Palmitoylation as a Key Regulator of Ras Localization and Function
- Department of Biological Chemistry, Laboratory of Chemical Biology, Institute of Advanced Chemistry of Catalonia (IQAC-CSIC), Barcelona, Spain
Ras proteins require membrane association for proper function. This process is tightly regulated by reversible palmitoylation that controls not only the distribution over different subcellular compartments but also Ras compartmentalization within membrane subdomains. As a result, there is a growing interest in protein palmitoylation and the enzymes that control this process. In this minireview, we discuss how palmitoylation affects the localization and function of Ras proteins. A better understanding of the regulatory mechanism controlling protein lipidation is expected to provide new insights into the functional role of these modifications and may ultimately lead to the development of novel therapeutic approaches.
Introduction
The Ras superfamily of small GTPases comprises more than 150 monomeric G proteins. Their ability to act as molecular switches upon stimulation by upstream signals, alternating between the GTP-bound active state and the inactive GDP-bound form, allows Ras proteins to play a role in a diverse array of biological processes such as cell proliferation, signaling, differentiation and survival (Simanshu et al., 2017). Some of the most prominent members of the Ras superfamily are the four Ras isoforms which are encoded by three different genes: H-Ras, N-Ras and K-Ras that generates two splice variants, K-Ras4A and K-Ras4B. The four isoforms share a highly conserved G domain but mainly differ in the hypervariable region (HVR) which comprises the last 24 amino acids and several posttranslational modifications. Hence, all proteins undergo a three-step maturation pathway at the C-terminus known as CaaX box processing (Lowy and Willumsen, 1989; Ahearn et al., 2012) which includes farnesylation of the cysteine, proteolytic cleavage of the last three amino acids (aaX) (Boyartchuk et al., 1997) and carboxyl methylation (Clarke et al., 1988; Dai et al., 1998). Since the prenyl moiety is essential but not sufficient to mediate the stable membrane association required for proper signaling, all isoforms display additional membrane targeting motifs (Hancock et al., 1990). N-Ras and H-Ras are both palmitoylated at either one or two cysteine residues, respectively. K-Ras4B contains a polybasic stretch of eight lysines and K-Ras4A presents a palmitoylated cysteine and two polybasic regions (Figure 1A). As a result of these differences, the four isoforms show distinct subcellular localization and distribution in membrane microdomains, and generate distinct signaling outputs (Rocks et al., 2005). However, other factors can also influence Ras signaling. Thus, apart from the HVR, the G-domain and its modifications (ubiquitination, sumoylation, acetylation, glucosylation and nitrosylation) may also contribute to a particular membrane orientation and isoform specific signaling (Kapoor et al., 2012; Ahearn et al., 2018). In addition, some functional redundancy has been suggested for the different isoforms, as although only K-Ras is essential for normal mouse embryogenesis, its function can be replaced by H-Ras, however, associated to significant cardiotoxicity (Potenza et al., 2005).
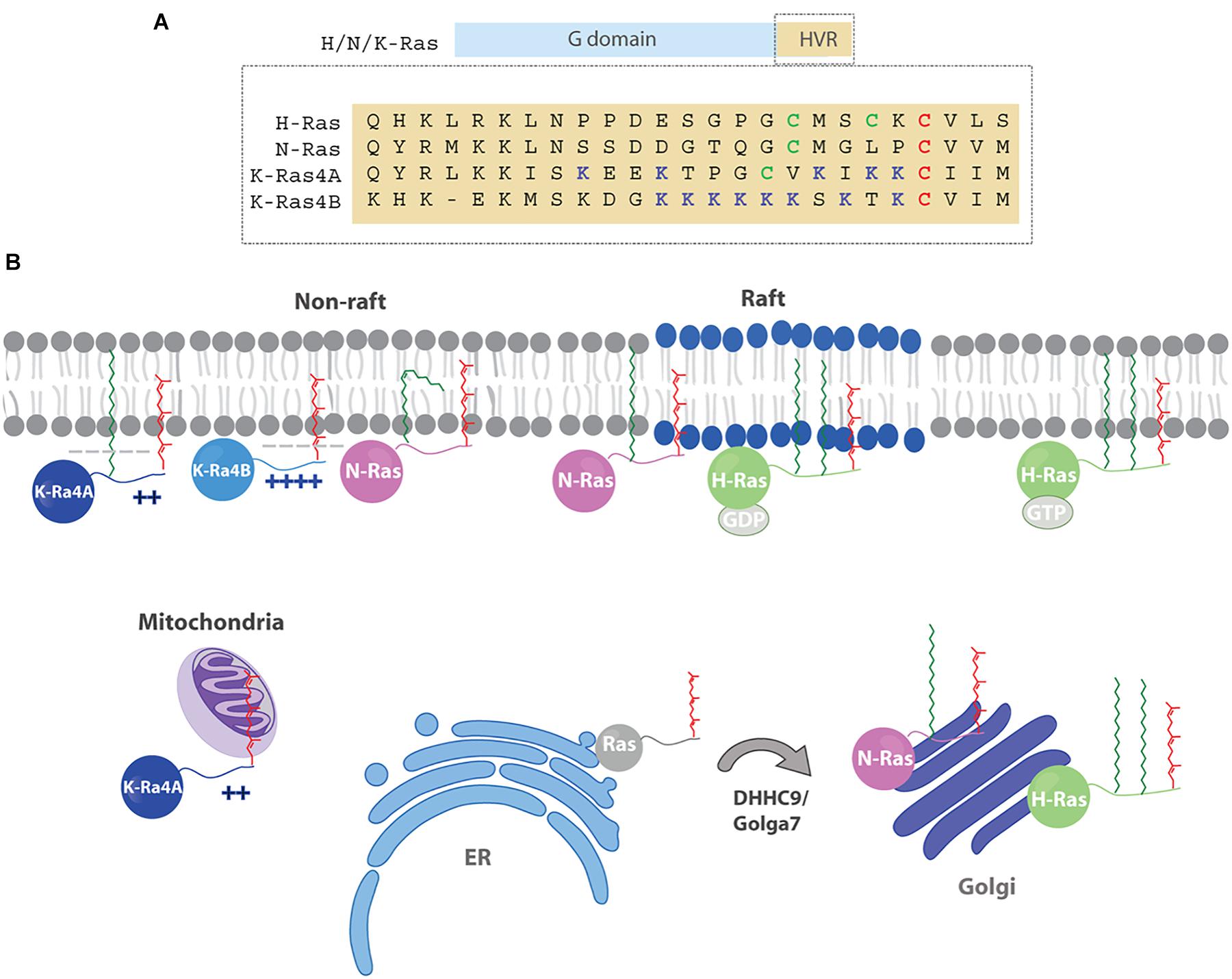
Figure 1. (A) The Ras isoforms contain a highly homologous G domain (90%) and a C-terminal hypervariable region (HVR) that comprises the last 24 amino acids. The HVR exhibit a low degree of homology between isoforms (∼ 10%) and present different post-translational lipid modifications. Red cysteines (C) are farnesylated, green cysteines (C) are palmitoylated, blue lysines (K) are polybasic residues. (B) Ras membrane distribution is dynamic and depends on membrane targeting motifs (polybasic sequences and lipids) and changes in palmitoylation state that combined, modulate Ras trafficking and localization to specific membranes (PM, endomembranes, subdomains). Hence, farnesylated H/N-Ras get palmitoylated at the Golgi apparatus by DHHC9/Golga7 and then are transferred to the PM via the secretory pathway. After depalmitoylation, H/N-Ras traffic back to the Golgi to be reacylated. Due to the presence of two palmitoyl moieties H-Ras gets enriched in the PM, whereas N-Ras is predominantly localized at the Golgi. Once in the membrane, H-Ras segregates in different microdomains (rafts, non-rafts) in a GDP/GTP dependent manner. Palmitoylated N-Ras associates preferentially with raft/non-raft boundary regions, although a N-Ras protein modified with an unsaturated palmitoleic shows preferential accumulation in fluid domains. The localization of K-Ras4A on the PM relies on the presence of polybasic regions and a palmitoylated cystine, whereas K-Ras4B is palmitoylation independent. After depalmitoylation, K-Ras4A travels to the mitochondria and binds HK1.
