- 1Brazilian Biorenewables National Laboratory (LNBR), Brazilian Center for Research in Energy and Materials (CNPEM), Campinas, Brazil
- 2Graduate Program in Genetics and Molecular Biology, Institute of Biology, University of Campinas (UNICAMP), Campinas, Brazil
- 3Molecular Phytobacteriology Laboratory, Korea Research Institute of Bioscience & Biotechnology (KRIBB), Daejeon, South Korea
- 4Biosystems and Bioengineering Program, University of Science and Technology, Daejeon, South Korea
Plant diseases caused by phytopathogens result in huge economic losses in agriculture. In addition, the use of chemical products to control such diseases causes many problems to the environment and to human health. However, some bacteria and fungi have a mutualistic relationship with plants in nature, mainly exchanging nutrients and protection. Thus, exploring those beneficial microorganisms has been an interesting and promising alternative for mitigating the use of agrochemicals and, consequently, achieving a more sustainable agriculture. Microorganisms are able to produce and excrete several metabolites, but volatile organic compounds (VOCs) have huge biotechnology potential. Microbial VOCs are small molecules from different chemical classes, such as alkenes, alcohols, ketones, organic acids, terpenes, benzenoids and pyrazines. Interestingly, volatilomes are species-specific and also change according to microbial growth conditions. The interaction of VOCs with other organisms, such as plants, insects, and other bacteria and fungi, can cause a wide range of effects. In this review, we show that a large variety of plant pathogens are inhibited by microbial VOCs with a focus on the in vitro and in vivo inhibition of phytopathogens of greater scientific and economic importance in agriculture, such as Ralstonia solanacearum, Botrytis cinerea, Xanthomonas and Fusarium species. In this scenario, some genera of VOC-producing microorganisms stand out as antagonists, including Bacillus, Pseudomonas, Serratia and Streptomyces. We also highlight the known molecular and physiological mechanisms by which VOCs inhibit the growth of phytopathogens. Microbial VOCs can provoke many changes in these microorganisms, such as vacuolization, fungal hyphal rupture, loss of intracellular components, regulation of metabolism and pathogenicity genes, plus the expression of proteins important in the host response. Furthermore, we demonstrate that there are aspects to investigate by discussing questions that are still not very clear in this research area, especially those that are essential for the future use of such beneficial microorganisms as biocontrol products in field crops. Therefore, we bring to light the great biotechnological potential of VOCs to help make agriculture more sustainable.
Introduction
World population growth is expected to reach almost 10 billion people by 2050 and one of the major concerns relies on the food insecurity that may accompany it (United Nations, 2022). The issue becomes even more complex since the most important crops (e.g., rice, wheat, tomato, potato, banana, citrus and apple) are strongly affected by bacterial and fungal pathogens. They infect crops at the early stages of plant growth and remain until the handling of the food product in the market or industry. For instance, postharvest diseases have been estimated to be responsible for 30–50% of crop losses in developing countries (Fakruddin et al., 2015; Kumar and Kalita, 2017). Of the 7,100 classified bacterial species, approximately 150 cause serious losses in many different crops throughout the entire world, being more frequent in tropical and subtropical countries (Kannan et al., 2015). These microorganisms, known as phytopathogenic bacteria, provoke symptoms such as spots, blights, cankers and tissue rots (Martins et al., 2018). The genera most often associated with plant disease are Clavibacter, Xanthomonas, Erwinia, Pectobacterium, Pantoea, Agrobacterium, Pseudomonas, Ralstonia, Burkholderia, Acidovorax, Streptomyces, Xylella, Spiroplasma, and Phytoplasma (Kannan et al., 2015). On the other hand, most fungal pathogen species belong to the phyla Ascomycota and Basidiomycota. Among ascomycetes, fungal plant pathogens belong to various classes, such as Sordariomycetes (e.g., Fusarium sp.), Dothideomycetes (e.g., Phaeosphaeria sp., Alternaria sp.) or Leotiomycetes (e.g., Botrytis sp., Monilinia sp., Sclerotium sp.). Basidiomycetes are represented by two large and important plant pathogen groups: rusts (Pucciniomycetes) and smuts (Ustilaginomycetes) (Doehlemann et al., 2017). Fungi have a wide spectrum of lifestyles and high genetic plasticity, which allow them to colonize new hosts, develop resistance to fungicides and break the resistance trait created by breeding programs (Doehlemann et al., 2017). In addition, Oomycetes (a phylogenetic lineage of fungus-like eukaryotic microorganisms) include some of the most important plant pathogens, which can cause seedling blights, damping-off, root rots, downy mildews and various other diseases (Kamoun et al., 2015). Consequently, new biotechnological strategies are also needed to reduce their damage to crops.
In this scenario, microorganisms have vast biotechnological potential to meet global agricultural demands in a sustainable way (Singh et al., 2020). One of these demands is to control plant diseases that are responsible for the huge losses in crop and ornamental plant production (Green et al., 2020; Thakur et al., 2020). Thus, scientists have sought to understand, reproduce and enhance the protective effects that beneficial microbes might offer to plants in nature. For this purpose, microbial volatile organic compounds (VOCs), a blend of small signaling molecules produced by microorganisms, are important bioactive compounds against plant pathogens (Mitchell et al., 2010; Munjal et al., 2016; Raza et al., 2016a; Guevara-Avendaño et al., 2019). Each microorganism produces a wide and unique range of VOCs, also called volatilome, encompassing compounds of several chemical classes, and its production may also vary according to the microbial growth conditions.
In the last two decades, several studies have reported the ability of bacteria and fungi to inhibit the growth of phytopathogens through VOC emission. Part of the above-mentioned phytopathogens have already been successfully inhibited by microbial antagonists in vitro. Interestingly, some of the studies have demonstrated the antimicrobial activity in vivo as well, controlling diseases in grapes, wheat, peaches, and strawberries, for instance. Bacteria such as Bacillus, Pseudomonas and Serratia, and fungi, such as Aureobasidium and Candida, stood out as potential biological control agents. Importantly, several VOCs produced by these antagonists have been validated, including alcohols (e.g., 2-phenylethanol and 3-methyl-1-butanol), ketones (2-nonanone and 2-heptanone), pyrazines (2,5-dimethyl pyrazine and 2-methyl pyrazine) and sulfur-containing compounds (dimethyl disulfide and dimethyl trisulfide). Such diversity of molecules reflects on the very different molecular mechanisms involved in the pathogen growth inhibition discovered so far. For instance, it was showed that VOCs from Pseudomonas sp. caused DNA damaged to the sugarcane pathogen Thielaviopsis ethacetica (Freitas et al., 2022). Structural damage on hyphae and down-regulation in the expression of virulence factors in Ralstonia solanacearum are other mechanisms by which VOCs act (Raza et al., 2016a,b,c).
