibited significant cytotoxicity against conventional human breast cancer cells MCF-7 and doxorubicin-resistant cancer cells, inducing the reversal of the resistant phenotype and enhancing the cell-killing activity against doxorubicin-resistant MCF-7 cells (Jiang et al., 2012). Triple-negative breast cancer (TNBC) is a subtype of breast cancer that lacks expression of Estrogen Receptor (ER), Progesterone Receptor (PR), and Human Epidermal growth factor Receptor 2 (HER-2). Studies have shown that overexpression of FOLR1 plays an important role in the occurrence and development of TNBC (Necela et al., 2015; Song et al., 2016; Cheung et al., 2018). Pal et al. utilized Rothemund’s original triangle DNA origami to enable targeted delivery of DOX to FOLR1 overexpressing cells in TNBC. This approach reduced the side effects of DOX treatment while decreasing the required dose of DOX needed to kill cancer cells by approximately 31-fold (Pal and Rakshit, 2021). Unida et al. functionalized folate and connected the AS1411 aptamer to DONs loaded DOX, exhibiting more than a 231% increase in cytotoxicity against MDA-MB-51 cells compared to free doxorubicin. Additionally, they demonstrated that the bimodal nanocage loaded with doxorubicin helped overcome drug resistance by more efficiently entering triple-negative breast cancer cells (Unida et al., 2022). Furthermore, DOX often faces challenges such as rapid clearance and non-specific distribution, leading to off-target effects. To address this issue, prodrugs have been developed. They are a class of non-active agents that exert the pharmacological effects of the parent drug through site-specific biotransformation. The design of prodrugs targeting unique molecular defects in cancer cells (e.g., overexpression, mutation) is advantageous in improving pharmacokinetics and reducing off-target effects. However, many prodrugs involve systemic prodrug activation by non-specific stimuli (such as esterases, phosphatases, and lysosomal acidification), which may result in severe adverse reactions (Amly and Karaman, 2016). He et al. constructed rectangular DNA origami nanostructures of an amsacrine prodrug with tumor-targeting specificity enzyme (NQO1), which showed therapeutic selectivity towards NQO1-overexpressing MCF-7 cells and healthy L02 cells (He et al., 2023). This approach retained the characteristics of low clearance efficiency and high off-target effects of the amsacrine prodrug while having lower risk of side effects, demonstrating innovation and providing an effective strategy for precision cancer therapy. In addition to breast cancer, the DONs-DOX complex based on DNA origami technology has shown great potential in improving drug efficacy against colon cancer, prostate cancer, and ovarian cancer (Chang et al., 2011; Taghdisi et al., 2018; Zeng et al., 2018; Ge et al., 2020; Li et al., 2020; Yao et al., 2020; Yaghoobi et al., 2022; Singh and Deshmukh, 2023).
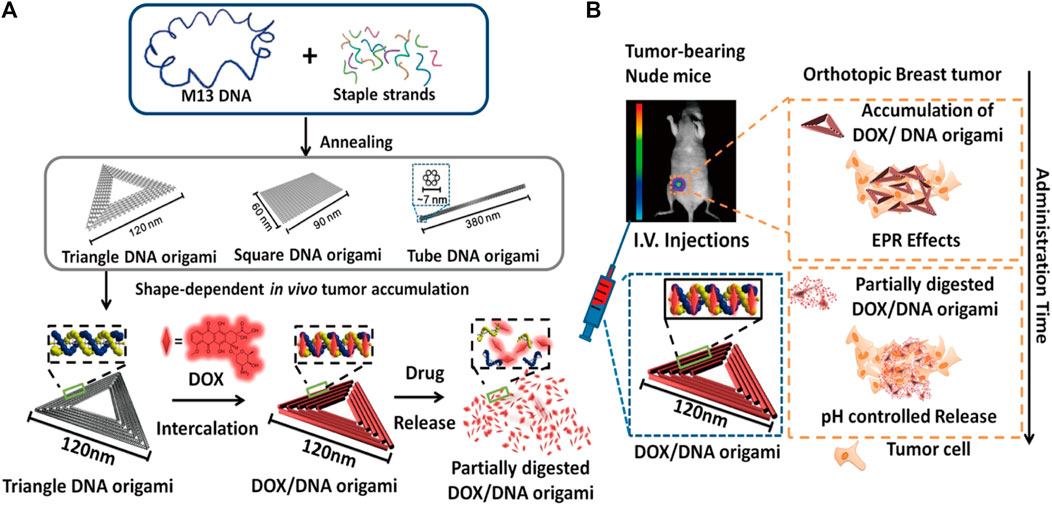
FIGURE 4. Diagram illustrating the use of triangular DONs for targeted delivery of DOX. Reproduced with permission (Zhang et al., 2014) Copyright © 2014, American Chemical Society. (A) Triangular, square, and tubular DONs were fabricated using DNA origami. Among them, triangular DONs exhibited the highest accumulation in tumors and were used for DOX loading. (B) Tail vein injection of DONs-DOX allowed them to enter the mouse breast tumor through blood circulation, accumulate in cancer cells, and eventually kill them.
Metal complexes are coordination polymers formed by the coordination bond between a metal atom and ligands, exhibiting diverse composition and tunable structure. They have shown tremendous potential in anti-tumor applications. Some examples of metal complexes include Platinum (Pt), Ruthenium (Ru), Rhenium (Re), Osmium (Os), Rhodium (Rh), Copper (Cu), Nickel (Ni), Zinc (Zn), Titanium (Ti), etc (Sponer et al., 2006; Deubel and Chifotides, 2007; Galindo-Murillo et al., 2012; Alvarado-Soto and Ramirez-Tagle, 2015; Sayin and Ungordu, 2018; Haleel et al., 2019). Platinum-based anti-cancer drugs, including cisplatin, carboplatin, oxaliplatin, and other metal complexes, are widely used in cancer treatment. These drugs belong to a class of non-specific cell cycle drugs that form Pt-DNA adducts through hydration dissociation with the DNA structure after entering tumor cells. This mechanism leads to tumor cell death or apoptosis, thereby producing an anti-cancer effect. Researchers have designed a dodecahedral DNA nanocage connected by telomerase primers and telomere repeat sequences. Platinum nano-drugs were encapsulated in the dodecahedron, and when entering tumor cells, the telomerase enzyme precisely released the platinum nano-drugs from the cage, targeting and killing tumor cells while enhancing the therapeutic effect against cisplatin-resistant tumors and reducing toxicity to normal tissues (Ma et al., 2018). In recent years, research has shown that ruthenium complexes have ligand exchange kinetics similar to platinum-based anti-tumor drugs, low toxicity, and high selectivity, making them a very promising therapeutic agent with the potential to become a new class of metal complex anti-cancer drugs widely used in clinical practice (Brabec and Novakova, 2006; Thangavel et al., 2017). Researchers have used tetrahedral nanostructures to load Ru polypyridine complexes, which effectively enhanced the specific cellular uptake and cytotoxicity of HepG2 cells, induced ROS-mediated cell apoptosis, and in nude mouse models, the nano system specifically accumulated at the tumor site, reducing damage to the liver, kidney, lung, and heart functions of the mice (Huang et al., 2016).
In addition to DOX, other types of chemotherapy drugs have also been reported to be loaded onto DONs for drug delivery purposes. For instance, 5-fluorouracil (5-FU) and 5-fluoro-2′-deoxyuridine (FdUrd), which are effective against digestive tract cancer and other solid tumors, have been loaded onto DONs with attached cholesterol on the surface to enhance cellular uptake. Compared to traditional 5-FU and FdU, FdUrd-functionalized DNA nanomaterials display enhanced cell toxicity and a higher ability to induce apoptosis of colorectal cancer cells (Jorge et al., 2018). Similarly, mitoxantrone (MX), an anthracycline anti-cancer drug used in chemotherapy for hematological malignancies, has been loaded onto rod-shaped DONs to improve drug efficacy and overcome multi-drug resistance mediated by efflux pumps in leukemia cells at clinically relevant drug concentrations (Halley et al., 2016).
As mentioned earlier, traditional chemotherapy drugs cause unnecessary toxicity damage to healthy cells, and their dispersion in various tissues and organs through the bloodstream leads to problems such as insufficient drug efficacy, difficulty in concentration, and striping (Li et al., 2022). DONs manufactured using DNA origami technology can be customized and designed to produce highly specific targeted carriers against tumor cells, achieving precise drug delivery and localized targeted therapy. With molecular beacons on their surfaces, these highly specific DONs can bind to specific receptors on tumor cell surfaces, facilitating targeted recognition and drug delivery to the interior of tumor cells (Table 1).
3.1.2 Nucleic acid drugs
Malignant tumors often result from multiple oncogenes’ activation and tumor suppressor genes’ inactivation. Nucleic acid drugs, such as CpG, siRNA, and antisense oligonucleotides (ASO), directly regulate the expression of specific genes associated with malignant tumor proteins at the genetic level. These drugs offer several advantages over traditional anti-tumor drugs, including simple sequence design, easy synthesis and modification, strong target specificity, a clear mechanism of action, a wide therapeutic range, and long-lasting efficacy. Consequently, they have become increasingly popular in anti-tumor treatment (Zou et al., 2017; Subhan and Torchilin, 2019; Wu et al., 2021b; Xu et al., 2021b; Meng et al., 2022; Noriega-Rivera et al., 2022; Revenko et al., 2022).
Nucleic acid drugs are composed of independent DNA or RNA sequences that have significant potential in clinical applications. However, their use is limited due to poor stability in vivo, low cellular uptake efficiency, and the potential for immune reactions (Hu et al., 2019). To overcome these limitations, nucleic acid drugs can be loaded onto DONs, providing numerous benefits (Roberts et al., 2020). The natural affinity between nucleic acid drugs and DNA makes them easy to load onto DONs. DONs-based nucleic acid drug delivery systems deliver several advantages, including protection from degradation by avoiding direct exposure to nucleases. Moreover, the outer layer of the DNA nanostructure effectively blocks premature degradation, enhancing their stability in vivo (Liu et al., 2012; Jiang et al., 2019). Modified DONs also improve the uptake efficiency of nucleic acid drugs in the target tissue, allowing accumulation in the surrounding tissues of the tumor (Kumar et al., 2016). Additionally, modification with peptides, aptamers, or antibodies enhances the specific binding of nucleic acid drugs to cancer cell receptors, thus improving their targeting ability (Lee et al., 2012). Lastly, the addition of DONs increases the molecular weight of nucleic acid drugs, making them less susceptible to rapid clearance (Lee et al., 2012; Kumar et al., 2016). This prolongs their half-life in vivo and allows for a prolonged therapeutic effect (Liu et al., 2012; Kumar et al., 2016). By overcoming the limitations of traditional nucleotide-based drugs, DONs-based nucleic acid drug delivery systems offer enormous promise in anti-tumor treatment and patient outcomes.