Ras proteins are among the most frequently altered oncogenes in human cancers (Hobbs et al., 2016) and overall, approximately 20% of cancer patients harbor Ras mutations (Prior et al., 2020). Point mutations occur in hotspots codons (mainly 12, 13, and 61) and lead to constitutively active proteins resulting in uncontrolled proliferation. However, the prevalence of each isoform in human cancers is not uniform. K-Ras is by far the most frequently mutated isoform (76%), whereas N-Ras contributes to the 17% of human cancers and H-Ras to the remaining 7%. Furthermore, each isoform is related to different types of cancer. While K-Ras is usually associated to lung, colorectal and pancreatic cancers, N-Ras is more predominant in skin melanomas and H-Ras in bladder carcinomas (Prior et al., 2012).
All the above mentioned factors reveal the increasing complexity of Ras biology. From one side, Ras signaling capacity and functional heterogeneity depends on specific isoforms and mutations. However, the extent to which the lipidation state determines the resident time, the specific subcellular localization or the partition into different membrane subdomains, and by doing so, it enables accessibility to a preferential set of effector proteins, is poorly understood. In this minireview we will discuss how changes in the acylation pattern influence the spatial and functional heterogeneity of Ras proteins.
Subcellular Localization and Function
H-Ras and N-Ras
The localization of palmitoylated isoforms is determined by the reversible nature of this modification. Although S-acyl groups of some proteins do not turn over or they do it at a very low rate, some other proteins, such as the Ras isoforms, show a very rapid cycling. Thus, after palmitoylation at the Golgi by palmitoyl acyl transferases (PATs) (Stix et al., 2020), N/H-Ras are transferred to the plasma membrane (PM) via the secretory pathway. In their way to the PM, fully palmitoylated and active H-/N-Ras can also localize at recycling endosomes (Misaki et al., 2010). Next, depalmitoylation is mediated by thioesterases (Won et al., 2018) and occurs everywhere in the cell. Depalmitoylated Ras then traffics back to the Golgi where it can be reacylated (Rocks et al., 2005, 2010; Figure 1B). Due to the presence of two fatty acid moieties, depalmitoylation of H-Ras takes longer causing enrichment at the PM, whereas N-Ras, bearing only one palmitate, is predominantly localized at the Golgi. Moreover, the combined action of PATs and thioesterases results in an acylation/deacylation cycle that has a shorter half-life than that of the protein (∼6 min for N-Ras and around 20 min for H-Ras vs. ∼24 h protein half-life) (Magee et al., 1987) and introduces an additional level of regulation in the spatial and temporal modulation of Ras signaling (Rocks et al., 2005, 2010). Interestingly, marked differences can exist in the turnover rates of oncogenic and wild type Ras, despite sharing similar subcellular localizations (Baker et al., 2003).
H-Ras
H-Ras gets palmitoylated by DHHC9/Golga7, a member of the Asp-His-His-Cys (DHHC) family of PATs that comprises 23 different proteins. Additional involvement of DHHC18 has also been suggested (Swarthout et al., 2005; Yokoi et al., 2016). Thioester cleavage was initially proven by APT1 (Duncan and Gilman, 1998), APT2 (Tomatis et al., 2010) and the lysosomal PPT1 (Camp and Hofmann, 1993; Verkruyse and Hofmann, 1996). The interaction of H-Ras with both APT1/2, mainly occurring at the PM, was also confirmed by FRET studies (Pedro et al., 2017). More recently, the involvement of other thioesterases has also been suggested since the disruption of APT1 gene in yeast did not completely abolished H-Ras deacylation (Duncan and Gilman, 2002). ABHD17, a member of the mammalian α,β hydrolase-domain (ABHD) family of serine hydrolases (SH) has been shown to deacylate an overexpressed H-Ras in HEK293T cells, but this effect could not be observed in neurons (Yokoi et al., 2016). As the SH family consists of over 100 members and most of them have not known substrate yet, it can not be discarded that additional thioesterases acting on H-Ras may be identified in the future.
Apart from the enzymes involved in de/acylation, FKBP12 may add an additional layer of regulation by controlling the time of residence of H-Ras at the PM. FKBP12 promotes the cis/trans isomerization of the peptidyl-prolyl bond at position 178–179, which facilitates depalmitoylation probably by rendering the thioester bond accessible to membrane associated thioesterases. Interaction of FKBP12 with N-Ras has also been detected, but not with K-Ras (Ahearn et al., 2011).
Some studies have suggested that the individual palmitoyl residues may have different roles. Thus, whereas a C184S mutant was present at both the PM and the Golgi, a C181S mutant was mostly localized at Golgi (Roy et al., 2005a). Moreover, the deacylation rate of the C184S mutant significantly increased upon overexpression of APT2, whereas the rate of the C181S mutant did not change (Pedro et al., 2017). Studies with monopalmitoylated mutants may shed light on the role and substrate specificity of these positions. However, results should be interpreted with caution since singly palmitoylated H-Ras species do not seem to be present in cells (Yokoi et al., 2016).