Thus, taking advantage on the effects of VOCs on phytopathogens, these bioactive compounds can be a safe biotechnological alternative to reduce the use of chemicals in agriculture and increase productivity. Interestingly, microbial VOC producers do not need to colonize the roots or the rhizosphere once VOCs can permeate through soil and then interact with plants and other microorganisms at a certain distance (Holopainen and Blande, 2012; Schulz-Bohm et al., 2018). However, important issues still rely on the production of a bioproduct that can effectively provide plant protection under open-field conditions, but also being economically viable and safe to human and environmental health (Korpi et al., 2009; Tilocca et al., 2020).
Herein, we approach the main aspects of microbial VOCs able to inhibit phytopathogens (Figure 1). First, it will describe the main characteristics about the volatilomes and its dynamicity. Sequentially, we highlight the biotechnological potential of these molecules in inhibiting some of the main economically important plant pathogens, as diverse studies have reported through in vitro and in vivo assays. Then, the mechanisms by which VOCs directly affect pathogens are addressed. Finally, similar to any innovation, there are several questions and challenges to overcome in the future, which are discussed in the last section.
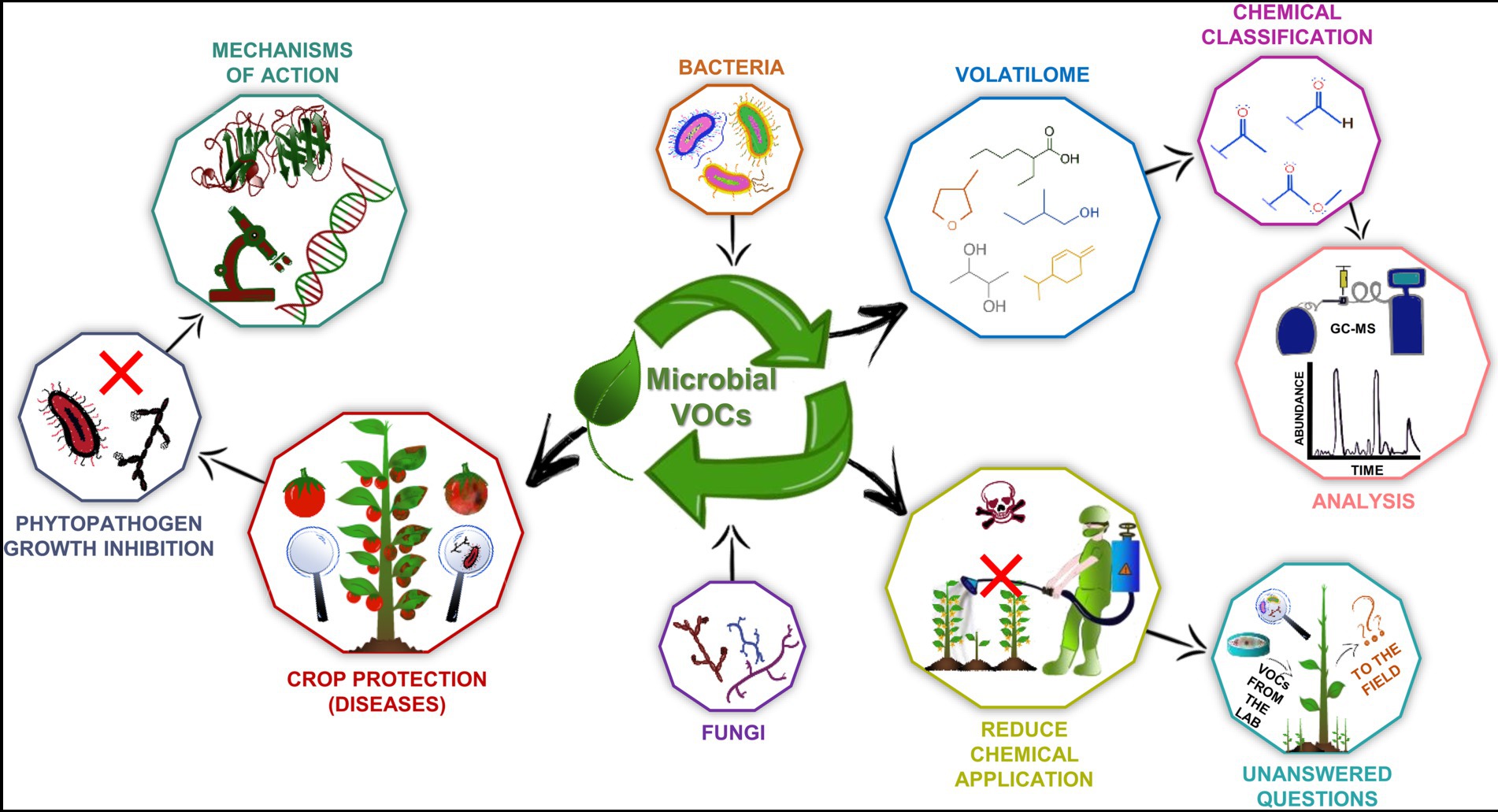
Figure 1. Biotechnological aspects of microbial volatile organic compounds (VOCs). They are produced by fungi and bacteria and belong to diverse chemical classes that can be identified by several techniques, such as gas chromatography coupled to mass spectrometry (GC–MS). These molecules have great biotechnology potential as plant growth promoters and phytopathogen inhibitors, and many studies have demonstrated such effects in vitro and in vivo. Therefore, it has become possible to develop bioproducts to be applied in the field and thereby reduce the use of agrochemicals. Nevertheless, some questions about the use of microbial VOCs as biotechnological products need to be answered. Moreover, although several beneficial microorganisms and bioactive VOCs have been described, the molecular mechanisms of action of these compounds remain poorly understood.
Microbial VOCs at a glance
Microbial VOCs are mostly carbon-based, small signaling molecules that evaporate easily at room temperature (Schulz and Dickschat, 2007; Korpi et al., 2009; Bitas et al., 2013; Kanchiswamy et al., 2015), and are able to move long distances through elements such as water, air and soil while in a gaseous state (Kanchiswamy et al., 2015). These compounds are mostly lipophilic, have low molecular weight (<300 g․mol−1), are derived from several biosynthetic pathways, and have high vapor pressures (0.01 KPa) at a temperature of 20°C (Bitas et al., 2013; Kanchiswamy et al., 2015). Generally, microbial VOCs are synthesized as side products during both primary and secondary metabolism (Schulz and Dickschat, 2007; Korpi et al., 2009).
Fungi and bacteria are capable of releasing a wide range of VOCs into the environment (Stahl and Parkin, 1996; Schulz and Dickschat, 2007). The predominant chemical classes of microbial VOCs are alkenes, alcohols, ketones, terpenes, benzenoids, pyrazines, acids, esters and aldehydes (Schulz and Dickschat, 2007; Korpi et al., 2009; Wenke et al., 2012; Peñuelas et al., 2014; Li et al., 2016), although the percentage of compounds in each class varies between bacteria and fungi (Peñuelas et al., 2014). For instance, bacteria emit a greater variety of terpenes, alkenes and ketones than fungi, whereas fungal VOCs emit a higher range of aldehydes, benzenoids and alcohols. Fungi also emit arsenics, chlorides, nitriles, thiofurans, alkynes, bromides and tellurium compounds, which are not commonly identified in bacterial volatilomes (Peñuelas et al., 2014).