Cancer cells can evade recognition and attack by the immune system, which significantly contributes to their sustained survival and proliferation in vivo. CpG drugs containing non-methylated CpG as the core sequence provide an effective solution to this problem. These drugs contain multiple human immune-stimulating sequences and have strong immune-stimulating activity (Krieg, 2002; Chen et al., 2021). After entering immune cells, they promote the production of immune-related factors to clear immune-escaped cancer cells. Using DNA origami technology, various DONs such as tetrahedrons, multi-legged animals, DNA nanotubes, and wireframes can be fabricated, and CpG can be loaded onto these structures to enhance its resistance to degradation by nucleases in serum. This improves its ability to enter macrophages and dendritic cells. Once inside macrophages, CpG sequences are recognized by Toll-like receptors, and downstream signaling pathways induce immune stimulation. This promotes high levels of secretion of pro-inflammatory cytokines such as tumor necrosis factor-alpha (TNF-α), interleukin-7 (IL-7), and interleukin-12 (IL-12), strengthening the immune system’s surveillance and clearance of cancer cells (Rattanakiat et al., 2009; Li et al., 2011; Schuller et al., 2011; Mohri et al., 2012a; Mohri et al., 2012b; Ouyang et al., 2013; Ouyang et al., 2014; Qu et al., 2017; Du et al., 2022). To further enhance the immune response to cancer cells, Kang et al. designed a self-assembling nanoplatform using DNA origami technology that contains nitrosylated CD4+ T cell epitopes and CpG (Kang et al., 2023). This adjuvant CpG explicitly activates Toll-like receptor 9 and promotes the presentation of new antigens on dendritic cells, starting and prolonging new antigen-specific CD8 T cell responses, and significantly inhibiting tumor growth. Similarly, Comberlato et al. arranged active CpG motifs on a DNA origami disk at the nanoscale (Comberlato et al., 2022). When the CpG motif was located 7 nm away from Toll-like receptor 7, it could better combine with RAW 9.264 macrophages, achieving stronger immune activation. Using DNA origami technology to deliver CpG drugs, a promising strategy has been developed to stimulate the immune system to fight against cancer cells.
siRNA is a unique type of double-stranded RNA structure that can trigger the degradation of target sequences by binding completely and rendering the Argonaute-2 protein inactive. This process effectively inhibits the translation of specific genes, leading to the suppression of gene expression and modification of disease effects. One promising strategy for the targeted delivery of siRNA for cancer treatment involves using DONs as efficient delivery carriers, which can be customized through surface modifications to selectively target cancer cells and silence malignant tumor-specific protein-related genes (Lee et al., 2012; Chen et al., 2015). In earlier studies on DNA tetrahedron research, Han et al. utilized DNA tetrahedra as a foundation to construct a novel DNA-based nanogel for intracellular siRNA delivery. This approach successfully protected siRNA molecules and facilitated significant gene silencing upon cellular uptake. The developed nanogel exhibited potential applications in anti-tumor therapies and also provided valuable insights for utilizing DNA origami techniques in the delivery of nanostructures for siRNA delivery (Xue et al., 2019). The anti-apoptotic protein Bcl-2 plays a critical role in both cancer development and drug resistance (Zhang et al., 2021). To address this challenge, Rahman et al. developed rectangular and tubular DNA nanocarriers of varying sizes for delivering targeted siRNA against Bcl-2. Their studies demonstrated significant inhibition of tumor progression associated with Bcl-2 overexpression, both in vitro and in vivo. In summary, targeting Bcl-2-related genes using siRNA-loaded DONs represents a promising therapeutic strategy for treating tumors (Rahman et al., 2017).
ASOs are single-stranded oligonucleotide molecules that can bind to complementary target mRNA through base-pairing principles in the presence of ribonuclease H1. This leads to inhibition of the expression of target genes and regulation of protein expression upon entering cells. Recent studies have demonstrated that ASOs hold great promise for inhibiting the development of ovarian cancer (Wang et al., 2022d), colorectal cancer, lung cancer (Shen et al., 2021), and hepatocellular carcinoma (Ma et al., 2022a) while also having potential applications in immune therapy for tumors (Revenko et al., 2022). Moreover, ASOs carried by DONs exhibit higher stability and safety (Long et al., 2021), and can deliver TGF-β1 mRNA-targeting ASOs to liver cells to combat hepatic fibrosis (Kim et al., 2022). The p53 tumor suppressor gene serves as a common tumor-suppressing gene, and its inactivation plays a crucial role in tumor formation. Mutated p53 helps with cancer proliferation and metastasis (Kim et al., 2019; Hu et al., 2021). To address this issue, Wu et al. developed an innovative approach to fold the ASO encoding the anti-tumor gene (p53) and its complementary antisense nucleotide chain into a DNA origami structure. This structure was then coated with a lipid envelope on its surface and modified with tumor-targeted groups. As a result, this structure could specifically target cancer cells, efficiently penetrate the cancer cell membrane, and express the ASO and complementary antisense nucleotide chain inside the cell. This led to a significant upregulation of p53 protein in tumor cells, promoting cancer cell apoptosis, and ultimately treating malignant tumors (Wu et al., 2023) (Figure 5) (Table 2).
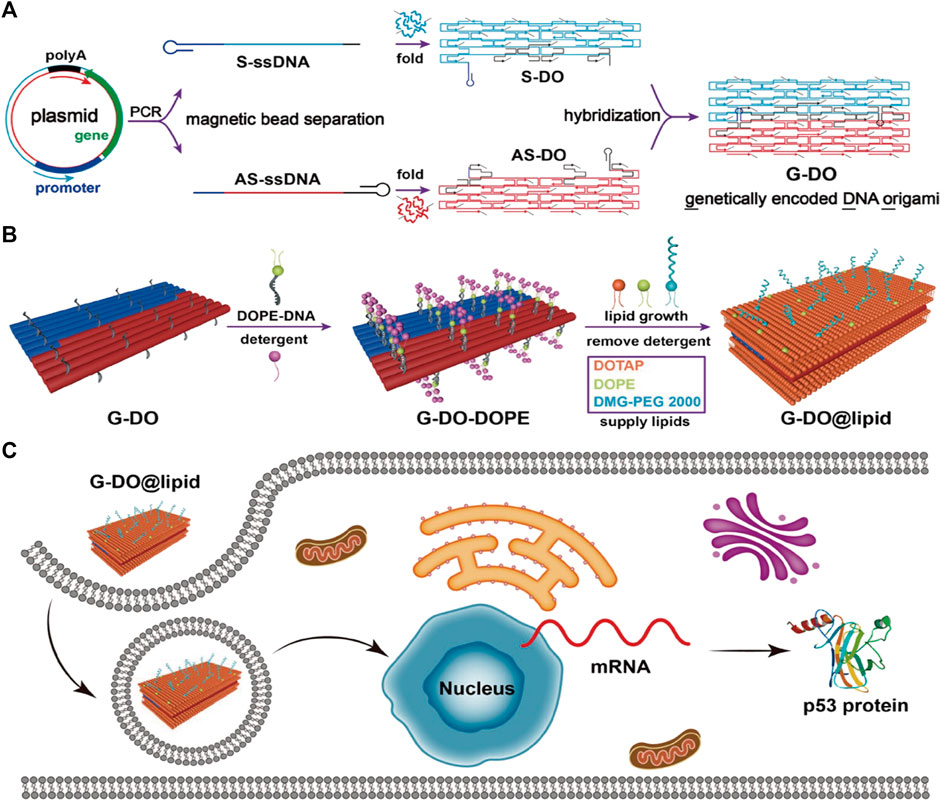
FIGURE 5. DNA origami is used to make nanostructures carrying gene drugs. Reproduced with permission (Wu et al., 2023) Copyright © 2023, American Chemical Society. (A) Folding the ASO encoding the anti-tumor gene (p53) and its complementary antisense nucleotide chain into a DNA origami structure. (B) Surface modification of the above-mentioned DONs. (C) The above-mentioned DONs enter the cell nucleus and upregulate p53 protein expression.
3.1.3 Peptides and protein drugs
Peptide and protein drugs have emerged as a major focus in anti-tumor therapy, encompassing various biochemical molecules such as antibodies, cytokines, receptor molecules, and enzymes. These drugs offer several advantages owing to their high specificity, multifunctionality, and good biocompatibility. Unlike nucleic acid drugs and chemotherapy drugs, peptide and protein drugs do not cause toxicity in normal cells or induce permanent or random changes in gene sequences. Despite these benefits, peptide and protein drugs also suffer from some limitations. Firstly, they exhibit poor stability both inside and outside the body, rendering them vulnerable to enzymatic degradation and other environmental factors, resulting in a short biological half-life. Secondly, peptides and proteins may elicit an immune response and pose a risk of allergic reactions. Thirdly, a significant first-pass effect occurs in the liver and gastrointestinal tract, leading to low effective concentrations within the body. Fourthly, most proteins have large relative molecular weights, complex structures, and poor membrane penetration under neutral pH conditions, resulting in low bioavailability. However, DNA origami offers a promising solution to overcome these limitations. By preparing DONs, researchers can achieve breakthroughs in maintaining the biological activity, targeting, controlled release, and prolonged duration of action of peptide and protein drugs.
Peptides and proteins have been precisely and conveniently assembled onto DONs (Zhou et al., 2018; Brown et al., 2022). To enhance the targeting of DON-protein structures, researchers have suggested using DONs and zinc fingers (ZnFs) to assemble targeted protein delivery nanoparticles (Ryu et al., 2018). Y-shaped DNA origami modified with ZnFs can deliver PTEN tumor suppressor proteins into cells, regulating kinase signaling pathways and inhibiting cancer cell growth (Ryu et al., 2020). Tumor necrosis factor (TNF) is involved in regulating the cell cycle of tumor cells. DONs modified with TNF-related apoptosis-inducing ligands can promote apoptosis of human breast cancer cells, providing a new strategy for ineffective TNF superfamily activation methods based on ligands and antibodies (Wang et al., 2021a). Ma et al. utilized flat rectangular DONs loaded with tumor necrosis factor-related apoptosis-inducing ligands (TRAIL) trimer to induce apoptosis of tumor cells. Ribonuclease (RNase) A is an RNA hydrolyzing enzyme (Ma et al., 2023). Zhao et al. added RNaseA and MUC1 adaptors to rectangular DNA origami nano-sheets, enabling DONs to specifically enter tumor cells and hydrolyze RNA inside cancer cells, inhibiting tumor progression. Caspase-9 is a pro-apoptotic protein (Zhao et al., 2019). Rosier et al. combined it with DONs to enhance the regulation of cell apoptosis. Additionally, overexpression of epidermal growth factor (EGF) and adrenaline receptors (Eph) promotes cancer development (Rosier et al., 2020). By rearranging the distribution and proportional quantity of corresponding binding ligands on the surface of DONs, cancer cell transcription and invasion can be effectively inhibited (Huang et al., 2019; Verheyen et al., 2020). Berger et al. arranged Fas ligands on DNA origami sheets into hexagonal arrays with a molecular spacing of 10 nm, revealing their high capability to induce cellular apoptosis. This finding holds great promise for applications in the field of tumor therapy (Berger et al., 2021). Wang et al. developed a DNA origami template-adaptor nanoarray (DOTA) that enables precise programming of receptor tyrosine kinase (RTK) oligomerization at the ligand-receptor interface. The DOTA chip allows for precise control over the activation levels of RTK signaling by defining valency, distribution, and stoichiometry of the receptor tyrosine kinases. This manipulation promotes the transition from epithelial to mesenchymal-like cells and regulates cellular behavior (Wang et al., 2022a).