Because of the continuous cycle of de/acylation, H-Ras populations are present at and signal from both the PM and the Golgi apparatus under steady-state conditions. However, functional Ras can also signal from additional subcellular compartments, such as the Endoplasmic Reticulum (ER) (Chiu et al., 2002; Fehrenbacher et al., 2009) and the differential subcellular localizations contribute to its wide signaling repertoire. Thus, organelle-specific interaction with effectors may be behind the variety of biological responses observed, such as proliferation (Chiu et al., 2002; Arozarena et al., 2004) or apoptosis (Herrero et al., 2016; Casar et al., 2018). Studies with engineered proteins have provided insight into H-Ras biology and its relationship with effector proteins. Hence, an active H-Ras directed to Golgi or ER led to the correlation of signaling outputs with defined subcellular protein pools (Matallanas et al., 2006; Agudo-Ibáñez et al., 2007) and enabled the identification of organelle-specific protein-protein interactions (Santra et al., 2019). Specific interactions were also unveiled employing an engineered exchange factor able to activate different subcellular pools of endogenous H-Ras (Herrero et al., 2020). In addition, activation at distinct subcellular sites also provides a temporal control of signaling, that is transient and rapid at the PM but slower and sustained at Golgi.
N-Ras
The singly palmitoylated N-Ras is predominantly localized at the Golgi apparatus under steady-state conditions. Palmitoylation of N-Ras is also mediated by DHHC9/Golga7 and, similarly to H-Ras, N-Ras can be depalmitoylated by the broad substrate-tolerant APT1 and APT2 (Rocks et al., 2010; Görmer et al., 2012; Vartak et al., 2014). Depalmitoylated N-Ras is then transported to the Golgi by the chaperone PDE6δ (phosphodiesterase of retinal rod subunit δ) which binds the prenyl group and enhances the cytoplasmic diffusion of the protein. PDE6δ can also transport K-Ras4B (Chen et al., 2010) and facilitates its delivery to membranes (Weise et al., 2012), but has much less effect on H-Ras. The reason behind this selectivity may be the degree of palmitoylation that negatively affects binding with PDE6δ, as only 25% of H-Ras is depalmitoylated at steady-state compared to the 50% of N-Ras (Chandra et al., 2012; Zimmermann et al., 2013). Depletion of PDE6δ or small-molecule based inhibition of Ras-PDE6δ interaction results in Ras mislocalization and consequently, in attenuated signaling (Chandra et al., 2012; Zimmermann et al., 2013).
Because lipidation impairment causes Ras mislocalization, significant efforts have been made to identify thioesterase inhibitors that might be not only interesting for fundamental research but also offer potential applications in drug discovery (Table 1). As a result, small-molecule inhibitors of thioesterases have emerged as key players in the study of de/acylation processes. The first potent APT1/2 inhibitors were the β-lactones Palmostatin B and M that led to impaired localization and signaling of N-Ras and H-Ras (Dekker et al., 2010; Hedberg et al., 2011; Rusch et al., 2011). However, the role of APT1/2 was later questioned, since their overexpression showed little effect on N-Ras localization (Agudo-Ibáñez et al., 2015) and selective inhibitors of APT1 or APT2 could not preserve the palmitoylation state of N-Ras (Adibekian et al., 2010a,b). Currently, there are accumulating evidences indicating that other thioesterases might contribute to the regulation of N-Ras palmitate turnover. Relevant candidates are the three isoforms ABHD17A/B/C, localized to PM and Rab6- and Rab11-positive endosomes and also targeted by Palmostatin M (Lin and Conibear, 2015). Thus, overexpression of ABHD17 redistributed N-Ras from the PM to intracellular membranes and a selective inhibitor of ABHD17, ABD957, has shown to inhibit the growth of cells that depend on N-Ras as an oncogenic driver (Remsberg et al., 2020). However, since ABD957 only partially impairs N-Ras depalmitoylation, additional, yet unknown, thioesterases may not be discarded (Lin and Conibear, 2015). Apart from the cis/trans isomerase FKBP12 mentioned above, an additional chaperone protein, VPS35, has also been involved in the regulation of N-Ras subcellular trafficking (Zhou et al., 2016).
K-Ras
The K-Ras gene has two splice variants, K-Ras4A and K-Ras4B, both of them encoding oncogenic proteins when K-Ras is activated by mutation. It has been long considered that K-Ras4A was the minor splice variant and that its contribution to oncogenesis or tumor maintenance was negligible. However, RT-qPCR-based measurements revealed that K-Ras4A accounted for 10–50% of total K-Ras in cell lines derived from colon carcinoma and melanoma, and the relative abundance of K-Ras4A was even higher in primary human tumors (Tsai et al., 2015). All together, these recent advances have renewed the interest in the K-Ras4A isoform as a potential therapeutic target.
The two K-Ras splice variants have distinct mechanism of subcellular trafficking. Both variants require an essential farnesyl moiety, but localization and trafficking of K-Ras4B relies on the presence of polybasic residues that anchor the protein to the inner leaflet of the PM, whereas the membrane-targeting signals in K-Ras4A are two polybasic regions and an additional palmitoyl group, that independently contribute to the PM localization and signal output. Hence, only mutation of either region combined with loss of palmitoylation caused a significant reduction in ERK phosphorylation (Tsai et al., 2015) or abolished the ability to induce leukemia in mice (Zhao et al., 2015). Interestingly, in contrast to K-Ras4B and N-Ras, PDE6δ does not seem to function as a cytosolic chaperone for K-Ras4A (Tsai et al., 2015). K-Ras has also been implicated in the biogenesis of exosomes, tiny extracellular vesicles involved in cell-cell communication that have been also considered potential Ras signaling pathways (Sexton et al., 2019).