It is well known that microbial volatilomes also vary according to the producing microorganism, showing some similarities among microbes belonging to the same genus (Choudoir et al., 2019; Guo et al., 2019b) or even the same species (Macías-Rubalcava et al., 2018; Guevara-Avendaño et al., 2019), but also some uniqueness by each one. Those conditions can be media composition (Fiddaman and Rossall, 1994; Bruce et al., 2004; Raza et al., 2015; Asari et al., 2016; Huang et al., 2018; Morita et al., 2019), media consistency (Dickschat et al., 2005; Qadri et al., 2015), period of incubation/growth stage (Weise et al., 2012; Giorgio et al., 2015; Sánchez-Fernández et al., 2016; Macías-Rubalcava et al., 2018; Misztal et al., 2018; Yang et al., 2019), humidity/water content (Jeleń, 2002; Misztal et al., 2018), temperature (Jeleń, 2002), oxygen and carbon dioxide levels (Zhang et al., 2013a), and interactions with other organisms (Sánchez-Fernández et al., 2016; Delgado et al., 2021).
Such dynamicity and diversity of microbial VOCs can influence complex environmental trophic interactions (Fiedler et al., 2001; Bitas et al., 2013). In this regard, microbial VOCs play an essential function as info-chemicals in microbial interactions by inducing or repressing the development and behavior of a wide range of organisms such as other microorganisms, plants, and animals (Bitas et al., 2013; Chaparro et al., 2014; Kanchiswamy et al., 2015). In plant-microbe interactions, microbial VOCs drives different responses in plant fitness and physiology, resulting in growth promotion, induced systemic resistance, tolerance against abiotic stress and microbiome shifting (Ryu et al., 2003; Vaishnav et al., 2015; Sharifi and Ryu, 2016; Dias et al., 2021; Kong et al., 2021). Regarding microbe-animal interactions, fungal and bacterial volatiles can also act as allelochemicals, triggering olfactory responses in insects and, consequently, influencing their behavior (Thakeow et al., 2008; Kandasamy et al., 2019; Goelen et al., 2020), which might also be applied to protect forest and crop production against these predators. Moreover, these molecules can enhance or inhibit the growth of other surrounding microorganisms. The interesting and efficient VOC-mediated antimicrobial activity against economically important phytopathogens will be explored in the upcoming sections.
Microbial VOCs inhibit a wide range of economically important phytopathogens
In the last two decades, studies have demonstrated that inhibition of phytopathogens can also be achieved through the release of VOCs by microorganisms. Thus, this section will provide an overview of fungal and bacterial species that were inhibited in vitro and in vivo by VOCs, with a focus on the main species that cause damage to agriculture and their antagonists. Table 1 brings those and more phytopathogens reported in literature and their respective antagonists. Furthermore, some specific VOCs were already validated as the molecules with the inhibitory activity. In order to validate them, the volatilomes produced by the microorganisms are first identified using different techniques (Morita et al., 2019; Guo et al., 2019b; Delgado et al., 2021), and then the compounds are purified using chromatography techniques or purchased in their synthetic form, to be tested in vitro against pathogens (Vinale et al., 2008; Munjal et al., 2016; Raza et al., 2016a, 2016b; Gotor-Vila et al., 2017). Due to some reasons that will be discussed later, it is not trivial to reproduce similar effects observed in the tests with the microbial antagonist by using synthetic compounds instead; however, several molecules with inhibitory activity have been validated thus far, which can be checked on Supplementary Table S1. Interestingly, some microbes and specific VOCs have already been validated in plants as well as in postharvest commodities (e.g., cherries, peaches, strawberries, tomatoes), which reinforces their biotechnological potential. We will also show that microbial VOCs can control numerous diseases affecting different parts of the plants (roots, stems, leaves, and fruits), thus improving their performance and productivity. Although some of these in vivo assays were performed with the microbial antagonist in physical contact with the phytopathogens (where other diffusible metabolites could be acting), the role of the VOCs in the growth inhibition was previously validated in vitro.
Crown gall pathogens
Agrobacterium tumefaciens is a soil-borne bacterium that easily infects wound sites in plant hosts and promotes neoplastic growth, causing crown gall tumors and, on a large scale, losses in crop production (Smith and Townsend, 1907). It is also one of the main model organisms in molecular biology, especially for its ability to mediate genetic transformation, which is very useful in fungal and plant biotechnology (Hwang et al., 2017). Another species of this genus is A. vitis, the causative agent of crown gall in grapevines (Vitis vinifera). It was demonstrated that diseases caused by both Agrobacterium species have the potential to be controlled by strains of the rhizobacteria Pseudomonas fluorescens and Serratia plymuthica (Dandurishvili et al., 2011). In vitro analysis indicated that the inhibitory effects against Agrobacterium were due to the VOCs emitted by the antagonist strains and not by antibiotics produced by them (Dandurishvili et al., 2011). Furthermore, analysis of the volatile profile of P. fluorescens and S. plymuthica revealed dimethyl disulfide (DMDS) as a compound emitted by all evaluated strains, and in vitro tests showed that this compound could efficiently suppress the growth of the Agrobacterium strains (Dandurishvili et al., 2011). The authors also demonstrated that the antagonists suppressed crown gall formation, resulting in a three- to eight-fold decrease in tumor mass, indicating that this molecule might be involved in the suppression of oncogenicity in tomato seedlings (Dandurishvili et al., 2011). Similar results were obtained with such plants infected with A. vitis. Accordingly, other studies reported the potential inhibitory activity of VOCs produced by different strains of Pseudomonas, as well as Serratia, against Agrobacterium sp. (Chernin et al., 2013; Popova et al., 2014; Plyuta et al., 2016). The observed effects on the pathogens include reduced biofilm formation, a decrease in the number of living cells composing the biofilm by almost 4-fold and a decreased mycelial growth. Those studies also confirmed DMDS as bioactive compound since it can cause the pathogens inhibition in almost 100%. The ketones 2-nonanone (Popova et al., 2014; Plyuta et al., 2016), 2-heptanone and 2-undecanone (Plyuta et al., 2016) were validated as well. Curiously, Ossowicki et al. (2017) did not observe inhibition of Agrobacterium sp. when it was in contact with VOCs produced by Pseudomonas donghuensis even that DMDS was identified in its volatilome. Sometimes, different results are reported in literature, regarding the inhibition of pathogens belonging to the same species. There are certain aspects that might influence it, and they will be further discussed.