One effective approach for treating tumors involves inducing thrombus formation to obstruct the tumor’s blood supply by activating thrombin activity (Li et al., 2019b). However, thrombin is a non-specific agent, and its release outside of tumor vessels can result in systemic thrombosis, which may lead to serious conditions such as pulmonary embolism, myocardial infarction, cerebral infarction, or even pose a risk to life. Nevertheless, the development of DNA nanorobots utilizing DNA origami provides a promising solution for the targeted delivery of thrombin. These DNA nanorobots can carry thrombin and release it solely within tumor-related blood vessels upon detecting specific tumor-related proteins. This targeted delivery method ensures that thrombin only induces thrombus formation where needed, effectively suppressing tumor growth while reducing the potential risks associated with systemic thrombosis (Li et al., 2018; Li et al., 2019a; Singh and Deshmukh, 2023).
Immunotherapy, which utilizes the body’s immune system, is a major focus of cancer treatment research. DNA origami holds immense potential in the field of immunotherapy by enhancing immune stimulation activity and improving targeting (Ouyang et al., 2017). Additionally, DNA origami plays a critical role in adoptive immunotherapy and cancer vaccines. Sun et al. optimized DNA origami structures for use in nanoscale artificial antigen-presenting cells (aAPCs), which have efficient signal delivery and great potential in adoptive cell therapy. By anchoring co-stimulatory ligands that target CD28 antibodies and T cell receptor (TCR) ligand peptide-major histocompatibility complex (pMHC) on its three vertices and three edges, their DNA-origami-based aAPCs demonstrated higher tumor growth inhibition in adoptive immunotherapy (Sun et al., 2022) (Figure 6). In another study, Liu et al. assembled molecular adjuvants and antigen peptides into the lumen of tubular DNA nanostructures (Liu et al., 2021). These structures were activated in subcellular environments to trigger T cell activation and cancer cell toxicity, which not only reduced tumor size but also prevented recurrence. This approach presents a promising avenue for the development of cancer vaccines. Kern et al. developed a DNA-origami-based phagocytic system carrying FcγR receptor ligands, which enhanced macrophage phagocytosis by regulating these ligands’ density on the DNA origami structure (Kern et al., 2021). This has significant implications for the design of immunotherapies, as macrophages play a crucial role in clearing pathogens and diseased cells through Fcγ receptors (FcγRs) driven regulation. Moreover, PD-1 is an inhibitory receptor expressed on activated T cells that suppresses T cell signaling and effector function, making it a current research hotspot in cancer immunotherapy. FANG et al. found that DNA origami plates modified with CD3 and CD28 activating antibodies (FS-α-CD3-CD28) induced strong T cell activation. The spatial organization of PD-L1 determines its ability to regulate T cell signaling, and this finding may guide the development of future nanomedicine-based immune modulation therapies (Fang et al., 2021) (Table 3).
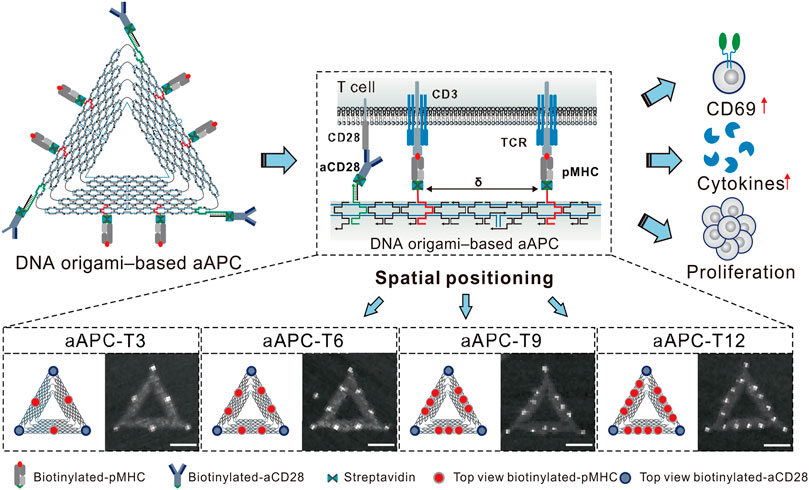
FIGURE 6. The aAPCs designed based on DNA origami were used to regulate T cell activation. Reproduced with permission (Sun et al., 2022) under copyright Creative Commons Attribution 4.0 International License (CC-BY license).
3.2 Multifunctional drug delivery system
A drug delivery system is considered multi-functional if it can perform multiple functions. DNA origami, due to its highly customizable nature, can be utilized to create carriers that can contain numerous drugs with different therapeutic effects. This enables a comprehensive treatment approach encompassing various methods such as photoacoustic diagnosis, photothermal therapy, chemotherapy, immunotherapy, gene therapy, and more. Hence, DNA origami is an essential component in the advancement of multi-functional delivery systems. Recently, several successful attempts have been made to utilize DNA origami in the production of multi-functional drug delivery carriers.
Drug therapy is a crucial approach to treating cancer. However, the problem of drug resistance has become increasingly common among cancer patients as they undergo treatment over extended periods. This resistance limits the cytotoxic effect of anti-cancer drugs on cancer cells, making it one of the primary reasons for the failure of cancer treatment. Consequently, overcoming cancer cell resistance is a significant challenge in modern clinical work. Gold nanomaterials (AuNPs) have unique photoelectric, physical and chemical properties, and excellent biocompatibility, making them an attractive option in current cancer treatments (Boisselier and Astruc, 2009). They are frequently utilized as drug carriers to deliver drugs into tumor cells and kill cancer cells through photothermal effects (Alle et al., 2022). A new method of treating resistant tumors involves loading gold nanorods and drugs onto DNA origami nanostructures (DONs) for targeted delivery to resistant tumor cells. By combining chemotherapy drugs and targeted drugs, they can work together to kill resistant tumor cells (Kong et al., 2016; Song et al., 2017; Zhang et al., 2017; Jin et al., 2019). To address deeper cancer cells in tumor tissues, Gu et al. combined DOX, gold nanoparticles (GNP), and tetrahedral DNA nanostructures (TDNs) (Gu et al., 2022). The structure was designed to cause damage to tumor tissue at different depths based on changes in pH value. Initially, the overall nanostructure accumulates in tumor cells and works together. When the pH value of the tumor microenvironment is around 6.5, the TDN transforms from a double helix into a triple structure through DNA sequence connection, separates from GNP, penetrates deep into the tumor stroma, and internalizes into cells using GNP photothermal therapy to kill the tumor. Finally, in the acidic lysosome with a pH of 5.0, TDN releases DOX by forming an i-motif structure to kill deep cancer cells using DOX toxicity.
DONs, in addition to gold nanoparticles, have the potential to treat drug-resistant tumors when used in combination with chemotherapy drugs and nucleic acid drugs. Co-delivery of Dox and two different ASOs using DONs by Pan et al. effectively inhibited the expression of drug-resistant proteins B-cell lymphoma 2 (Bcl2) and P-glycoprotein (P-gp), thus enhancing the therapeutic effect on chemoresistant cells (Pan et al., 2020). In another study, Xu et al. loaded siRNA, DOX, and AuNRs onto octahedral DONs as carriers, downregulating the expression of connective tissue growth factor (CTGF) and heat shock protein 72 (HSP72) in drug-resistant cancer cells. This made cancer cells sensitive to both chemical drugs and thermal therapy (Xu et al., 2021a) (Figure 7). The multifunctional DONs constructed by Liu et al. delivered siRNA and DOX into MCF-7R cells through active targeting and controlled release, synergistically inhibiting tumor growth without significant systemic toxicity (Liu et al., 2018a). Wang et al. also used DONs to deliver siRNA and DOX, which effectively enhanced cell toxicity and inhibited tumor growth without causing systemic toxicity (Wang et al., 2021b). Additionally, Wu et al. embedded platinum-based drug 56MESS and anti-EGFR nanobody into DNA tetrahedral structures (Wu et al., 2019). This combination medication targeted and killed cancer cells with platinum-based drugs while blocking EGFR-related signal transduction in cells with high EGFR expression, achieving multi-drug combination therapy.
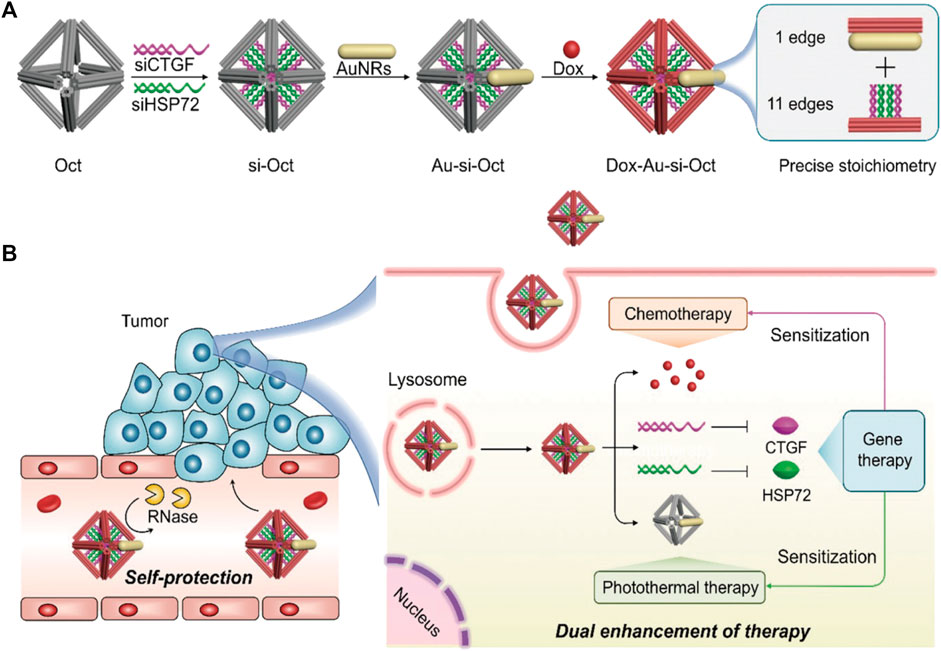
FIGURE 7. Octahedral DNA nanostructures fabricated by DNA origami are utilized for multifunctional drug delivery. Reproduced with permission (Xu et al., 2021a) Copyright © 2021, John Wiley and Sons. (A) The siRNA (siCTGF and siHSP72), DOX, and AuNRs were jointly mounted on the octahedral DONs. (B) This composite structure maintains stability in the bloodstream and exerts chemotherapy, photothermal therapy, and gene therapy upon internalization by cancer cells.
To summarize, the multifunctional drug delivery system that is founded on DONs can trigger synergistic effects of physical, chemical, genetic, immune, and other treatments in a targeted manner toward cancer cells. This approach leads to enhanced efficacy in killing drug-resistant cancer cells. As a result, the DNA origami-based multifunctional drug delivery system presents a promising novel strategy for treating cancers that are resistant to multiple drugs (Table 4).