Recently, super-resolution immunofluorescence microscopy studies confirmed that the non-palmitoylated form of K-Ras4A also localizes on the outer mitochondrial membrane, where it specifically interacts with Hexokinase 1 (HK1), an enzyme that initiates glucose metabolism. Upon binding, K-Ras4A blocks the allosteric inhibition of HK1 resulting in an enhanced glucose consumption, which might contribute to the metabolic reprogramming of tumor cells aimed to sustain rapid tumor growth (Amendola et al., 2019). The interaction occurs only with the GTP-bound form and it requires the presence of the prenyl moiety but it is negatively regulated by palmitoylation. It is currently unknown which are the enzymes responsible for K-Ras4A de/acylation. However, it has been suggested that palmitoylation may be in charge of a PM-resident enzyme, whereas mitochondrial depalmitoylation could be performed by APT1 or ABHD10 (Cao et al., 2019). In addition, a third lipid modification could act as an additional regulatory mechanism for K-Ras4A. Hence, Lin et al. have shown that K-Ras4A can also be reversibly acylated with palmitic acid at lysine residues located at the HVR (K182/184/185). Lysine acylation occurs on fully lipidated proteins and lipid removal, that promotes its transforming activity, is mediated by Sirtuin 2 (Jing et al., 2017) and inhibited by JH-T4 (Spiegelman et al., 2019) (Table 1). Lysine acylation has been also detected on H-Ras (K170) and N-Ras, but these proteins are not substrates of Sirtuin 2. Further work is required to elucidate the role lysine acylation on H-/N-Ras and to identify the enzymes that control this process.
Membrane Microdomain Localization of Ras Proteins
Lipidation not only regulates the subcellular localization of Ras proteins, but also its lateral segregation and distribution between membrane microdomains, which is crucial for efficient signal transmission. Thus, the lateral heterogenous composition of cellular membranes results in the transient formation of distinct subcompartments: packed domains enriched with cholesterol and sphingolipids referred to as lipid ordered (lo) domains o rafts, and more fluid domains termed liquid disordered (ld) domains or non-rafts. In this second part, we will give a brief overview on Ras segregation in membrane subdomains (for a more detailed description see Erwin et al., 2017 and references herein).
Initial studies by Hancock et al. showed that H-Ras segregation within membrane microdomains was GDP/GTP-dependent. H-Ras resides in lipid rafts in its inactive form, but the active GTP-bound form as well as the active mutant HRasV12 migrate to ld membranes (Prior et al., 2001; Rotblat et al., 2004), where they can activate proliferation and differentiation (Herrero et al., 2016; Figure 1B). However, GDP/GTP-dependent H-Ras partitioning may also be cell-specific (Agudo-Ibáñez et al., 2015). These different lateral segregations might regulate the biological output of H-Ras by a yet unknown mechanism. Thus, Ras signaling from rafts results in phosphorylation of epidermal growth factor receptor and cytosolic phospholipase A2, whereas signaling from fluid domains causes activation of kinase suppressor of Ras 1 (Casar et al., 2009). Moreover, H/N-Ras signals from lipid rafts or ER yield big tumors but with a reduced propensity to disseminate, whereas signaling from Golgi and disordered regions displayed higher migration rates (Agudo-Ibáñez et al., 2015; García-Ibáñez et al., 2020).
The effect of lipidation in membrane partitioning and clustering behavior of N-Ras has also been widely characterized. Initial studies in homogeneous membranes showed the formation of dimers (Güldenhaupt et al., 2012), that could be an initial step to the formation of small nanoclusters (Erwin et al., 2016). More complex systems gave, however, controversial results. Thus, the first insight into N-Ras microlocalization suggested that N-Ras was mainly found in rafts (Matallanas et al., 2003) at least in its GTP-form (Prior et al., 2001; Roy et al., 2005b).
A major advancement in the field came with the development of semisynthetic methods to obtain fully lipidated Ras proteins in quantities enabling biophysical studies (Triola et al., 2012). Hence, atomic force microscopy studies performed in heterogeneous model membranes revealed that N-Ras partitioning occurs preferentially into ld domains followed by time-dependent clustering in domain (lo/ld) boundaries regions (Weise et al., 2009, 2010). No significant GDP/GTP-dependent partitioning was observed, although the inactive form showed stronger membrane association (Gohlke et al., 2010). Moreover, upon membrane binding, N-Ras showed free rotation of the G-domain, what may facilitate its interaction with effectors (Werkmuller et al., 2013). Accumulation in lo/ld phase boundaries could also be observed in viral membrane extracts (Vogel et al., 2009) or using a FRET-based study (Shishina et al., 2018).
Recent breakthroughs have developed more sensitives techniques that revealed that N-Ras S-acylation is not restricted to the saturated palmitate but also includes the unsaturated palmitoleate. Characterization of palmitoleated N-Ras distribution in model membranes indicated a different behavior compared to the saturated N-Ras, showing a rapid membrane insertion and preferentially clustering in the ld domains. Interestingly, these results suggest that S-acylation with different fatty acids may be an additional regulation point in N-Ras signaling (Schulte-Zweckel et al., 2019). The existence of thioesterases or PATs specifically committed to palmitoleated N-Ras remains elusive (Schulte-Zweckel et al., 2019). However, the fact that the 23 DHHCs have shown marked differences in fatty acid selectivity might suggest some substrate specificity (Greaves et al., 2017).
Currently, there is no information about the lateral segregation behavior of K-Ras4A. On the contrary, the splice variant K-Ras4B is better characterized. Thus, the polybasic K-Ras 4B preferentially distributes in ld domains and spontaneous assembles to form new domains containing proteins and lipids (Weise et al., 2011). The presence of the prenyl group combined with the precise amino acid sequence of the polybasic region define the lipid composition of these nanoclusters (Zhou et al., 2017). The enrichments was independent of GDP/GTP loading but the active form showed bigger clusters (Kapoor et al., 2012). K-Ras4B distribution on the ld domain was also observed in GUVs made from the envelope membrane of viral lipids (Weise et al., 2011) and protein-containing GPMVs (Erwin et al., 2016). Transport to the membrane is mediated by PDE6δ, whereas phosphorylation at Ser181 facilitates the PM dissociation (Zhang et al., 2017). Extraction from negatively charged membranes can also be performed in a GDP/GTP-independent manner by Calmodulin (Sperlich et al., 2016).
Conclusions and Perspectives
It is becoming clear that the differential spatiotemporal distribution on organelles and subdomains has a key role in regulating the functional versatility of Ras proteins. As reversible lipidation is critical for maintaining the correct localization of H-/N- and probably KRas4A, a better understanding of the mechanism and dynamics by which S-acylation is controlled will provide new insights into the functional role of these modifications. Major outstanding questions still remain unanswered, such as how is the dynamic of lipid turnover regulated, how the presence of saturated or unsaturated fatty acids may influence protein function, which are all the enzymes involved in de/acylation and their selectivity profile or whether changes in the S-acylation (turnover rate, fatty acid identity) are linked with specific disease states. Furthermore, an increase in our knowledge of the mechanism and outcomes of protein S-acylation could lead to the identification of novel therapeutic opportunities.