Soft rot and bacterial speck pathogens
Of great agronomic importance, the genus Pectobacterium has a broad host range since it is isolated from various plant species, including important crops and ornamental plants (Ma et al., 2007). Complete inhibition of Pe. atrosepticum was achieved with VOCs emitted by the fungus Muscodor albus (Corcuff et al., 2011). Another important phytobacterium is Pseudomonas syringae, which has more than 60 pathovars, each of which is capable of infecting specific plant hosts and causing high economic impacts in several crops and trees (Xin et al., 2018), making it one of the most impactful phytopathogens worldwide (Mansfield et al., 2012). Ps. syrinage pv. tomato is the causal agent of bacterial speck of tomatoes, and it is a model species in molecular studies for understanding bacterial virulence mechanisms (Preston, 2000; Xin et al., 2018). A study showed that the strain Ps. syringae pv. tomato DC3000 could be inhibited by volatiles from two actinobacteria, Streptomyces sp. and Dietzia sp. (Choudoir et al., 2019). Although the volatilomes of these antagonists were determined, no functional validation was performed. Moreover, in vivo experiments showed that a blend of VOCs (2-methyl-propanol, 3-methyl-butanol, 4-heptanone, 3-octanone, m-methyl-anisole, m-cresol, 2-phenylethanol and cubenene) from Ampelomyces sp. and methyl benzoate from Cladosporium sp., reduced disease severity on Arabidopsis thaliana leaves infected with Ps. syringae pv. tomato DC3000 (Naznin et al., 2014). When individually tested, three VOCs from Ampelomyces sp., 3-octanone, m-cresol and 2-phenylethanol, and synthetic methacrylic acid and isobutyl acetate, both previously identified in Phoma sp. volatilome (Naznin et al., 2013), reduced disease severity in A. thaliana as well. Yet, the VOC hexadecane, produced by Paenibacillus polymyxa, conferred induced systemic resistance to A. thaliana against Ps. syringae (Park et al., 2013), which represents another mechanism by which VOCs can increase productivity without compromising the environment.
Bacterial wilt pathogens
The ‘Ralstonia solanacearum species complex’ (RSSC) comprises several strains belonging to a wide range of geographical origins, hosts, and pathogenic behaviors (Mansfield et al., 2012), and a recent classification proposed a division into three different species, R. solanacearum, R. pseudosolanacearum and R. syzygyi (Safni et al., 2014; Prior et al., 2016). They are capable of infecting more than 200 plant hosts, causing bacterial wilt and, consequently, leading to extensive economic losses in crops (e.g., tomato, eggplant, potato, tobacco, pepper, banana, peanut, and ginger) and ornamental plants, especially in some developing countries where the disease is endemic (Mansfield et al., 2012; Peeters et al., 2013; Tjou-Tam-Sin et al., 2017). It was shown that R. solanacearum was inhibited by up to 45% by B. amyloliquefaciens SQR-9 (Raza et al., 2016b), 44% by B. amyloliquefaciens T-5 (Raza et al., 2016c), and 51% by P. fluorescens (Raza et al., 2016a). Among the 22 and 25 VOCs identified in the volatilome of B. amyloliquefaciens SQR-9 and T-5, respectively, nine and 25 compounds were tested in vitro against R. solanacearum (Raza et al., 2016b, 2016c). Similarly, in both studies, the compounds had weak inhibitory effects when tested individually, reaching up to nearly 10% inhibition. However, different blends of VOCs achieved inhibition rates of 62–85% (Supplementary Table S1; Raza et al., 2016b, 2016c). Among the 13 VOCs identified in P. fluorescens, synthetic m-xylene and benzaldehyde caused complete inhibition of R. solanacearum, while partial inhibition was achieved with toluene, ethylbenzene, DMDS (50–55%), 2-decanol (85%), 2-tridecanol and 1-undecanol (<30%) (Raza et al., 2016a). Thus, VOCs from Bacillus and Pseudomonas species have been a biocontrol alternative against R. solanacearum.
Furthermore, Bacillus species seem to have great biocontrol potential (Munjal et al., 2016; Tahir et al., 2017a). The bacteria B. amyloliquefaciens and B. artrophaeus reduced the growth of R. solanacearum by 49 and 47%, respectively (Tahir et al., 2017a); their volatilomes comprised 13 and 10 VOCs, respectively: six were commonly identified in both species, while seven and four were distinct from each bacterium. Fifteen of these VOCs were tested against R. solanacearum, and the compounds benzaldehyde (60% inhibition), 1,2-benzisothiazol-3(2H)-one (51%) and 1,3-butadiene (30%) had the most effective results. The volatilome of the two bacteria and the synthetic compounds were also able to reduce wilt index on tobacco seedlings infected with the pathogen. More effectively, it was shown that B. megaterium completely inhibited R. solanacearum (Munjal et al., 2016). Among the several compounds detected in the B. megaterium volatilome, four pyrazine derivative compounds were tested in vitro against the pathogen. The synthetic 2-ethyl-3-methyl pyrazine completely inhibited R. solanacearum, while 2,5-dimethyl pyrazine, 2-ethyl pyrazine, and 2-methyl pyrazine caused 80, 55, and 32% inhibition, respectively (Munjal et al., 2016). Interestingly, these four pyrazine derivative compounds, also identified in the Pseudomonas putida volatilome, had inhibitory effects against R. pseudosolanacearum, another species of RSSC (Agisha et al., 2019). The same study showed that dimethyl trisulfide (DMTS) completely inhibited this pathogen.
Bacterial spot pathogens
The high-ranking positions of important bacterial phytopathogens are occupied by some Xanthomonas species (X. oryzae pv. oryzae, X. campestris and X. axonopodis pathovars), causing diseases on crops such as rice, cassava, cotton and cultivated brassicas (Mansfield et al., 2012). For such reason, their VOC-mediated biocontrol has been pursued. The growth of X. oryzae pv. oryzae was reduced by up to 38% by VOCs from Bacillus sp. strains (Xie et al., 2018). Among the 12 VOCs identified in the volatilome of the most effective strain, Bacillus sp. D13, two compounds, 3,5,5-trimethylhexanol (TMH) and decyl alcohol, were validated with 61 and 54% of inhibition, respectively. Inhibitory effects of TMH were also observed against X. oryzae pv. oryzicola. Regarding X. axonopodis pathovars, studies have demonstrated that they can be also inhibited via microbial VOCs (Mitchell et al., 2010; Munjal et al., 2016). Complete inhibition of X. axonopodis pv. citri, as well as 30 other fungi and bacteria, was achieved using Muscodor crispans as antagonist (Mitchell et al., 2010). Volatilome analysis of this fungus identified 17 compounds, but no validation test was performed. Likewise, X. axonopodis pv. punicae was completely inhibited by B. megaterium (Munjal et al., 2016). Although the volatilome of B. megaterium was determined (hydrocarbons, acids, alcohols, esters, pyrazines and sulfoxides), validation tests were not performed with this pathogen, only with Phytophthora capsici, R. soloanacearum and Magnaporthe oryzae (Supplementary Table S1).