4 Challenges and prospects
DNA origami has made significant strides in drug delivery applications. However, there are still some obstacles to overcome. Firstly, the stability of DONs within the human body is not yet optimal and can be affected by several factors such as enzymes, pH levels, electrolytes, and temperature (Bila et al., 2019). The ability to stably load drugs into the body is critical for a drug delivery platform, as it significantly affects the safe entry of loaded drugs into targeted cells. Due to the fact that physiological fluids and blood serve as the primary media for the in vivo transportation of DONs, it is necessary to further enhance the stability of DNA origami structures within the circulatory system. This will enable them to effectively deliver anti-tumor drugs and fulfill their therapeutic potential. Existing research findings suggest that adding coatings to DNA nanostructures, such as lipids (Perrault and Shih, 2014), nucleotides (Kim and Yin, 2020), peptides (Auvinen et al., 2017), proteins (Wang et al., 2020), and other materials, can effectively enhance the in vivo stability of DONs. In the future, with the discovery and utilization of more valuable coatings, the stability of DONs is expected to be further strengthened. Xin et al. discovered that the use of cryoprotectants such as glycerol and trehalose effectively prevents the damage to DONs during multiple freeze-thaw cycles, ensuring their long-term preservation for drug in vitro applications (Xin et al., 2020). In contrast, Roodhuize et al. investigated the binding mechanisms between various ions and DONs due to the instability and degradation caused by low concentrations of cations in physiological fluids (Roodhuizen et al., 2019). This study lays the foundation for improving the stability of DONs in the liquid environment inside the body.
Modifying the surface of DONs can enable targeted delivery of anti-tumor drugs. However, off-target effects are still observed, which reduce the specific distribution of anti-tumor drugs in tissues, lower delivery efficiency, and pose a risk of severe adverse reactions. Therefore, improving the specific distribution capability of DONs in tissues and reducing off-target effects remains a crucial area of research in the field of DNA origami. One effective strategy to enhance target-specific binding affinity is to spatially arrange ligands on DONs, maximizing their binding with target proteins (Kwon et al., 2020). Additionally, increasing the number of ligands on DONs’ surfaces can also strengthen their targeting ability. For example, Lacroix et al. decorated DNA nanotubes with varying quantities of amphiphilic dendritic DNA (DDNA) to enhance the binding activity of DNA nanostructures with receptors, which were subsequently used for siRNA delivery (Seeman and Sleiman, 2017). In order to achieve more specific drug delivery, DONs need to exhibit more intelligent responsiveness to the external environment. In intelligent drug delivery system, Scherf et al. developed a DNA origami nanocage that responds to external stimuli (Scherf et al., 2022). The nanocage has small molecules on its surface that can be triggered to open and release drug payloads at specific sites upon receiving particular external cues. Additionally, even though DONs display high biocompatibility and low toxicity, they may still trigger an immune response if rejected by the body’s immune system (Surana et al., 2015). As drug delivery systems continue to evolve, precision and intelligence have become increasingly important.
Equally important is achieving efficient cellular uptake and release of DONs. Due to the negative charge and hydrophilic nature of DONs, passive transport into cells becomes challenging. Endocytosis is the primary mechanism by which DONs are internalized by cells, with clathrin-mediated endocytosis, caveolin-independent endocytosis, and macropinocytosis being significant pathways (Lim et al., 2015; Vindigni et al., 2016). Constructing more suitable shapes of DONs can facilitate cellular endocytosis. For instance, DNA nanostructures with a high aspect ratio are more readily absorbed by cells (Ouyang et al., 2013). Larger-sized DNA nanostructures exhibit higher cellular uptake efficiency compared to smaller ones. Additionally, rod-shaped DNA nanostructures perform better than similarly sized tetrahedral DNA nanostructures (Wang et al., 2018). Modifying DONs with folate, ligands, proteins, and other molecules can also enhance cellular internalization. Research has shown that viral capsid proteins can be electrostatically interacted with DONs, leading to their binding and self-assembly. Compared to bare DONs, the ability of virus protein-coated DONs to be internalized into cells was found to be increased by 13-fold (Mikkila et al., 2014). Furthermore, only those drugs that interact with DNA, such as CpG and siRNA, can be encapsulated or conjugated to these DONs. For other drugs, they may need to be combined with DNA, which could introduce additional barriers to their release within cells. For example, in the case of DOX, both pH values and DNA origami shapes can affect its release efficiency (Zhao et al., 2012; Zhang et al., 2014). Designing DONs that are compatible with these drugs is essential for their efficient release. For instance, pH-sensitive DONs that are highly sensitive to the intracellular pH environment. Researchers have designed an octahedral DNA origami that senses pH value changes and can switch between simple cubic (SC) and simple tetragonal (ST) structures (Wang et al., 2022c). This scientific advancement holds promise for delivering drugs based on pH value changes. In the future, extensive research is needed to explore and discover suitable DNA origami shapes for efficient delivery of various types of drugs. This will enable enhanced drug release within cells, mitigating the adverse effects associated with these limitations.
In addtion, DNA origami holds great potential for application in tumor diagnosis, with capabilities to detect tumor markers and perform molecular imaging of tumors. Tumor cells express specific markers on their membranes, which can be identified using DNA origami. By utilizing the controllability and programmability of DNA, DNA origami allows for the design of DNA molecules that can specifically recognize these markers, resulting in highly sensitive detection of tumor markers or tumor mutation genes (Liu et al., 2015; Chen et al., 2022; Domljanovic et al., 2022; Palankar, 2022; Chen et al., 2023). Moreover, on DONs, tracer molecules such as fluorescent dyes (commonly cyanine dyes like Cy5.5), quantum dots (QDs), radioactive isotopes, and other imaging agents can be precisely arranged at the nanoscale (Zeng et al., 2021). Subsequently, functionalization of DONs with ligands and other molecules can enable their targeting to tumor-associated cells, facilitating superior imaging of tumor tissues (Meng et al., 2016). This technology boasts higher spatial resolution and better biocompatibility compared to traditional imaging techniques (Bhatia et al., 2011; Zeng et al., 2021; Zeng et al., 2022). Overall, DNA origami offers numerous benefits in tumor diagnosis, including improved early diagnosis rates and high-sensitivity, high-resolution imaging of tumor tissues. These advantages bring about new possibilities for tumor research and treatment.
In summary, DNA origami is advancing towards better stability, safety, and intelligence. It has the potential to revolutionize drug delivery in the future.
5 Summary
DNA origami is a novel self-assembly technique with unique programmability, addressability, and biocompatibility. This technology offers several advantages in drug delivery. First, it allows for precise control of drug delivery by designing DONs. This improves therapeutic efficacy while reducing side effects. Second, DONs have demonstrated the ability to effectively cross biological barriers, such as cell membranes and the blood-brain barrier, resulting in efficient drug delivery. Third, DNA origami can be customized according to the characteristics of different drugs and diseases, enabling personalized treatment. Fourth, DNA is a natural material in the body and has excellent biocompatibility, with no significant immune response or toxicity in humans.
However, there are some shortcomings of DNA origami that need further research. Firstly, preparing DONs through DNA origami is a complicated process requiring strict control of experimental conditions, which can lead to structural instability and uneven assembly. Secondly, large-scale production of DNA origami is still in its early stages, with high production costs, low efficiency, and batch-to-batch variability, which limits its promotion and application in practical use (Praetorius et al., 2017). Thirdly, DONs are susceptible to external factors such as pH, temperature, and ion concentration, which may cause structural instability and deactivation, affecting their drug delivery effect. Finally, the complex nucleic acid sequences in DONs may pose risks such as instability and tumor induction, requiring further research on their biosafety.
Despite these challenges, DNA origami has made significant progress in chemotherapy drugs, nucleic acid drugs, peptide and protein drugs, immunotherapy, and other areas, demonstrating great potential for application. Moreover, multifunctional drug delivery systems can be created by loading multiple drugs into DONs, which can exert a combined therapeutic effect and improve tumor resistance. Although further research is required to address the stability and safety concerns associated with DNA origami, existing research results indicate that it is expected to play an extensive role in drug delivery.
Author contributions
Conceptualization, SJ and QL; writing original draft preparation, YZ; writing review and editing, SJ, QL, XT, ZW, HW, and FL; visualization: YZ; supervision: SJ and QL; funding acquisition: SJ and QL. All authors contributed to the article and approved the submitted version.
Funding
This study was supported by National Natural Science Foundation of China (grant no. 82074360), Shandong Provincial Natural Science Foundation (grant nos ZR2022MH319 and ZR2022LZY027), Young Taishan Scholars Program of Shandong Province (grant no. tsqn201909200), and Doctoral Fund of Jining No. 1 People’s Hospital (grant no. 2022-BS-007).
Conflict of interest
The authors declare that the research was conducted in the absence of any commercial or financial relationships that could be construed as a potential conflict of interest.
Publisher’s note
All claims expressed in this article are solely those of the authors and do not necessarily represent those of their affiliated organizations, or those of the publisher, the editors and the reviewers. Any product that may be evaluated in this article, or claim that may be made by its manufacturer, is not guaranteed or endorsed by the publisher.