Author Contributions
CB-H wrote the manuscript. GT wrote and revised the manuscript. Both authors contributed to the article and approved the submitted version.
Funding
Financial support from the Caixa Foundation under the agreement LCF/PR/HR20/52400006 and the Ministerio de Ciencia e Innocación (RTI2018-096323-b-100) are gratefully acknowledged.
Conflict of Interest
The authors declare that the research was conducted in the absence of any commercial or financial relationships that could be construed as a potential conflict of interest.
Acknowledgments
We acknowledge support of the publication fee by the CSIC Open Access Publication Support Initiative through its Unit of Information Resources for Research (URICI). We apologize to all the colleagues whose relevant work could not be cited due to space restrictions.
References
Adibekian, A., Martin, B. R., Chang, J. W., Hsu, K. L., Tsuboi, K., Bachovchin, D. A., et al. (2010a). “Characterization of a Selective, Reversible Inhibitor of Lysophospholipase 1 (LYPLA1),” in Probe Reports from the NIH Molecular Libraries Program. Bethesda, MD: National Center for Biotechnology Information.
Adibekian, A., Martin, B. R., Chang, J. W., Hsu, K. L., Tsuboi, K., Bachovchin, D. A., et al. (2010b). “Characterization of a Selective, Reversible Inhibitor of Lysophospholipase 2 (LYPLA2),” in Probe Reports from the NIH Molecular Libraries Program. Bethesda, MD: National Center for Biotechnology Information.
Agudo-Ibáñez, L., Herrero, A., Barbacid, M., and Crespo, P. (2015). H-Ras distribution and signaling in plasma membrane microdomains are regulated by acylation and deacylation events. Mol. Cell. Biol. 35, 1898–1914. doi: 10.1128/mcb.01398-14
Agudo-Ibáñez, L., Núñez, F., Calvo, F., Berenjeno, I. M., Bustelo, X. R., and Crespo, P. (2007). Transcriptomal profiling of site-specific Ras signals. Cell. Signal. 19, 2264–2276. doi: 10.1016/j.cellsig.2007.06.025
Ahearn, I., Zhou, M., and Philips, M. R. (2018). Posttranslational modifications of RAS proteins. Cold Spring Harb. Perspect. Med. 8:a031484. doi: 10.1101/cshperspect.a031484
Ahearn, I. M., Haigis, K., Bar-Sagi, D., and Philips, M. R. (2012). Regulating the regulator: post-translational modification of RAS. Nat. Rev. Mol. Cell Biol. 13, 39–51. doi: 10.1038/nrm3255
Ahearn, I. M., Tsai, F. D., Court, H., Zhou, M., Jennings, B. C., Ahmed, M., et al. (2011). FKBP12 binds to acylated h-ras and promotes depalmitoylation. Mol. Cell. 41, 173–185. doi: 10.1016/j.molcel.2011.01.001
Amendola, C. R., Mahaffey, J. P., Parker, S. J., Ahearn, I. M., Chen, W. C., Zhou, M., et al. (2019). KRAS4A directly regulates hexokinase 1. Nature 576, 482–486. doi: 10.1038/s41586-019-1832-9
Arozarena, I., Matallanas, D., Berciano, M. T., Sanz-Moreno, V., Calvo, F., Muñoz, M. T., et al. (2004). Activation of H-Ras in the endoplasmic reticulum by the rasGRF family guanine nucleotide exchange factors. Mol. Cell. Biol. 24, 1516–1530. doi: 10.1128/mcb.24.4.1516-1530.2004
Baker, T. L., Zheng, H., Walker, J., Coloff, J. L., and Buss, J. E. (2003). Distinct rates of palmitate turnover on membrane-bound cellular and oncogenic H-Ras. J. Biol. Chem. 278, 19292–19300. doi: 10.1074/jbc.M206956200
Boyartchuk, V. L., Ashby, M. N., and Rine, J. (1997). Modulation of ras and a-factor function by carboxyl-terminal proteolysis. Science 275, 1796–1800.
Camp, L. A., and Hofmann, S. L. (1993). Purication and properties of a palmitoyl-protein thioesterase that cleaves palmitate from H-Ras. J. Biol. Chem. 268, 22566–22574.
Cao, Y., Qiu, T., Kathayat, R. S., Azizi, S. A., Thorne, A. K., Ahn, D., et al. (2019). ABHD10 is an S-depalmitoylase affecting redox homeostasis through peroxiredoxin-5. Nat. Chem. Biol. 15, 1232–1240. doi: 10.1038/s41589-019-0399-y
Casar, B., Arozarena, I., Sanz-Moreno, V., Pinto, A., Agudo-Ibáñez, L., Marais, R., et al. (2009). Ras subcellular localization defines extracellular signal-regulated kinase 1 and 2 substrate specificity through distinct utilization of scaffold proteins. Mol. Cell. Biol. 29, 1338–1353. doi: 10.1128/mcb.01359-08
Casar, B., Badrock, A. P., Jiménez, I., Arozarena, I., Colón-Bolea, P., Lorenzo-Martín, L. F., et al. (2018). RAS at the Golgi antagonizes malignant transformation through PTPRκ-mediated inhibition of ERK activation. Nat. Commun. 9:3595. doi: 10.1038/s41467-018-05941-8
Chandra, A., Grecco, H. E., Pisupati, V., Perera, D., Cassidy, L., Skoulidis, F., et al. (2012). The GDI-like solubilizing factor PDEδ sustains the spatial organization and signalling of Ras family proteins. Nat. Cell Biol. 14, 148–158. doi: 10.1038/ncb2394
Chen, Y.-X., Koch, S., Uhlenbrock, K., Weise, K., Das, D., Gremer, L., et al. (2010). Synthesis of the Rheb and K-Ras4B GTPases. Angew. Chemie Int. Ed Engl. 49, 6090–6095. doi: 10.1002/anie.201001884
Chiu, V. K., Bivona, T., Hach, A., Sajous, J. B., Silletti, J., Wiener, H., et al. (2002). Ras signalling on the endoplasmic reticulum and the Golgi. Nat. Cell. Biol. 4, 343–350. doi: 10.1038/ncb783
Clarke, S., Vogel, J. P., Deschenes, R. J., and Stock, J. (1988). Posttranslational modification of the ha-ras oncogene protein: evidence for a third class of protein carboxyl methyltransferases. Proc. Natl. Acad. Sci. U. S. A. 85, 4643–4647.