Gray mold pathogens
In relation to phytopathogenic fungi, one of the most critical is the species Botrytis cinerea, the causal agent of gray mold, which causes serious problems in agriculture, such as the rotting of postharvest vegetables, fruits, and flowers, especially because it can easily develop resistance to fungicides (Williamson et al., 2007; Dean et al., 2012). In vitro and in vivo assays demonstrated the potential of VOCs produced by Ba. velezensis strains to inhibit Bo. cinerea and other pathogens (Table 1; Calvo et al., 2020). In in vitro tests, the antagonists were able to inhibit the growth of Bo. cinerea by up to 100%. Considering the VOCs identified on the volatilome of the Ba. velezensis strains, 11 synthetic compounds presented inhibitory effects against the pathogen, with diacetyl being the most effective bioactive compound at the lowest evaluated dose (Supplementary Table S1 for the complete list of compounds). Individually, the synthetic compounds diacetyl, benzaldehyde and isoamyl alcohol completely inhibited the fungal growth on the fruits (Calvo et al., 2020). The authors also showed that the whole volatilome of the antagonist was able to reduce disease incidence and severity caused by this pathogen on infected grapes by approximately 50%.
The biotechnological potential of Bacillus is reinforced against Bo. cinerea in other studies. For instance, Ba. amyloliquefaciens VOCs suppressed the mycelial growth of the pathogen by 87% (Gotor-Vila et al., 2017). In addition, three of the most abundant VOCs produced by this bacterium, thiophene, 1,3-pentadiene and acetoin (3-hydroxy-2-butanone), were validated as bioactive molecules, showing 83, 63 and 47% inhibition of mycelial growth, respectively (Gotor-Vila et al., 2017). Several other studies also reported inhibition of Bo. cinerea via VOCs emitted by Bacillus species: B. amyloliquefaciens (Asari et al., 2016; Chen et al., 2019), B. atrophaeus (Zhang et al., 2013b), B. licheniformis (Chen et al., 2019), B. pumillus (Liu et al., 2008), B. subtilis (Liu et al., 2008; Gao et al., 2018; Chen et al., 2019) and B. velezensis (Jiang et al., 2018; Cheffi et al., 2019; Chen et al., 2019; Myo et al., 2019).
Microorganisms belonging to other taxa also presented inhibitory activity against this pathogen. A recent study showed that the bacterium Gluconobacter cerinus and the yeast Hanseniaspora osmophila emitted VOCs that were able to inhibit the growth of Bo. cinerea by 32 and 39%, respectively (Delgado et al., 2021). Interestingly, a bioproduct composed of those two microorganisms was also tested (Besoain Canales et al., 2017), and it was able to inhibit the pathogen by 86% via VOCs, showing a synergic effect of both antagonists (Delgado et al., 2021). This bioproduct was also tested on grape cultivars infected with this pathogen, showing a higher inhibition rate (up to 98%). In addition, both biocontrol agents and the bioproduct were able to inhibit other three phytopathogens in vitro and in vivo (Table 1). Mostly, the bioproduct had similar or even better results than the antagonists individually tested. Therefore, it demonstrates the biotechnological potential of developing biofungicides based on combined VOC-emitter microorganisms. Furthermore, Parafati et al. (2017) showed that four yeasts, Wickerhamomyces anomalus, Metschnikowia pulcherrima, Aureobasidium pullulans and Saccharomyces cerevisiae, strongly reduced conidia germination and mycelial growth of B. cinerea. Those antagonists were also able to reduce at least two out of three disease parameters (disease incidence, disease severity and lesion diameter) in strawberries and grapes infected with the pathogen, especially W. anomalus, which reduced all parameters by 100%. These yeasts also controlled blue mold decay caused by Penicillium species on the same fruit. It is interesting to mention that, in addition to showing the potential use of microbial VOCs to control postharvest diseases, this study demonstrates that the immobilization of microbial cells in a polymeric matrix can be a more suitable and efficient method for biological control in postharvest management.
In this regard, the literature presents more microbial antagonists with such potential in vitro and, sometimes, in vivo. Regarding bacteria, Hanseniaspora uvarum (Qin et al., 2017), Paenibacillus polymyxa (Liu et al., 2008), Pseudomonas stutzeri (Rojas-Solís et al., 2018), Stenotrophomonas maltophilia (Rojas-Solís et al., 2018), Streptomyces mycarofaciens (Boukaew et al., 2017), Streptomyces platensis (Wan et al., 2008) and Streptomyces philanti (Boukaew et al., 2017) were reported as potential antagonists. As for fungi, especially yeasts, the studies show inhibitory activity induced by VOCs from Aureobasidium sp. (Di Francesco et al., 2015; Parafati et al., 2015; Chen et al., 2018; Yalage Don et al., 2020), Candida sp. (Arrarte et al., 2017; Chen et al., 2018; Mewa-Ngongang et al., 2019), Pichia sp. (Chen et al., 2018; Mewa-Ngongang et al., 2019), Saccharomyces sp. (Parafati et al., 2015; Chen et al., 2018), Monilliela sp. (Chen et al., 2018), M. pulcherrima, Wickerhamomyces cerevisiae (Parafati et al., 2015) and Muscodor crispans (Mitchell et al., 2010; Table 1). Compounds identified in the volatilome of some of the above mentioned microorganisms, such as 1-octen-3-ol (Zhao et al., 2011), 2-phenylethanol (Di Francesco et al., 2015; Guo et al., 2019a) and DMDS (Rojas-Solís et al., 2018), were validated in vitro against the pathogen as well (Supplementary Table S1 for the complete list).
Anthracnose pathogens
Colletotrichum species encompassing critical fungi that causes anthracnose in important fruits, such as mango, avocado, banana and citrus, and in common bean as well (Perfect et al., 1999; Peres et al., 2005; Cannon et al., 2012). In this scenario, the yeast Debaryomyces nepalensis has been studied as a sustainable alternative to inhibit C. gloeosporioides, being capable of reducing the mycelial growth by 40%, possibly due to the compound 2-phenylethanol (Zhou et al., 2018). The pathogen was also inhibited by VOCs from Pseudomonas putida (Sheoran et al., 2015) and, later, some compounds produced by the antagonist were validated, such as DMTS (complete inhibition) and the pyrazine derivative compounds 2,5-dimethyl pyrazine, 2-methyl pyrazine, 2-ethyl-5-methyl pyrazine and 2-ethyl-3,6-dimethyl pyrazine (partial inhibition; Agisha et al., 2019). Furthermore, inhibition of C. gloeosporioides was achieved via VOCs from Aureobasidium pullulans, Galactomyces candidum (Chen et al., 2018), Bacillus megaterium (Munjal et al., 2016), B. mycoides (Guevara-Avendaño et al., 2019), Ba. velezensis (Cheffi et al., 2019; Guevara-Avendaño et al., 2019), Burkholderia tropica (Tenorio-Salgado et al., 2013), Ba. subtilis, as well as by four compounds (benzothiazole, anisole, 3-methylbutanal, and 2,4-di-tert-butylthiophenol) identified in the Ba. subtilis volatilome (Gao et al., 2018) and Streptomyces sp., as well as methyl anthranilate (Gómez et al., 2021; Tables 1; Supplementary Table S1). Interestingly, Bu. tropica controlled anthracnose caused by this pathogen on maize plants by almost 80% (Martins et al., 2019).