References
Agarwal, N. P., Matthies, M., Gur, F. N., Osada, K., and Schmidt, T. L. (2017). Block copolymer micellization as a protection strategy for DNA origami. Angew. Chem. Int. Ed. Engl. 56, 5460–5464. doi:10.1002/anie.201608873
Alle, M., Sharma, G., Lee, S. H., and Kim, J. C. (2022). Next-generation engineered nanogold for multimodal cancer therapy and imaging: A clinical perspectives. J. Nanobiotechnology 20, 222. doi:10.1186/s12951-022-01402-z
Alvarado-Soto, L., and Ramirez-Tagle, R. (2015). A theoretical study of the binding of [Re₆Se₈(OH)₂(H₂O)₄] Rhenium clusters to DNA purine base guanine. Mater. (Basel) 8, 3938–3944. doi:10.3390/ma8073938
Amly, W., and Karaman, R. (2016). Recent updates in utilizing prodrugs in drug delivery (2013-2015). Expert Opin. Drug Deliv. 13, 571–591. doi:10.1517/17425247.2016.1142527
Auvinen, H., Zhang, H., Nonappakopilow, A., Niemela, E. H., Nummelin, S., Correia, A., et al. (2017). Protein coating of DNA nanostructures for enhanced stability and immunocompatibility. Adv. Healthc. Mater 6, 1700692. doi:10.1002/adhm.201700692
Balakrishnan, D., Wilkens, G. D., and Heddle, J. G. (2019). Delivering DNA origami to cells. Nanomedicine (Lond) 14, 911–925. doi:10.2217/nnm-2018-0440
Berger, R. M. L., Weck, J. M., Kempe, S. M., Hill, O., Liedl, T., Radler, J. O., et al. (2021). Nanoscale FasL organization on DNA origami to decipher apoptosis signal activation in cells. Small 17, e2101678. doi:10.1002/smll.202101678
Bhatia, D., Surana, S., Chakraborty, S., Koushika, S. P., and Krishnan, Y. (2011). A synthetic icosahedral DNA-based host-cargo complex for functional in vivo imaging. Nat. Commun. 2, 339. doi:10.1038/ncomms1337
Bila, H., Kurisinkal, E. E., and Bastings, M. M. C. (2019). Engineering a stable future for DNA-origami as a biomaterial. Biomater. Sci. 7, 532–541. doi:10.1039/c8bm01249k
Boisselier, E., and Astruc, D. (2009). Gold nanoparticles in nanomedicine: Preparations, imaging, diagnostics, therapies and toxicity. Chem. Soc. Rev. 38, 1759–1782. doi:10.1039/b806051g
Brabec, V., and Novakova, O. (2006). DNA binding mode of ruthenium complexes and relationship to tumor cell toxicity. Drug Resist Updat 9, 111–122. doi:10.1016/j.drup.2006.05.002
Brown, J. W. P., Alford, R. G., Walsh, J. C., Spinney, R. E., Xu, S. Y., Hertel, S., et al. (2022). Rapid exchange of stably bound protein and DNA cargo on a DNA origami receptor. ACS Nano 16, 6455–6467. doi:10.1021/acsnano.2c00699
Chang, M., Yang, C. S., and Huang, D. M. (2011). Aptamer-conjugated DNA icosahedral nanoparticles as a carrier of doxorubicin for cancer therapy. ACS Nano 5, 6156–6163. doi:10.1021/nn200693a
Chen, G., Liu, D., He, C., Gannett, T. R., Lin, W., and Weizmann, Y. (2015). Enzymatic synthesis of periodic DNA nanoribbons for intracellular pH sensing and gene silencing. J. Am. Chem. Soc. 137, 3844–3851. doi:10.1021/ja512665z
Chen, N., Qin, S., Yang, X., Wang, Q., Huang, J., and Wang, K. (2016). Sense-and-Treat" DNA nanodevice for synergetic destruction of circulating tumor cells. ACS Appl. Mater Interfaces 8, 26552–26558. doi:10.1021/acsami.6b08695
Chen, Q., Wang, X., Chen, J., Xiang, Y., Xiao, M., Pei, H., et al. (2022). Multiple-aptamer-Integrated DNA-origami-based chemical nose sensors for accurate identification of cancer cells. Anal. Chem. 94, 10192–10197. doi:10.1021/acs.analchem.2c01646
Chen, W., Jiang, M., Yu, W., Xu, Z., Liu, X., Jia, Q., et al. (2021). CpG-based nanovaccines for cancer immunotherapy. Int. J. Nanomedicine 16, 5281–5299. doi:10.2147/IJN.S317626
Chen, W., Li, T., Chen, C., Zhang, J., Ma, Z., Hou, W., et al. (2023). Three-dimensional ordered DNA network constructed by a biomarker pair for accurate monitoring of colorectal cancer. Biosens. Bioelectron. 232, 115335. doi:10.1016/j.bios.2023.115335
Cheung, A., Opzoomer, J., Ilieva, K. M., Gazinska, P., Hoffmann, R. M., Mirza, H., et al. (2018). Anti-folate receptor alpha-directed antibody therapies restrict the growth of triple-negative breast cancer. Clin. Cancer Res. 24, 5098–5111. doi:10.1158/1078-0432.CCR-18-0652
Chiu, Y. T. E., Li, H., and Choi, C. H. J. (2019). Progress toward understanding the interactions between DNA nanostructures and the cell. Small 15, e1805416. doi:10.1002/smll.201805416
Cho, Y., Lee, J. B., and Hong, J. (2014). Controlled release of an anti-cancer drug from DNA structured nano-films. Sci. Rep. 4, 4078. doi:10.1038/srep04078
Comberlato, A., Koga, M. M., Nussing, S., Parish, I. A., and Bastings, M. M. C. (2022). Spatially controlled activation of toll-like receptor 9 with DNA-based nanomaterials. Nano Lett. 22, 2506–2513. doi:10.1021/acs.nanolett.2c00275
De Llano, E., Miao, H., Ahmadi, Y., Wilson, A. J., Beeby, M., Viola, I., et al. (2020). Adenita: Interactive 3D modelling and visualization of DNA nanostructures. Nucleic Acids Res. 48, 8269–8275. doi:10.1093/nar/gkaa593
Deubel, D. V., and Chifotides, H. T. (2007). Guanine binding to dirhodium tetracarboxylate anticancer complexes: Quantum chemical calculations unravel an elusive mechanism. Chem. Commun. (Camb) 33, 3438–3440. doi:10.1039/b709209a
Dey, S., Fan, C., Gothelf, K. V., Li, J., Lin, C., Liu, L., et al. (2021). DNA origami. Nat. Rev. Methods Prim. 1, 13. doi:10.1038/s43586-020-00009-8
Domljanovic, I., Loretan, M., Kempter, S., Acuna, G. P., Kocabey, S., and Ruegg, C. (2022). DNA origami book biosensor for multiplex detection of cancer-associated nucleic acids. Nanoscale 14, 15432–15441. doi:10.1039/d2nr03985k
Douglas, S. M., Marblestone, A. H., Teerapittayanon, S., Vazquez, A., Church, G. M., and Shih, W. M. (2009). Rapid prototyping of 3D DNA-origami shapes with caDNAno. Nucleic Acids Res. 37, 5001–5006. doi:10.1093/nar/gkp436
Du, R. R., Cedrone, E., Romanov, A., Falkovich, R., Dobrovolskaia, M. A., and Bathe, M. (2022). Innate immune stimulation using 3D wireframe DNA origami. ACS Nano 16, 20340–20352. doi:10.1021/acsnano.2c06275
Fang, T., Alvelid, J., Spratt, J., Ambrosetti, E., Testa, I., and Teixeira, A. I. (2021). Spatial regulation of T-cell signaling by programmed death-ligand 1 on wireframe DNA origami flat sheets. ACS Nano 15, 3441–3452. doi:10.1021/acsnano.0c10632
Galindo-Murillo, R., Ruiz-Azuara, L., Moreno-Esparza, R., and Cortes-Guzman, F. (2012). Molecular recognition between DNA and a copper-based anticancer complex. Phys. Chem. Chem. Phys. 14, 15539–15546. doi:10.1039/c2cp42185b
Ge, Z., Guo, L., Wu, G., Li, J., Sun, Y., Hou, Y., et al. (2020). DNA origami-enabled engineering of ligand-drug conjugates for targeted drug delivery. Small 16, e1904857. doi:10.1002/smll.201904857
Gu, D., Qiao, Y., Fu, H., Zhao, H., Yue, X., Wang, S., et al. (2022). Size-controllable DNA origami-stacked gold nanoparticles for deep tumor-penetrating therapy. ACS Appl. Mater Interfaces 14, 38048–38055. doi:10.1021/acsami.2c05750
Haleel, A. K., Rafi, U. M., Mahendiran, D., Mitu, L., Veena, V., and Rahiman, A. K. (2019). DNA profiling and in vitro cytotoxicity studies of tetrazolo[1,5-a]pyrimidine-based copper(II) complexes. Biometals 32, 611–626. doi:10.1007/s10534-019-00196-2
Halley, P. D., Lucas, C. R., Mcwilliams, E. M., Webber, M. J., Patton, R. A., Kural, C., et al. (2016). Daunorubicin-loaded DNA origami nanostructures circumvent drug-resistance mechanisms in a leukemia model. Small 12, 308–320. doi:10.1002/smll.201502118
Han, X., Jiang, Y., Li, S., Zhang, Y., Ma, X., Wu, Z., et al. (2018). Multivalent aptamer-modified tetrahedral DNA nanocage demonstrates high selectivity and safety for anti-tumor therapy. Nanoscale 11, 339–347. doi:10.1039/c8nr05546g
Hannewald, N., Winterwerber, P., Zechel, S., Ng, D. Y. W., Hager, M. D., Weil, T., et al. (2021). DNA origami meets polymers: A powerful tool for the design of defined nanostructures. Angew. Chem. Int. Ed. Engl. 60, 6218–6229. doi:10.1002/anie.202005907
He, Z., Xiang, W., Fan, Q., Wang, L., and Chao, J. (2023). A DNA origami nanostructure embedded with NQO1-activated prodrugs for precision drug delivery. Chem. Commun. (Camb) 59, 912–915. doi:10.1039/d2cc06367k
Hu, J., Cao, J., Topatana, W., Juengpanich, S., Li, S., Zhang, B., et al. (2021). Targeting mutant p53 for cancer therapy: Direct and indirect strategies. J. Hematol. Oncol. 14, 157. doi:10.1186/s13045-021-01169-0
Hu, Q., Li, H., Wang, L., Gu, H., and Fan, C. (2019). DNA nanotechnology-enabled drug delivery systems. Chem. Rev. 119, 6459–6506. doi:10.1021/acs.chemrev.7b00663
Huang, D., Patel, K., Perez-Garrido, S., Marshall, J. F., and Palma, M. (2019). DNA origami nanoarrays for multivalent investigations of cancer cell spreading with nanoscale spatial resolution and single-molecule control. ACS Nano 13, 728–736. doi:10.1021/acsnano.8b08010
Huang, Y., Huang, W., Chan, L., Zhou, B., and Chen, T. (2016). A multifunctional DNA origami as carrier of metal complexes to achieve enhanced tumoral delivery and nullified systemic toxicity. Biomaterials 103, 183–196. doi:10.1016/j.biomaterials.2016.06.053
Hyungmin, J., Xiao, W., William, P. B., Steve, J., and Mark, B. (2020). Rapid prototyping of wireframe scaffolded DNA origami using ATHENA. bioRxiv.