Dai, Q., Choy, E., Chiu, V., Romano, J., Slivka, S. R., Steitz, S. A., et al. (1998). Mammalian prenylcysteine carboxyl methyltransferase is in the endoplasmic reticulum. J. Biol. Chem. 273, 15030–15034.
Dekker, F. J., Rocks, O., Vartak, N., Menninger, S., Hedberg, C., Balamurugan, R., et al. (2010). Small-molecule inhibition of APT 1 affects Ras localization and signaling. Nat. Chem. Biol. 6, 449–456.
Duncan, J. A., and Gilman, A. G. (1998). A cytoplasmic acyl-protein thioesterase that removes palmitate from G protein α subunits and p21RAS. J. Biol. Chem. 273, 15830–15837. doi: 10.1074/jbc.273.25.15830
Duncan, J. A., and Gilman, A. G. (2002). Characterization of Saccharomyces cerevisiae acyl-protein thioesterase 1, the enzyme responsible for G protein alpha subunit deacylation in vivo. J. Biol. Chem. 277, 31740–31752. doi: 10.1074/jbc.M202505200
Erwin, N., Patra, S., Dwivedi, M., Weise, K., and Winter, R. (2017). Influence of isoform-specific Ras lipidation motifs on protein partitioning and dynamics in model membrane systems of various complexity. Biol. Chem. 398, 547–563. doi: 10.1515/hsz-2016-0289
Erwin, N., Sperlich, B., Garivet, G., Waldmann, H., Weise, K., and Winter, R. (2016). Lipoprotein insertion into membranes of various complexity: Lipid sorting, interfacial adsorption and protein clustering. Phys. Chem. Chem. Phys. 18, 8954–8962. doi: 10.1039/c6cp00563b
Fehrenbacher, N., Bar-Sagi, D., and Philips, M. (2009). Ras/MAPK signaling from endomembranes. Mol. Oncol. 3, 297–307. doi: 10.1016/j.molonc.2009.06.004
García-Ibáñez, Y., Riesco-Eizaguirre, G., Santisteban, P., Casar, B., and Crespo, P. (2020). RAS subcellular localization inversely regulates thyroid tumor growth and dissemination. Cancers (Basel). 12:2588. doi: 10.3390/cancers12092588
Gohlke, A., Triola, G., Waldmann, H., and Winter, R. (2010). Influence of the lipid anchor motif of N-Ras on the interaction with lipid membranes: a surface plasmon resonance study. Biophys. J. 98, 2226–2235. doi: 10.1016/j.bpj.2010.02.005
Görmer, K., Bürger, M., Kruijtzer, J. A. W., Vetter, I., Vartak, N., Brunsveld, L., et al. (2012). Chemical-biological exploration of the limits of the ras de- and repalmitoylating machinery. Chem. BioChem. 13, 1017–1023. doi: 10.1002/cbic.201200078
Greaves, J., Munro, K. R., Davidson, S. C., Riviere, M., Wojno, J., Smith, T. K., et al. (2017). Molecular basis of fatty acid selectivity in the zDHHC family of S-acyltransferases revealed by click chemistry. Proc. Natl. Acad. Sci. 114:201612254. doi: 10.1073/pnas.1612254114
Güldenhaupt, J., Rudack, T., Bachler, P., Mann, D., Triola, G., Waldmann, H., et al. (2012). N-Ras forms dimers at POPC membranes. Biophys. J. 103, 1585–1593. doi: 10.1016/j.bpj.2012.08.043
Hancock, J. P., Paterson, H., and Marshall, C. J. (1990). A polybasic domain or palmitoylation is required in addition to the CAAX motif to localize p21ras to the plasma membrane. Cell 63, 133–139.
Hedberg, C., Dekker, F. J., Rusch, M., Renner, S., Wetzel, S., Vartak, N., et al. (2011). Development of highly potent inhibitors of the ras-targeting human acyl protein thioesterases based on substrate similarity design. Angew. Chem. Int. Ed Engl. 50, 9832–9837. doi: 10.1002/anie.201102965
Herrero, A., Casar, B., Colón-Bolea, P., Agudo-Ibáñez, L., and Crespo, P. (2016). Defined spatiotemporal features of RAS-ERK signals dictate cell fate in MCF-7 mammary epithelial cells. Mol. Biol. Cell. 27, 1958–1968. doi: 10.1091/mbc.E15-02-0118
Herrero, A., Reis-Cardoso, M., Jiménez-Gómez, I., Doherty, C., Agudo-Ibañez, L., Pinto, A., et al. (2020). Characterisation of HRas local signal transduction networks using engineered site-specific exchange factors. Small GTPases 11, 371–383. doi: 10.1080/21541248.2017.1406434
Hobbs, G. A., Der, C. J., and Rossman, K. L. (2016). RAS isoforms and mutations in cancer at a glance. J. Cell Sci. 129, 1287–1292. doi: 10.1242/jcs.182873
Jing, H., Zhang, X., Wisner, S. A., Chen, X., Spiegelman, N. A., Linder, M. E., et al. (2017). SIRT2 and lysine fatty acylation regulate the transforming activity of K-Ras4a. Elife 6:e32436. doi: 10.7554/eLife.32436
Kapoor, S., Weise, K., Erlkamp, M., Triola, G., Waldmann, H., and Winter, R. (2012). The role of G-domain orientation and nucleotide state on the Ras isoform-specific membrane interaction. Eur. Biophys. J. 41, 801–813. doi: 10.1007/s00249-012-0841-5
Lin, D. T., and Conibear, E. (2015). ABHD17 proteins are novel protein depalmitoylases that regulate N-Ras palmitate turnover and subcellular localization. Elife 4:e11306. doi: 10.7554/eLife.11306
Magee, A. I., Gutierrez, L., Mckay, I. A., Marshall, C. J., and Hall, A. (1987). Dynamic fatty acylation of P21n-Ras. Embo J. 6, 3353–3357.