Moreover, VOCs emitted by Lysinibacillus sp. were capable of totally inhibiting the mycelial growth of C. acutatum (Che et al., 2017). Validation tests of three VOCs produced by the antagonist showed that 2-ethyl-1-hexanol and benzaldehyde completely inhibited fungal growth, while 2-nonanone presented 61% of inhibition. Furthermore, A. pullulans (Di Francesco et al., 2015), Candida pyralidae and Pichia kluyveri (Mewa-Ngongang et al., 2019) were also reported as antagonists of C. acutatum. As for C. lindemuthianum, it was reported that this pathogen could be inhibited in vitro by Ba. velezensis (Gao et al., 2017) and both in vitro and in vivo (infected beans) by B. amyloliquefaciens (Martins et al., 2019). Another species of the genus Colletotrichum (C. fragariae) was efficiently inhibited by VOCs. Analysis of the volatilome of Irpex lacteus detected two VOCs (Koitabashi et al., 2002), lately identified as 5-pentyl-2-furaldehyde and 5-(4-pentenyl)-2-furaldehyde, which completely inhibited C. fragariae, in vitro (Koitabashi et al., 2004).
Fusarium wilt complex
Species of the genus Fusarium are soil-borne fungi with a worldwide distribution, affecting almost all existing crops (Bullerman, 2003). Thus, biocontrol alternatives against Fusarium species have been also widely pursued. For instance, a study reported that several soil bacterial strains could strongly or partially inhibit the growth of F. oxysporum, F. solani and F. culmorum (Garbeva et al., 2014). The antagonists included bacteria of 16 different genera (e.g., Burkholderia, Collimonas, Pseudomonas, Serratia and Stenotrophomonas), and they presented inhibitory effects against at least one of the Fusarium species (Table 1). Moreover, four Hypoxylum anthochroum strains were able to inhibit the growth of F. oxysporum in a range of 60 to 80% (Macías-Rubalcava et al., 2018). In total, 36 volatiles were identified in their volatilome, mainly sesquiterpenes (16) and monoterpenes (11). Eucalyptol and 3-methyl-1-butanol were the most abundant in all treatments and, interestingly, they were previously validated as potential biopesticides against F. oxysporum (Medina-Romero et al., 2017).
Yet, six Bacillus isolates and one Pseudomonas sp. partially inhibited F. solani (Guevara-Avendaño et al., 2019). Among the VOCs of different chemical classes identified in the volatilome of these strains, six compounds were evaluated in vitro (2,3,5-trimethylpyrazine, 2-nonanone, 2-decanone, 2-dodecanone, DMDS and DMTS), and all of them completely inhibited F. solani growth, with the exception of 2-dodecanone (38%). Other studies have reported VOC-mediated inhibition in vitro of the above-mentioned Fusarium species, as well as F. culmorum, F. moniliforme, F. flocciferum and F. graminearum. They include efficient antagonists such as Bacillus sp. (Liu et al., 2008; Li et al., 2015; Gao et al., 2017; Morita et al., 2019), Diaporthe phaseolorum (Qadri et al., 2015), Lysinibacillus sp. (Che et al., 2017), M. crispans (Mitchell et al., 2010), Nodulisporium sp. (Sánchez-Fernández et al., 2016), P. donghuensis (Ossowicki et al., 2017), and Trichoderma sp. (Chen et al., 2016; Rajani et al., 2021; complete list in Table 1). The synthetic compounds validated in some of these studies are presented in Supplementary Table S1.
Interestingly, in vivo inhibition of Fusarium species was also achieved. In cherry tomatoes, four H. anthochroum strains inhibited the growth of F. oxysporum by over 50%, varying the inhibition percentage according to the strain and the incubation period (Macías-Rubalcava et al., 2018). Furthermore, six synthetic VOCs (phenylethyl alcohol, 2-methyl-1-butanol, 3-methyl-1-butanol, eucalyptol, ocimene and terpinolene) identified on the H. anthochroum volatilome inhibited the growth of F. oxysporum on cherry tomatoes in a dose-dependent manner (Medina-Romero et al., 2017). Individually, the highest doses of 2-methyl-1-butanol, 3-methyl-1-butanol and ocimene completely inhibited the pathogens. Mixtures of the six compounds or of the four alcohols also presented complete inhibition. On tomato leaves infected with F. oxysporum, it was observed that the disease symptoms were reduced by spraying B. velezensis over them, even as early as the first sampling time (Myo et al., 2019). The plants treated with the antagonist not only suppressed pathogen growth but also increased shoot length and vigor index. Under greenhouse conditions, B. velezensis completely controlled F. oxysporum growth after up to 14 days. Other studies showed the biocontrol F. graminearum and F. oxysporum on wheat leaves (Koitabashi et al., 2002), F. culmorum and F. oxysporum on maize (Tenorio-Salgado et al., 2013) and F. oxysporum on watermelon seedlings (Faheem et al., 2015).
Rice blast pathogens
Other important phytopathogens belong to the family Magnaporthaceae, which comprises approximately 100 fungal species, including plant pathogens that cause diseases to grasses and related species, such as rice, millet, maize and wheat (Ebbole, 2007; Illana et al., 2013). For example, Magnaporthe oryzae is present in all rice-growing areas, causing blast disease, the most devastating rice disease worldwide (Illana et al., 2013); for this reason, and because it has been a model in plant-pathogen interaction studies, this pathogen leads a top 10 ranking of fungal pathogens in molecular plant pathology (Dean et al., 2012). In this scenario, a potential bacterial antagonist is Bacillus megaterium, which caused complete inhibition of M. oryzae via VOCs (Munjal et al., 2016). Among the 20 VOCs identified, four were tested and validated as bioactive compounds: 2-ethyl-3-methyl pyrazine, 2-ethyl pyrazine and 2-methyl pyrazine completely inhibited the pathogen, and 2,5-dimethyl pyrazine inhibited it by up to 74%. It was also reported that VOCs emitted by Pseudomonas isolates could inhibit M. oryzae by up to almost 50%, and one of these isolates, Pseudomonas sp. EA105, could also inhibit appressoria formation (Spence et al., 2014). Curiously, pyrazines (2,5-dimethyl pyrazine, 2-methyl pyrazine, 2-ethyl-5methyl pyrazine and 2-ethyl-3,6-dimethyl pyrazine) were also identified in P. putida (Sheoran et al., 2015), and all of them presented inhibitory activity against M. oryzae (Agisha et al., 2019).
Other pathogens
Although we have discussed the VOC-mediated inhibition of important pathogens that have prominent positions within publications in this research area, there are a range of other microbial pathogens that were inhibited via microbial VOCs. For instance, the inhibition of food spoilage fungi characterized by aflatoxin production affects postharvest losses during grain storage (Fakruddin et al., 2015; Kumar and Kalita, 2017), such as Aspergillus flavus and A parasiticus (Jeong et al., 2018; Yang et al., 2019). The studies also explored Alternaria, Cochliobolus, Monilinia, Penicillium, Phytophthora, Rhizoctonia, Thielaviopsis and Sclerotinia species. Those and other phytopathogens, that we could not properly discuss in our review, are succinctly presented in Table 1, as well as their respective antagonists. However, the role of VOCs in the inhibition of several phytopathogens ranked as the most important bacteria and fungi (Dean et al., 2012; Mansfield et al., 2012), such as Xanthomonas campestris pathovars, Erwinia amylovira, Xylella fastidiosa, Dickeya sp., Puccinia sp., Blumeria graminis, Mycospharella graminicola, Ustilago maydis, and Melampsora lini, has not yet been explored, and they certainly deserve attention from the scientific community.