Ijas, H., Shen, B., Heuer-Jungemann, A., Keller, A., Kostiainen, M. A., Liedl, T., et al. (2021). Unraveling the interaction between doxorubicin and DNA origami nanostructures for customizable chemotherapeutic drug release. Nucleic Acids Res. 49, 3048–3062. doi:10.1093/nar/gkab097
Jiang, D., Ge, Z., Im, H. J., England, C. G., Ni, D., Hou, J., et al. (2018). DNA origami nanostructures can exhibit preferential renal uptake and alleviate acute kidney injury. Nat. Biomed. Eng. 2, 865–877. doi:10.1038/s41551-018-0317-8
Jiang, Q., Liu, S., Liu, J., Wang, Z. G., and Ding, B. (2019). Rationally designed DNA-origami nanomaterials for drug delivery in vivo. Adv. Mater 31, e1804785. doi:10.1002/adma.201804785
Jiang, Q., Song, C., Nangreave, J., Liu, X., Lin, L., Qiu, D., et al. (2012). DNA origami as a carrier for circumvention of drug resistance. J. Am. Chem. Soc. 134, 13396–13403. doi:10.1021/ja304263n
Jin, Z., Nguyen, K. T., Go, G., Kang, B., Min, H. K., Kim, S. J., et al. (2019). Multifunctional nanorobot system for active therapeutic delivery and synergistic chemo-photothermal therapy. Nano Lett. 19, 8550–8564. doi:10.1021/acs.nanolett.9b03051
Jorge, A. F., Avino, A., Pais, A., Eritja, R., and Fabrega, C. (2018). DNA-based nanoscaffolds as vehicles for 5-fluoro-2'-deoxyuridine oligomers in colorectal cancer therapy. Nanoscale 10, 7238–7249. doi:10.1039/c7nr08442k
Kang, Y., Zhang, W., Yu, Q., Gao, L., Quan, J., Gu, F., et al. (2023). Self-assembled nanoparticles based on DNA origami and a nitrated T helper cell epitope as a platform for the development of personalized cancer vaccines. Cancer Immunol. Immunother. 72, 2741–2755. doi:10.1007/s00262-023-03446-y
Kern, N., Dong, R., Douglas, S. M., Vale, R. D., and Morrissey, M. A. (2021). Tight nanoscale clustering of Fcγ receptors using DNA origami promotes phagocytosis. Elife 10, e68311. doi:10.7554/eLife.68311
Kim, J., Yu, L., Chen, W., Xu, Y., Wu, M., Todorova, D., et al. (2019). Wild-type p53 promotes cancer metabolic switch by inducing PUMA-dependent suppression of oxidative phosphorylation. Cancer Cell. 35, 191–203. doi:10.1016/j.ccell.2018.12.012
Kim, K. R., Kim, J., Back, J. H., Lee, J. E., and Ahn, D. R. (2022). Cholesterol-mediated seeding of protein corona on DNA nanostructures for targeted delivery of oligonucleotide therapeutics to treat liver fibrosis. ACS Nano 16, 7331–7343. doi:10.1021/acsnano.1c08508
Kim, Y., and Yin, P. (2020). Enhancing biocompatible stability of DNA nanostructures using dendritic oligonucleotides and brick motifs. Angew. Chem. Int. Ed. Engl. 59, 700–703. doi:10.1002/anie.201911664
Kong, F., Zhang, H., Qu, X., Zhang, X., Chen, D., Ding, R., et al. (2016). Gold nanorods, DNA origami, and porous silicon nanoparticle-functionalized biocompatible double emulsion for versatile targeted therapeutics and antibody combination therapy. Adv. Mater 28, 10195–10203. doi:10.1002/adma.201602763
Krieg, A. M. (2002). CpG motifs in bacterial DNA and their immune effects. Annu. Rev. Immunol. 20, 709–760. doi:10.1146/annurev.immunol.20.100301.064842
Kumar, V., Palazzolo, S., Bayda, S., Corona, G., Toffoli, G., and Rizzolio, F. (2016). DNA nanotechnology for cancer therapy. Theranostics 6, 710–725. doi:10.7150/thno.14203
Kwon, P. S., Ren, S., Kwon, S. J., Kizer, M. E., Kuo, L., Xie, M., et al. (2020). Designer DNA architecture offers precise and multivalent spatial pattern-recognition for viral sensing and inhibition. Nat. Chem. 12, 26–35. doi:10.1038/s41557-019-0369-8
Lacroix, A., Edwardson, T. G. W., Hancock, M. A., Dore, M. D., and Sleiman, H. F. (2017). Development of DNA nanostructures for high-affinity binding to human serum albumin. J. Am. Chem. Soc. 139, 7355–7362. doi:10.1021/jacs.7b02917
Lee, H., Lytton-Jean, A. K., Chen, Y., Love, K. T., Park, A. I., Karagiannis, E. D., et al. (2012). Molecularly self-assembled nucleic acid nanoparticles for targeted in vivo siRNA delivery. Nat. Nanotechnol. 7, 389–393. doi:10.1038/nnano.2012.73
Li, B., Shao, H., Gao, L., Li, H., Sheng, H., and Zhu, L. (2022). Nano-drug co-delivery system of natural active ingredients and chemotherapy drugs for cancer treatment: A review. Drug Deliv. 29, 2130–2161. doi:10.1080/10717544.2022.2094498
Li, H., Liu, J., and Gu, H. (2019a). Targeting nucleolin to obstruct vasculature feeding with an intelligent DNA nanorobot. J. Cell. Mol. Med. 23, 2248–2250. doi:10.1111/jcmm.14127
Li, J., Fan, C., Pei, H., Shi, J., and Huang, Q. (2013). Smart drug delivery nanocarriers with self-assembled DNA nanostructures. Adv. Mater 25, 4386–4396. doi:10.1002/adma.201300875
Li, J., Pei, H., Zhu, B., Liang, L., Wei, M., He, Y., et al. (2011). Self-assembled multivalent DNA nanostructures for noninvasive intracellular delivery of immunostimulatory CpG oligonucleotides. ACS Nano 5, 8783–8789. doi:10.1021/nn202774x
Li, S., Jiang, Q., Ding, B., and Nie, G. (2019b). Anticancer activities of tumor-killing nanorobots. Trends Biotechnol. 37, 573–577. doi:10.1016/j.tibtech.2019.01.010
Li, S., Jiang, Q., Liu, S., Zhang, Y., Tian, Y., Song, C., et al. (2018). A DNA nanorobot functions as a cancer therapeutic in response to a molecular trigger in vivo. Nat. Biotechnol. 36, 258–264. doi:10.1038/nbt.4071
Li, W., Wang, C., Lv, H., Wang, Z., Zhao, M., Liu, S., et al. (2021). A DNA nanoraft-based cytokine delivery platform for alleviation of acute kidney injury. ACS Nano 15, 18237–18249. doi:10.1021/acsnano.1c07270
Li, X., Wang, X., Li, H., Shi, X., and Zheng, P. (2020). A programming 20-30nm rectangular DNA origami for loading doxorubicin to penetrate ovarian cancer cells. IEEE Trans. Nanobioscience 19, 152–157. doi:10.1109/TNB.2019.2943923
Lim, K. S., Lee, D. Y., Valencia, G. M., Won, Y. W., and Bull, D. A. (2015). Nano-self-assembly of nucleic acids capable of transfection without a gene carrier. Adv. Funct. Mater. 25, 5445–5451. doi:10.1002/adfm.201502067
Liu, J., Song, L., Liu, S., Zhao, S., Jiang, Q., and Ding, B. (2018a). A tailored DNA nanoplatform for synergistic RNAi-/chemotherapy of multidrug-resistant tumors. Angew. Chem. Int. Ed. Engl. 57, 15486–15490. doi:10.1002/anie.201809452
Liu, S., Jiang, Q., Zhao, X., Zhao, R., Wang, Y., Wang, Y., et al. (2021). A DNA nanodevice-based vaccine for cancer immunotherapy. Nat. Mater 20, 421–430. doi:10.1038/s41563-020-0793-6
Liu, S., Su, W., Li, Z., and Ding, X. (2015). Electrochemical detection of lung cancer specific microRNAs using 3D DNA origami nanostructures. Biosens. Bioelectron. 71, 57–61. doi:10.1016/j.bios.2015.04.006
Liu, X., Xu, Y., Yu, T., Clifford, C., Liu, Y., Yan, H., et al. (2012). A DNA nanostructure platform for directed assembly of synthetic vaccines. Nano Lett. 12, 4254–4259. doi:10.1021/nl301877k
Liu, X., Zhang, F., Jing, X., Pan, M., Liu, P., Li, W., et al. (2018b). Complex silica composite nanomaterials templated with DNA origami. Nature 559, 593–598. doi:10.1038/s41586-018-0332-7
Long, Q., Jia, B., Shi, Y., Wang, Q., Yu, H., and Li, Z. (2021). DNA nanodevice as a Co-delivery vehicle of antisense oligonucleotide and silver ions for selective inhibition of bacteria growth. ACS Appl. Mater Interfaces 13, 47987–47995. doi:10.1021/acsami.1c15585
Lu, X., Liu, J., Wu, X., and Ding, B. (2019). Multifunctional DNA origami nanoplatforms for drug delivery. Chem. Asian J. 14, 2193–2202. doi:10.1002/asia.201900574
Ma, N., Cheng, K., Feng, Q., Liu, G., Liang, J., Ma, X., et al. (2023). Nanoscale organization of TRAIL trimers using DNA origami to promote clustering of death receptor and cancer cell apoptosis. Small 19, e2206160. doi:10.1002/smll.202206160
Ma, W. K., Voss, D. M., Scharner, J., Costa, A. S. H., Lin, K. T., Jeon, H. Y., et al. (2022a). ASO-based PKM splice-switching therapy inhibits hepatocellular carcinoma growth. Cancer Res. 82, 900–915. doi:10.1158/0008-5472.CAN-20-0948
Ma, Y., Lu, Z., Jia, B., Shi, Y., Dong, J., Jiang, S., et al. (2022b). DNA origami as a nanomedicine for targeted rheumatoid arthritis therapy through reactive oxygen species and nitric oxide scavenging. ACS Nano 16, 12520–12531. doi:10.1021/acsnano.2c03991
Ma, Y., Wang, Z., Ma, Y., Han, Z., Zhang, M., Chen, H., et al. (2018). A telomerase-responsive DNA icosahedron for precise delivery of platinum nanodrugs to cisplatin-resistant cancer. Angew. Chem. Int. Ed. Engl. 57, 5389–5393. doi:10.1002/anie.201801195
Madhanagopal, B. R., Zhang, S., Demirel, E., Wady, H., and Chandrasekaran, A. R. (2018). DNA nanocarriers: Programmed to deliver. Trends Biochem. Sci. 43, 997–1013. doi:10.1016/j.tibs.2018.09.010
Marras, A. E., Zhou, L., Su, H. J., and Castro, C. E. (2015). Programmable motion of DNA origami mechanisms. Proc. Natl. Acad. Sci. U. S. A. 112, 713–718. doi:10.1073/pnas.1408869112
Meng, H. M., Liu, H., Kuai, H., Peng, R., Mo, L., and Zhang, X. B. (2016). Aptamer-integrated DNA nanostructures for biosensing, bioimaging and cancer therapy. Chem. Soc. Rev. 45, 2583–2602. doi:10.1039/c5cs00645g
Meng, J., Zhang, P., Chen, Q., Wang, Z., Gu, Y., Ma, J., et al. (2022). Two-pronged intracellular Co-delivery of antigen and adjuvant for synergistic cancer immunotherapy. Adv. Mater 34, e2202168. doi:10.1002/adma.202202168
Mikkila, J., Eskelinen, A. P., Niemela, E. H., Linko, V., Frilander, M. J., Torma, P., et al. (2014). Virus-encapsulated DNA origami nanostructures for cellular delivery. Nano Lett. 14, 2196–2200. doi:10.1021/nl500677j