Matallanas, D., Arozarena, I., Berciano, M. T., Aaronson, D. S., Pellicer, A., Lafarga, M., et al. (2003). Differences on the inhibitory specificities of H-Ras, K-Ras, and N-Ras (N17) dominant negative mutants are related to their membrane microlocalization. J. Biol. Chem. 278, 4572–4581. doi: 10.1074/jbc.M209807200
Matallanas, D., Sanz-Moreno, V., Arozarena, I., Calvo, F., Agudo-Ibáñez, L., Santos, E., et al. (2006). Distinct utilization of effectors and biological outcomes resulting from site-specific ras activation: ras functions in lipid rafts and golgi complex are dispensable for proliferation and transformation. Mol. Cell. Biol. 26, 100–116. doi: 10.1128/mcb.26.1.100-116.2006
Misaki, R., Morimatsu, M., Uemura, T., Waguri, S., Miyoshi, E., Taniguchi, N., et al. (2010). Palmitoylated ras proteins traffic through recycling endosomes to the plasma membrane during exocytosis. J. Cell Biol. 191, 23–29. doi: 10.1083/jcb.200911143
Pedro, M. P., Vilcaes, A. A., Gomez, G. A., and Daniotti, J. L. (2017). Individual S-acylated cysteines differentially contribute to H-Ras endomembrane traffcking and acylation/deacylation cycles. Mol. Biol. Cell. 28, 962–974. doi: 10.1091/mbc.E16-08-0603
Potenza, N., Vecchione, C., Notte, A., De Rienzo, A., Rosica, A., Bauer, L., et al. (2005). Replacement of K-Ras with H-Ras supports normal embryonic development despite inducing cardiovascular pathology in adult mice. EMBO Rep. 6, 432–437. doi: 10.1038/sj.embor.7400397
Prior, I. A., Harding, A., Yan, J., Sluimer, J., Parton, R. G., and Hancock, J. F. (2001). GTP-dependent segregation of H-ras from lipid rafts is required for biological activity. Nat. Cell Biol. 3, 368–375. doi: 10.1038/35070050
Prior, I. A., Hood, F. E., and Hartley, J. L. (2020). The frequency of ras mutations in cancer. Cancer Res. 80, 2969–2974. doi: 10.1158/0008-5472.CAN-19-3682
Prior, I. A., Lewis, P. D., and Mattos, C. (2012). A comprehensive survey of ras mutations in cancer. Cancer Res. 72, 2457–2467. doi: 10.1158/0008-5472.CAN-11-2612
Remsberg, J. R., Suciu, R. M., Zambetti, N. A., Hanigan, T. W., Firestone, A. J., Inguva, A., et al. (2020). ABHD17 enzymes regulate dynamic plasma membrane palmitoylation and N-Ras-dependent cancer growth. bioRxiv 2020:108316. doi: 10.1101/2020.05.21.108316
Rocks, O., Gerauer, M., Vartak, N., Koch, S., Huang, Z. P., Pechlivanis, M., et al. (2010). The palmitoylation machinery is a spatially organizing system for peripheral membrane proteins. Cell 141, 458–471.
Rocks, O., Peyker, A., Kahms, M., Verveer, P. J., Koerner, C., Lumbierres, M., et al. (2005). An acylation cycle regulates localization and activity of palmitoylated Ras isoforms. Science 307, 1746–1752.
Rotblat, B., Prior, I. A., Muncke, C., Parton, R. G., Kloog, Y., Henis, Y. I., et al. (2004). Three separable domains regulate GTP-dependent association of H-ras with the plasma membrane. Mol. Cell. Biol. 24, 6799–6810. doi: 10.1128/MCB.24.15.6799-6810.2004
Roy, S., Plowman, S., Rotblat, B., Prior, I. A., Muncke, C., Grainger, S., et al. (2005a). Individual palmitoyl residues serve distinct roles in H-Ras trafficking, microlocalization, and signaling. Mol. Cell. Biol. 25, 6722–6733. doi: 10.1128/mcb.25.15.6722-6733.2005
Roy, S., Plowman, S., Rotblat, B., Prior, I. A., Muncke, C., Grainger, S., et al. (2005b). Individual palmitoyl residues serve distinct roles in H-Ras trafficking, microlocalization, and signaling. Mol. Cell. Biol. 25, 6722–6733.
Rusch, M., Zimmermann, T. J., Bürger, M., Dekker, F. J., Görmer, K., Triola, G., et al. (2011). Identification of acyl protein thioesterases 1 and 2 as the cellular targets of the ras-signaling modulators palmostatin B and M. Angew. Chemie Int. Ed Engl. 50, 9838–9842. doi: 10.1002/anie.201102967
Santra, T., Herrero, A., Rodriguez, J., von Kriegsheim, A., Iglesias-Martinez, L. F., Schwarzl, T., et al. (2019). An integrated global analysis of compartmentalized hras signaling. Cell. Rep. 26, 3100–3115. doi: 10.1016/j.celrep.2019.02.038
Schulte-Zweckel, J., Dwivedi, M., Brockmeyer, A., Janning, P., Winter, R., and Triola, G. (2019). A hydroxylamine probe for profiling S-acylated fatty acids on proteins †. Chem. Commun 55:11183. doi: 10.1039/c9cc05989j
Sexton, R. E., Mpilla, G., Kim, S., Philip, P. A., and Azmi, A. S. (2019). Ras and exosome signaling. Semin. Cancer Biol. 54, 131–137. doi: 10.1016/j.semcancer.2019.02.004
Shishina, A. K., Kovrigina, E. A., Galiakhmetov, A. R., Rathore, R., and Kovrigin, E. L. (2018). Study of förster resonance energy transfer to lipid domain markers ascertains partitioning of semisynthetic lipidated N-Ras in lipid raft nanodomains. Biochemistry 57, 872–881. doi: 10.1021/acs.biochem.7b01181
Simanshu, D. K., Nissley, D. V., and McCormick, F. (2017). RAS proteins and their regulators in human disease. Cell 170, 17–33. doi: 10.1016/j.cell.2017.06.009
Sperlich, B., Kapoor, S., Waldmann, H., Winter, R., and Weise, K. (2016). Regulation of K-Ras4B membrane binding by calmodulin. Biophys. J. 111, 113–122. doi: 10.1016/j.bpj.2016.05.042
Spiegelman, N. A., Hong, J. Y., Hu, J., Jing, H., Wang, M., Price, I. R., et al. (2019). A small-molecule SIRT2 inhibitor that promotes K-Ras4a lysine fatty-acylation. Chem. MedChem. 14, 744–748. doi: 10.1002/cmdc.201800715
Stix, R., Lee, C. J., Faraldo-Gómez, J. D., and Banerjee, A. (2020). Structure and mechanism of DHHC protein acyltransferases. J. Mol. Biol. 432, 4983–4998. doi: 10.1016/j.jmb.2020.05.023
Swarthout, J. T., Lobo, S., Farh, L., Croke, M. R., Greentree, W. K., Deschenes, R. J., et al. (2005). DHHC9 and GCP16 constitute a human protein fatty acyltransferase with specificity for H- and N-Ras. J. Biol. Chem. 280, 31141–31148. doi: 10.1074/jbc.M504113200
Tomatis, V. M., Trenchi, A., Gomez, G. A., and Daniotti, J. L. (2010). Acyl-protein thioesterase 2 catalizes the deacylation of peripheral membrane-associated GAP-43. PLoS One 5:e15045. doi: 10.1371/journal.pone.0015045
Triola, G., Waldmann, H., and Hedberg, C. (2012). Chemical biology of lipidated proteins. ACS Chem. Biol. 7, 87–99. doi: 10.1021/cb200460u
Tsai, F. D., Lopes, M. S., Zhou, M., Court, H., Ponce, O., Fiordalisi, J. J., et al. (2015). K-Ras4A splice variant is widely expressed in cancer and uses a hybrid membrane-targeting motif. Proc. Natl. Acad. Sci. U S A. 112, 779–784. doi: 10.1073/pnas.1412811112
Vartak, N., Papke, B., Grecco, H. E., Rossmannek, L., Waldmann, H., Hedberg, C., et al. (2014). The autodepalmitoylating activity of APT maintains the spatial organization of palmitoylated membrane proteins. Biophys. J. 106, 93–105. doi: 10.1016/j.bpj.2013.11.024
Verkruyse, L. A., and Hofmann, S. L. (1996). Lysosomal targeting of palmitoyl-protein thioesterase. J. Biol. Chem. 271, 15831–15836.