To finalize, as we explored in this section, there are a multitude of microorganisms with the potential to inhibit phytopathogens, and it is notable that some bacteria and yeasts have stood out as potential biocontrol agents, such as Bacillus (especially B. amyloliquefaciens, B. subtilis and B. velezensis), Pseudomonas (especially P. chlororaphis, P. fluorescens and P. putida), Serratia and Streptomyces species. The VOCs emitted by them display promising futures as efficient and ecological alternatives for controlling diseases in crops.
VOCs cause several molecular changes on phytopathogens
Although it is well known that microbial VOCs can inhibit the growth of phytopathogens, the mechanisms involved in such processes remain poorly understood. Recent studies suggest that VOCs affect phytopathogens by modulating the activity of specific enzymes plus changing motility and protein production, which subsequently influence growth, cell morphology and virulence factors (Figure 2). In this section, we will present what these studies have reported and discussed about the mechanisms by which VOCs cause inhibition of these microorganisms.
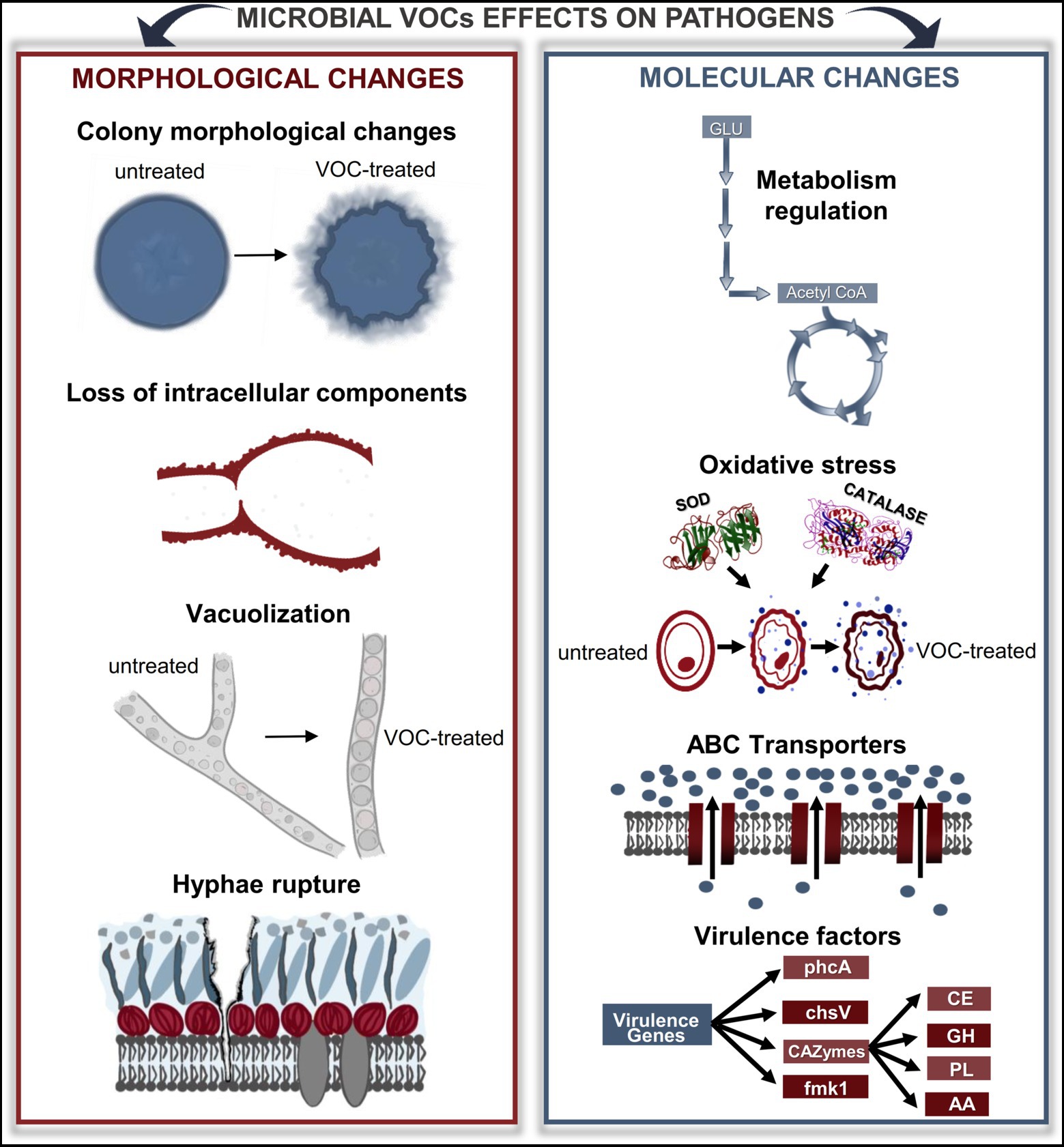
Figure 2. Microbial VOCs affect the morphology and physiology of phytopathogens. Through diverse approaches, it is possible to understand the changes that microbial VOCs cause in phytopathogens. Microscopy analyses revealed changes in the morphology of the colonies, loss of intracellular components, vacuolization, and cell rupture. In addition, omics-approaching studies have shown that many genes and proteins are up-and downregulated under the effects of VOCs, thus interfering with several important metabolic pathways, such as carbon metabolism and oxidative stress. GLU, glucose; SOD, superoxide dismutase; ABC, ATP-binding cassette; CAZymes, carbohydrate-activated enzymes; CE, carbohydrate esterases; GH, glycosyl hydrolases; PL, polysaccharide lyases; AA, auxiliary activities.
VOCs induce critical morphological changes
Microscopy has been the most common technique used to assess and observe the effects of VOCs on phytopathogens. Transmission electron microscopy (TEM), scanning electron microscopy (SEM) and optical microscopy are some types of techniques used to observe changes in cell and organelle morphology and consequently, improve the understanding of the mechanisms involved. Pathogenic bacteria, oomycetes and fungi are responsible for major losses in crops (Toth and Birch, 2005; Makovitzki et al., 2007; Dean et al., 2012; Mansfield et al., 2012; Drenth and Guest, 2016), and regarding the biocontrol of such microorganisms, it is important to bear in mind that bacteria and fungi have great differences in cell components, structure and morphology. While fungi can be multicellular eukaryotes, bacteria are single-celled prokaryotes without nuclei and other membrane-bound organelles and with asexual reproduction. Thus, the morphological changes caused by VOCs in bacteria are mostly associated with degradation of the outer membrane, disruption of the cytoplasmic membrane and loss of internal material that directly affects the bacterial ability to infect host plants (Helander et al., 1998; Bouhdid et al., 2009; Goel, 2017).