Mohri, K., Nishikawa, M., Takahashi, N., Shiomi, T., Matsuoka, N., Ogawa, K., et al. (2012a). Design and development of nanosized DNA assemblies in polypod-like structures as efficient vehicles for immunostimulatory CpG motifs to immune cells. ACS Nano 6, 5931–5940. doi:10.1021/nn300727j
Mohri, K., Takahashi, N., Nishikawa, M., Kusuki, E., Shiomi, T., Takahashi, Y., et al. (2012b). Increased immunostimulatory activity of polypod-like structured DNA by ligation of the terminal loop structures. J. Control Release 163, 285–292. doi:10.1016/j.jconrel.2012.08.001
Necela, B. M., Crozier, J. A., Andorfer, C. A., Lewis-Tuffin, L., Kachergus, J. M., Geiger, X. J., et al. (2015). Folate receptor-α (FOLR1) expression and function in triple negative tumors. PLoS One 10, e0122209. doi:10.1371/journal.pone.0122209
Nguyen, L., Doblinger, M., Liedl, T., and Heuer-Jungemann, A. (2019). DNA-Origami-Templated silica growth by sol-gel chemistry. Angew. Chem. Int. Ed. Engl. 58, 912–916. doi:10.1002/anie.201811323
Noriega-Rivera, R., Rivera-Serrano, M., Rabelo-Fernandez, R. J., Perez-Santiago, J., Valiyeva, F., and Vivas-Mejia, P. E. (2022). Increased expression of the RBPMS splice variants inhibits cell proliferation in ovarian cancer cells. Int. J. Mol. Sci. 23, 14742. doi:10.3390/ijms232314742
Ouyang, X., De Stefano, M., Krissanaprasit, A., Bank Kodal, A. L., Bech Rosen, C., Liu, T., et al. (2017). Docking of antibodies into the cavities of DNA origami structures. Angew. Chem. Int. Ed. Engl. 56, 14423–14427. doi:10.1002/anie.201706765
Ouyang, X., Li, J., Liu, H., Zhao, B., Yan, J., He, D., et al. (2014). Self-assembly of DNA-based drug delivery nanocarriers with rolling circle amplification. Methods 67, 198–204. doi:10.1016/j.ymeth.2013.05.024
Ouyang, X., Li, J., Liu, H., Zhao, B., Yan, J., Ma, Y., et al. (2013). Rolling circle amplification-based DNA origami nanostructrures for intracellular delivery of immunostimulatory drugs. Small 9, 3082–3087. doi:10.1002/smll.201300458
Pal, S., and Rakshit, T. (2021). Folate-functionalized DNA origami for targeted delivery of doxorubicin to triple-negative breast cancer. Front. Chem. 9, 721105. doi:10.3389/fchem.2021.721105
Palankar, R. (2022). Light responsive DNA origami detects breast cancer marker. Nat. Nanotechnol. 17, 1048–1049. doi:10.1038/s41565-022-01240-y
Palazzolo, S., Hadla, M., Russo Spena, C., Caligiuri, I., Rotondo, R., Adeel, M., et al. (2019). An effective multi-stage liposomal DNA origami nanosystem for in vivo cancer therapy. Cancers (Basel) 11, 1997. doi:10.3390/cancers11121997
Pan, Q., Nie, C., Hu, Y., Yi, J., Liu, C., Zhang, J., et al. (2020). Aptamer-functionalized DNA origami for targeted codelivery of antisense oligonucleotides and doxorubicin to enhance therapy in drug-resistant cancer cells. ACS Appl. Mater Interfaces 12, 400–409. doi:10.1021/acsami.9b20707
Perez-Arnaiz, C., Busto, N., Leal, J. M., and Garcia, B. (2014). New insights into the mechanism of the DNA/doxorubicin interaction. J. Phys. Chem. B 118, 1288–1295. doi:10.1021/jp411429g
Perrault, S. D., and Shih, W. M. (2014). Virus-inspired membrane encapsulation of DNA nanostructures to achieve in vivo stability. ACS Nano 8, 5132–5140. doi:10.1021/nn5011914
Ponnuswamy, N., Bastings, M. M. C., Nathwani, B., Ryu, J. H., Chou, L. Y. T., Vinther, M., et al. (2017). Oligolysine-based coating protects DNA nanostructures from low-salt denaturation and nuclease degradation. Nat. Commun. 8, 15654. doi:10.1038/ncomms15654
Praetorius, F., Kick, B., Behler, K. L., Honemann, M. N., Weuster-Botz, D., and Dietz, H. (2017). Biotechnological mass production of DNA origami. Nature 552, 84–87. doi:10.1038/nature24650
Qu, Y., Yang, J., Zhan, P., Liu, S., Zhang, K., Jiang, Q., et al. (2017). Self-assembled DNA dendrimer nanoparticle for efficient delivery of immunostimulatory CpG motifs. ACS Appl. Mater Interfaces 9, 20324–20329. doi:10.1021/acsami.7b05890
Rahman, M. A., Wang, P., Zhao, Z., Wang, D., Nannapaneni, S., Zhang, C., et al. (2017). Systemic delivery of bc12-targeting siRNA by DNA nanoparticles suppresses cancer cell growth. Angew. Chem. Int. Ed. Engl. 56, 16023–16027. doi:10.1002/anie.201709485
Rajendran, A., Krishnamurthy, K., Giridasappa, A., Nakata, E., and Morii, T. (2021). Stabilization and structural changes of 2D DNA origami by enzymatic ligation. Nucleic Acids Res. 49, 7884–7900. doi:10.1093/nar/gkab611
Rattanakiat, S., Nishikawa, M., Funabashi, H., Luo, D., and Takakura, Y. (2009). The assembly of a short linear natural cytosine-phosphate-guanine DNA into dendritic structures and its effect on immunostimulatory activity. Biomaterials 30, 5701–5706. doi:10.1016/j.biomaterials.2009.06.053
Revenko, A., Carnevalli, L. S., Sinclair, C., Johnson, B., Peter, A., Taylor, M., et al. (2022). Direct targeting of FOXP3 in Tregs with AZD8701, a novel antisense oligonucleotide to relieve immunosuppression in cancer. J. Immunother. Cancer 10, e003892. doi:10.1136/jitc-2021-003892
Roberts, T. C., Langer, R., and Wood, M. J. A. (2020). Advances in oligonucleotide drug delivery. Nat. Rev. Drug Discov. 19, 673–694. doi:10.1038/s41573-020-0075-7
Roodhuizen, J. A. L., Hendrikx, P., Hilbers, P. A. J., De Greef, T. F. A., and Markvoort, A. J. (2019). Counterion-dependent mechanisms of DNA origami nanostructure stabilization revealed by atomistic molecular simulation. ACS Nano 13, 10798–10809. doi:10.1021/acsnano.9b05650
Rosier, B., Markvoort, A. J., Gumi Audenis, B., Roodhuizen, J. A. L., Den Hamer, A., Brunsveld, L., et al. (2020). Proximity-induced caspase-9 activation on a DNA origami-based synthetic apoptosome. Nat. Catal. 3, 295–306. doi:10.1038/s41929-019-0403-7
Rothemund, P. W. (2006). Folding DNA to create nanoscale shapes and patterns. Nature 440, 297–302. doi:10.1038/nature04586
Ryu, Y., Hong, C. A., Song, Y., Beak, J., Seo, B. A., Lee, J. J., et al. (2020). Modular protein-DNA hybrid nanostructures as a drug delivery platform. Nanoscale 12, 4975–4981. doi:10.1039/c9nr08519j
Ryu, Y., Kang, J. A., Kim, D., Kim, S. R., Kim, S., Park, S. J., et al. (2018). Programed assembly of nucleoprotein nanoparticles using DNA and zinc fingers for targeted protein delivery. Small 14, e1802618. doi:10.1002/smll.201802618
Sayin, K., and Ungordu, A. (2018). Investigation of anticancer properties of caffeinated complexes via computational chemistry methods. Spectrochim. Acta A Mol. Biomol. Spectrosc. 193, 147–155. doi:10.1016/j.saa.2017.12.013
Schaffert, D. H., Okholm, A. H., Sorensen, R. S., Nielsen, J. S., Torring, T., Rosen, C. B., et al. (2016). Intracellular delivery of a planar DNA origami structure by the transferrin-receptor internalization pathway. Small 12, 2634–2640. doi:10.1002/smll.201503934
Scherf, M., Scheffler, F., Maffeo, C., Kemper, U., Ye, J., Aksimentiev, A., et al. (2022). Trapping of protein cargo molecules inside DNA origami nanocages. Nanoscale 14, 18041–18050. doi:10.1039/d2nr05356j
Schuller, V. J., Heidegger, S., Sandholzer, N., Nickels, P. C., Suhartha, N. A., Endres, S., et al. (2011). Cellular immunostimulation by CpG-sequence-coated DNA origami structures. ACS Nano 5, 9696–9702. doi:10.1021/nn203161y
Seeman, N. C., and Sleiman, H. F. (2017). DNA nanotechnology. Nat. Rev. Mater. 3, 17068. doi:10.1038/natrevmats.2017.68
Seitz, I., Ijas, H., Linko, V., and Kostiainen, M. A. (2022). Optically responsive protein coating of DNA origami for triggered antigen targeting. ACS Appl. Mater Interfaces 14, 38515–38524. doi:10.1021/acsami.2c10058
Shen, M., Xie, S., Rowicki, M., Michel, S., Wei, Y., Hang, X., et al. (2021). Therapeutic targeting of metadherin suppresses colorectal and lung cancer progression and metastasis. Cancer Res. 81, 1014–1025. doi:10.1158/0008-5472.CAN-20-1876
Singh, R., and Deshmukh, R. (2023). DNA nanobots - emerging customized nanomedicine in oncology. Curr. Drug Deliv. 20, 111–126. doi:10.2174/1567201819666220331094812
Song, D. G., Ye, Q., Poussin, M., Chacon, J. A., Figini, M., and Powell, D. J. (2016). Effective adoptive immunotherapy of triple-negative breast cancer by folate receptor-alpha redirected CAR T cells is influenced by surface antigen expression level. J. Hematol. Oncol. 9, 56. doi:10.1186/s13045-016-0285-y
Song, L., Jiang, Q., Liu, J., Li, N., Liu, Q., Dai, L., et al. (2017). DNA origami/gold nanorod hybrid nanostructures for the circumvention of drug resistance. Nanoscale 9, 7750–7754. doi:10.1039/c7nr02222k
Sponer, J. E., Leszczynski, J., and Sponer, J. (2006). Mechanism of action of anticancer titanocene derivatives: An insight from quantum chemical calculations. J. Phys. Chem. B 110, 19632–19636. doi:10.1021/jp063477r
Subhan, M. A., and Torchilin, V. P. (2019). Efficient nanocarriers of siRNA therapeutics for cancer treatment. Transl. Res. 214, 62–91. doi:10.1016/j.trsl.2019.07.006
Sun, Y., Sun, J., Xiao, M., Lai, W., Li, L., Fan, C., et al. (2022). DNA origami-based artificial antigen-presenting cells for adoptive T cell therapy. Sci. Adv. 8, eadd1106. doi:10.1126/sciadv.add1106
Surana, S., Shenoy, A. R., and Krishnan, Y. (2015). Designing DNA nanodevices for compatibility with the immune system of higher organisms. Nat. Nanotechnol. 10, 741–747. doi:10.1038/nnano.2015.180
Taghdisi, S. M., Danesh, N. M., Ramezani, M., Yazdian-Robati, R., and Abnous, K. (2018). A novel AS1411 aptamer-based three-way junction pocket DNA nanostructure loaded with doxorubicin for targeting cancer cells in vitro and in vivo. Mol. Pharm. 15, 1972–1978. doi:10.1021/acs.molpharmaceut.8b00124