Vogel, A., Reuther, G., Weise, K., Triola, G., Nikolaus, J., Tan, K.-T., et al. (2009). The lipid modifications of ras that sense membrane environments and induce local enrichment. Angew. Chemie Int. Ed Engl. 48, 8784–8787. doi: 10.1002/anie.200903396
Weise, K., Kapoor, S., Denter, C., Nikolaus, J., Opitz, N., Koch, S., et al. (2011). Membrane-mediated induction and sorting of K-Ras microdomain signaling platforms. J. Am. Chem. Soc. 133, 880–887. doi: 10.1021/ja107532q
Weise, K., Kapoor, S., Werkmüller, A., Möbitz, S., Zimmermann, G., Triola, G., et al. (2012). Dissociation of the K-Ras4B/PDEδ complex upon contact with lipid membranes: MEMBRANE delivery instead of extraction. J. Am. Chem. Soc. 134, 11503–11510. doi: 10.1021/ja305518h
Weise, K., Triola, G., Brunsveld, L., Waldmann, H., and Winter, R. (2009). Influence of the lipidation motif on the partitioning and association of N-ras in model membrane subdomains. J. Am. Chem. Soc. 131, 1557–1564. doi: 10.1021/ja808691r
Weise, K., Triola, G., Janosch, S., Waldmann, H., and Winter, R. (2010). Visualizing association of lipidated signaling proteins in heterogeneous membranes-partitioning into subdomains, lipid sorting, interfacial adsorption, and protein association. Biochim. Biophys. Acta 1798, 1409–1417. doi: 10.1016/j.bbamem.2009.12.006
Werkmuller, A., Triola, G., Waldmann, H., and Winter, R. (2013). Rotational and translational dynamics of ras proteins upon binding to model membrane systems. Chemphyschem 14, 3698–3705. doi: 10.1002/cphc.201300617
Won, S. J., Cheung See Kit, M., and Martin, B. R. (2018). Protein depalmitoylases. Crit. Rev. Biochem. Mol. Biol. 53, 83–98. doi: 10.1080/10409238.2017.1409191
Yokoi, N., Fukata, Y., Sekiya, A., Murakami, T., Kobayashi, K., and Fukata, M. (2016). Identification of PSD-95 Depalmitoylating Enzymes. J. Neurosci. 36, 6431–6444. doi: 10.1523/JNEUROSCI.0419-16.2016
Zhang, S. Y., Sperlich, B., Li, F. Y., Al-Ayoubi, S., Chen, H. X., Zhao, Y. F., et al. (2017). Phosphorylation weakens but does not inhibit membrane binding and clustering of K-Ras4B. ACS Chem. Biol. 12, 1703–1710. doi: 10.1021/acschembio.7b00165
Zhao, H., Liu, P., Zhang, R., Wu, M., Li, D., Zhao, X., et al. (2015). Roles of palmitoylation and the KIKK membrane-targeting motif in leukemogenesis by oncogenic KRAS4A. J. Hematol. Oncol. 8:132. doi: 10.1186/s13045-015-0226-1
Zhou, M., Wiener, H., Su, W., Zhou, Y., Liot, C., Ahearn, I., et al. (2016). VPS35 binds farnesylated N-Ras in the cytosol to regulate N-Ras trafficking. J. Cell. Biol. 214, 445–458. doi: 10.1083/jcb.201604061
Zhou, Y., Prakash, P., Liang, H., Cho, K. J., Gorfe, A. A., and Hancock, J. F. (2017). Lipid-sorting specificity encoded in K-Ras membrane anchor regulates signal output. Cell 168, 239–251. doi: 10.1016/j.cell.2016.11.059
Keywords: Ras, palmitoylation, acylation, lipid posttranslational modifications, cancer, membrane subdomains, thioesterases
Citation: Busquets-Hernández C and Triola G (2021) Palmitoylation as a Key Regulator of Ras Localization and Function. Front. Mol. Biosci. 8:659861. doi: 10.3389/fmolb.2021.659861
Received: 28 January 2021; Accepted: 22 February 2021;
Published: 17 March 2021.
Edited by:
Piero Crespo, Instituto de Biomedicina y Biotecnología de Cantabria, Consejo Superior de Investigaciones Científicas, SpainReviewed by:
David Santamaria, Spanish National Cancer Research Center (CNIO), SpainLorena Agudo Ibáñez, Centro de Investigación Biomédica en Red del Cáncer (CIBERONC), Spain
Copyright © 2021 Busquets-Hernández and Triola. This is an open-access article distributed under the terms of the Creative Commons Attribution License (CC BY). The use, distribution or reproduction in other forums is permitted, provided the original author(s) and the copyright owner(s) are credited and that the original publication in this journal is cited, in accordance with accepted academic practice. No use, distribution or reproduction is permitted which does not comply with these terms.
*Correspondence: Gemma Triola, gemma.triola@iqac.csic.es