Thangavel, P., Viswanath, B., and Kim, S. (2017). Recent developments in the nanostructured materials functionalized with ruthenium complexes for targeted drug delivery to tumors. Int. J. Nanomedicine 12, 2749–2758. doi:10.2147/IJN.S131304
Udomprasert, A., and Kangsamaksin, T. (2017). DNA origami applications in cancer therapy. Cancer Sci. 108, 1535–1543. doi:10.1111/cas.13290
Unida, V., Vindigni, G., Raniolo, S., Stolfi, C., Desideri, A., and Biocca, S. (2022). Folate-functionalization enhances cytotoxicity of multivalent DNA nanocages on triple-negative breast cancer cells. Pharmaceutics 14, 2610. doi:10.3390/pharmaceutics14122610
Veneziano, R., Ratanalert, S., Zhang, K., Zhang, F., Yan, H., Chiu, W., et al. (2016). Designer nanoscale DNA assemblies programmed from the top down. Science 352, 1534. doi:10.1126/science.aaf4388
Verheyen, T., Fang, T., Lindenhofer, D., Wang, Y., Akopyan, K., Lindqvist, A., et al. (2020). Spatial organization-dependent EphA2 transcriptional responses revealed by ligand nanocalipers. Nucleic Acids Res. 48, 5777–5787. doi:10.1093/nar/gkaa274
Vindigni, G., Raniolo, S., Ottaviani, A., Falconi, M., Franch, O., Knudsen, B. R., et al. (2016). Receptor-mediated entry of pristine octahedral DNA nanocages in mammalian cells. ACS Nano 10, 5971–5979. doi:10.1021/acsnano.6b01402
Wang, M. (2019). DNA origami scavenges ROS in the kidney. Nat. Rev. Nephrol. 15, 61. doi:10.1038/s41581-018-0090-7
Wang, M., Yang, D., Lu, Q., Liu, L., Cai, Z., Wang, Y., et al. (2022a). Spatially reprogramed receptor organization to switch cell behavior using a DNA origami-templated aptamer nanoarray. Nano Lett. 22, 8445–8454. doi:10.1021/acs.nanolett.2c02489
Wang, P., Rahman, M. A., Zhao, Z., Weiss, K., Zhang, C., Chen, Z., et al. (2018). Visualization of the cellular uptake and trafficking of DNA origami nanostructures in cancer cells. J. Am. Chem. Soc. 140, 2478–2484. doi:10.1021/jacs.7b09024
Wang, S. T., Gray, M. A., Xuan, S., Lin, Y., Byrnes, J., Nguyen, A. I., et al. (2020). DNA origami protection and molecular interfacing through engineered sequence-defined peptoids. Proc. Natl. Acad. Sci. U. S. A. 117, 6339–6348. doi:10.1073/pnas.1919749117
Wang, X., Li, S., Jun, H., John, T., Zhang, K., Fowler, H., et al. (2022b). Planar 2D wireframe DNA origami. Sci. Adv. 8, eabn0039. doi:10.1126/sciadv.abn0039
Wang, Y., Baars, I., Fordos, F., and Hogberg, B. (2021a). Clustering of death receptor for apoptosis using nanoscale patterns of peptides. ACS Nano 15, 9614–9626. doi:10.1021/acsnano.0c10104
Wang, Y., Yan, X., Zhou, Z., Ma, N., and Tian, Y. (2022c). pH-induced symmetry conversion of DNA origami lattices. Angew. Chem. Int. Ed. Engl. 61, e202208290. doi:10.1002/anie.202208290
Wang, Z., Song, L., Liu, Q., Tian, R., Shang, Y., Liu, F., et al. (2021b). A tubular DNA nanodevice as a siRNA/chemo-drug Co-delivery vehicle for combined cancer therapy. Angew. Chem. Int. Ed. Engl. 60, 2594–2598. doi:10.1002/anie.202009842
Wang, Z., Wang, S., Qin, J., Zhang, X., Lu, G., Liu, H., et al. (2022d). Splicing factor BUD31 promotes ovarian cancer progression through sustaining the expression of anti-apoptotic BCL2L12. Nat. Commun. 13, 6246. doi:10.1038/s41467-022-34042-w
Wassermann, L. M., Scheckenbach, M., Baptist, A. V., Glembockyte, V., and Heuer-Jungemann, A. (2023). Full site-specific addressability in DNA origami-templated silica nanostructures. Adv. Mater 35, e2212024. doi:10.1002/adma.202212024
Wu, S., Zhang, M., Song, J., Weber, S., Liu, X., Fan, C., et al. (2021a). Fine customization of calcium phosphate nanostructures with site-specific modification by DNA templated mineralization. ACS Nano 15, 1555–1565. doi:10.1021/acsnano.0c08998
Wu, T., Liu, J., Liu, M., Liu, S., Zhao, S., Tian, R., et al. (2019). A nanobody-conjugated DNA nanoplatform for targeted platinum-drug delivery. Angew. Chem. Int. Ed. Engl. 58, 14224–14228. doi:10.1002/anie.201909345
Wu, X., Yang, C., Wang, H., Lu, X., Shang, Y., Liu, Q., et al. (2023). Genetically encoded DNA origami for gene therapy in vivo. J. Am. Chem. Soc. 145, 9343–9353. doi:10.1021/jacs.3c02756
Wu, Y., Li, Q., Shim, G., and Oh, Y. K. (2021b). Melanin-loaded CpG DNA hydrogel for modulation of tumor immune microenvironment. J. Control Release 330, 540–553. doi:10.1016/j.jconrel.2020.12.040
Xin, Y., Kielar, C., Zhu, S., Sikeler, C., Xu, X., Moser, C., et al. (2020). Cryopreservation of DNA origami nanostructures. Small 16, e1905959. doi:10.1002/smll.201905959
Xin, Y., Piskunen, P., Suma, A., Li, C., Ijas, H., Ojasalo, S., et al. (2022). Environment-dependent stability and mechanical properties of DNA origami six-helix bundles with different crossover spacings. Small 18, e2107393. doi:10.1002/smll.202107393
Xu, T., Yu, S., Sun, Y., Wu, S., Gao, D., Wang, M., et al. (2021a). DNA origami frameworks enabled self-protective siRNA delivery for dual enhancement of chemo-photothermal combination therapy. Small 17, e2101780. doi:10.1002/smll.202101780
Xu, X., Gan, M., Ge, Y., Yi, C., Feng, T., Liu, M., et al. (2021b). Multifaceted glycoadjuvant@AuNPs inhibits tumor metastasis through promoting T cell activation and remodeling tumor microenvironment. J. Nanobiotechnology 19, 376. doi:10.1186/s12951-021-01129-3
Xue, H., Ding, F., Zhang, J., Guo, Y., Gao, X., Feng, J., et al. (2019). DNA tetrahedron-based nanogels for siRNA delivery and gene silencing. Chem. Commun. (Camb) 55, 4222–4225. doi:10.1039/c9cc00175a
Yaghoobi, E., Zavvar, T., Ramezani, M., Alibolandi, M., Rahimzadeh Oskuei, S., Zahiri, M., et al. (2022). A multi-storey DNA nanostructure containing doxorubicin and AS1411 aptamer for targeting breast cancer cells. J. Drug Target 30, 1106–1112. doi:10.1080/1061186X.2022.2094387
Yan, J., Hu, C., Liu, X., Zhong, J., Sun, G., and He, D. (2015). Recent developments of new DNA origami nanostructures for drug delivery. Curr. Pharm. Des. 21, 3181–3190. doi:10.2174/1381612821666150531165551
Yao, F., An, Y., Li, X., Li, Z., Duan, J., and Yang, X. D. (2020). Targeted therapy of colon cancer by aptamer-guided holliday junctions loaded with doxorubicin. Int. J. Nanomedicine 15, 2119–2129. doi:10.2147/IJN.S240083
Zeng, Y., Chang, P., Ma, J., Li, K., Zhang, C., Guo, Y., et al. (2022). DNA origami-anthraquinone hybrid nanostructures for in vivo quantitative monitoring of the progression of tumor hypoxia affected by chemotherapy. ACS Appl. Mater Interfaces 14, 6387–6403. doi:10.1021/acsami.1c22620
Zeng, Y., Liu, J., Yang, S., Liu, W., Xu, L., and Wang, R. (2018). Time-lapse live cell imaging to monitor doxorubicin release from DNA origami nanostructures. J. Mater Chem. B 6, 1605–1612. doi:10.1039/C7TB03223D
Zeng, Y., Nixon, R. L., Liu, W., and Wang, R. (2021). The applications of functionalized DNA nanostructures in bioimaging and cancer therapy. Biomaterials 268, 120560. doi:10.1016/j.biomaterials.2020.120560
Zhang, H., Qu, X., Chen, H., Kong, H., Ding, R., Chen, D., et al. (2017). Fabrication of calcium phosphate-based nanocomposites incorporating DNA origami, gold nanorods, and anticancer drugs for biomedical applications. Adv. Healthc. Mater 6, 1700664. doi:10.1002/adhm.201700664
Zhang, L., Lu, Z., and Zhao, X. (2021). Targeting Bcl-2 for cancer therapy. Biochim. Biophys. Acta Rev. Cancer 1876, 188569. doi:10.1016/j.bbcan.2021.188569
Zhang, Q., Jiang, Q., Li, N., Dai, L., Liu, Q., Song, L., et al. (2014). DNA origami as an in vivo drug delivery vehicle for cancer therapy. ACS Nano 8, 6633–6643. doi:10.1021/nn502058j
Zhang, S., Lou, X. Y., Liu, L., and Yang, Y. W. (2023). The creation of DNA origami-based supramolecular nanostructures for cancer therapy. Adv. Healthc. Mater 2023, e2301066. doi:10.1002/adhm.202301066
Zhao, S., Duan, F., Liu, S., Wu, T., Shang, Y., Tian, R., et al. (2019). Efficient intracellular delivery of RNase A using DNA origami carriers. ACS Appl. Mater Interfaces 11, 11112–11118. doi:10.1021/acsami.8b21724
Zhao, Y. X., Shaw, A., Zeng, X., Benson, E., Nystrom, A. M., and Hogberg, B. (2012). DNA origami delivery system for cancer therapy with tunable release properties. ACS Nano 6, 8684–8691. doi:10.1021/nn3022662
Zhou, K., Ke, Y., and Wang, Q. (2018). Selective in situ assembly of viral protein onto DNA origami. J. Am. Chem. Soc. 140, 8074–8077. doi:10.1021/jacs.8b03914
Keywords: DNA origami, DNA nanostructures, anti-tumor drug delivery, cancer treatment, DNA self-assembly technique
Citation: Zhang Y, Tian X, Wang Z, Wang H, Liu F, Long Q and Jiang S (2023) Advanced applications of DNA nanostructures dominated by DNA origami in antitumor drug delivery. Front. Mol. Biosci. 10:1239952. doi: 10.3389/fmolb.2023.1239952
Received: 14 June 2023; Accepted: 27 July 2023;
Published: 07 August 2023.
Edited by:
Muling Zeng, Wuyi University, ChinaReviewed by:
Amelie Heuer-Jungemann, Max Planck Institute of Biochemistry, GermanyDhiraj Bhatia, Indian Institute of Technology Gandhinagar, India
Copyright © 2023 Zhang, Tian, Wang, Wang, Liu, Long and Jiang. This is an open-access article distributed under the terms of the Creative Commons Attribution License (CC BY). The use, distribution or reproduction in other forums is permitted, provided the original author(s) and the copyright owner(s) are credited and that the original publication in this journal is cited, in accordance with accepted academic practice. No use, distribution or reproduction is permitted which does not comply with these terms.
*Correspondence: Qipeng Long, longqipeng1990@163.com; Shulong Jiang, jnsljiang@163.com