For instance, in the presence of VOCs from the rhizobacteria Bacillus subtilis, the colonies of Clavibacter michiganensis subsp. sepedonicus became internally distorted, and cells were damaged (Rajer et al., 2017). A wide range of abnormalities in the pathogen cells, such as misshapenness, disintegration of cells, formation of inclusions, movement of cytoplasmic content toward the ruptured cell walls or cytoplasmic membranes, and a lack of cytoplasmic content or fragmented cytoplasm were observed. Other studies showed similar changes in Ralstonia solanacearum morphology after exposing this pathogen to VOCs produced by antagonistic strains (Raza et al., 2016a,b,c; Tahir et al., 2017a). Microbial VOCs were capable of suppressing biofilm formation (Plyuta et al., 2016; Raza et al., 2016a,b,c), an important bacterial defense factor against external conditions that also plays a role in virulence (Danhorn and Fuqua, 2007). In addition, it has been reported that microbial VOCs have effects on virulence factors of phytopathogens, decreasing motility traits (swarming, swimming, and chemotaxis), production of antioxidant enzymes (e.g., superoxide dismutase and catalase) and exopolysaccharides, and the capability of colonizing tomato roots (Raza et al., 2016a,b,c).
Regarding phytopathogenic fungi, morphological changes were shown in their hyphal and mycelial structures. For instance, branched hyphae became straight and tiny when the saprotrophic fungus Mucor hiemalis was exposed to volatiles produced by Collimonas sp., a mycophagous soil bacterium (Garbeva et al., 2014). These changes were also observed on Fusarium sp. exposed to VOCs produced by Bacillus sp.; the pathogen developed shorter hyphal segments between septa and slightly distorted, ramified and curved hyphae at the edge of its colonies (Guevara-Avendaño et al., 2019). Flattened and perforated hyphae were observed in F. oxysporum, while shrinkage and perforation occurred in Penicillium digitatum, when both pathogens were treated with isooctanol, an alcohol identified in the Corrallococcus sp. (Ye et al., 2020). An increase in vacuolization processes (i.e., enlargement of vacuoles in mycelia, conidiophores or hyphae) has also been observed in some fungi inhibited by microbial VOCs or the respective synthetic compounds (Chaurasia et al., 2005; Tenorio-Salgado et al., 2013; Giorgio et al., 2015; Medina-Romero et al., 2017; Huang et al., 2018; Freitas et al., 2022). According to Macías-Rubalcava et al. (2018), these changes can compromise the respiration of microorganisms and affect cell membrane permeability, as was observed in the endophytic fungus Hypoxylon anthochroum (Macías-Rubalcava et al., 2018). In fact, depending on the severity of the vacuolization, hyphae rupture can also occur (Medina-Romero et al., 2017). Furthermore, large amounts of balloon-shaped cells, aggregation of cytoplasm and protoplasm, degradation of cell walls, cell breakage and leakage of intracellular substances on F. oxysporum were also observed due to exposure to volatiles emitted by Bacillus tropica (Tenorio-Salgado et al., 2013).
Plasmatic membranes of fungi can also be affected by microbial VOCs. The VOCs of Saccharomyces cerevisiae increased the lipid peroxidation of the pathogen Phyllosticta citricarpa and, consequently, decreased membrane fluidity, increased permeability to H+ and other ions and eventual cellular rupture, which directly affected the progress of the disease symptoms on plants. Such results corroborate the increase in membrane permeability of pathogenic spores of F. culmorum and Cochliobolus sativus as a result of the decrease in the efflux of K+ ions into the intracellular space caused by the compounds methyl propanoate and methyl prop-2-enoate (Kaddes et al., 2019). Moreover, volatiles emitted by B. subtilis and the pure compound benzothiazole affected the membrane ergosterol content of Monilinia fructicola and inhibited the activity of the enzymes pectinase and cellulase, which have an important role in cell wall and cell membrane integrity (Zhou et al., 2019). These recent findings elucidate that VOCs can directly alter membrane fluidity, resulting in leakage of intracellular contents and loss of cell viability, as well as triggering a cascade reaction to avoid oxidative stress.
Other effects on membranes were also observed when fungi were exposed to VOCs, such as strongly retracted plasma membrane in F. oxysporum, S. sclerotiorum (Wu et al., 2015) and Thielaviopsis ethacetica (Freitas et al., 2022), congregated and browned protoplasms in S. sclerotiorum (Liu et al., 2008), abnormal morphology of appressoria, an alteration that could affect the pathogen infection ability, in Peronophythora litchi (Xing et al., 2018) and M. fructicola (Zhou et al., 2019). Additionally, it was also shown that the intracellular components in M. fructicola were destroyed and an empty shell was formed, corroborating the idea that VOCs can destroy the barrier function of the cell wall and cell membrane (Zhou et al., 2019).
Furthermore, studies have also shown that VOCs mediate changes in the production and germination of conidia and spores. Conidia became swollen and thick-walled, and there was suppression of normal formation of sporangia and oogonia in Pythium afertile exposed to microbial VOCs (Chaurasia et al., 2005). The same study reported that the transverse and longitudinal septae completely disappeared in hyphae of Alternaria alternata, while conidiophores became vegetative and stunted in Cladosporium oxysporum (Chaurasia et al., 2005). Yet, other structural effects such as cell wall granulation, reduced number of mitochondria, destruction of organelles and internal cell darkening can be caused by VOCs, as it was observed in T. ethacetica (Freitas et al., 2022).
VOCs alter several metabolic pathways
More recently, many studies have incorporated modern molecular biology approaches to investigate the mechanisms related to the growth inhibition of phytopathogens. For instance, it was shown that treatment with the microbial VOC α-humulene inhibited the expression of two virulence genes, fmk1 and chsV, of the phytopathogen Fusarium oxysporum (Minerdi et al., 2009). The fmk1 gene encodes a mitogen-activated protein kinase that controls a number of key steps that regulate plant infection and fungal growth in living plant tissue (Roncero et al., 2003). The chsV gene encodes a protein homologous to class V chitin synthases that plays an important role in cell wall biosynthesis for pathogen adhesion (Liu et al., 2017). Curiously, in addition to the effects on Fusarium wilt pathogen growth and cell wall stability, α-humulene was also validated as a lettuce growth promoter biomolecule (Minerdi et al., 2011).
VOCs emitted by Bacillus amyloliquefaciens presented effects over the virulence factors of the phytopathogen Ralstonia solanacearum as well (Raza et al., 2016c). The VOCs produced were able to significantly reduce the expression of antioxidant activity-related genes katG and sobB and the transcriptional regulator PhcA by more than two times. PhcA is a cell density-dependent regulator that controls the expression of virulence factors such as extracellular polysaccharides (EPS), cell wall-degrading enzymes such as endoglucanases, and bacterial motility (Genin et al., 2005; Hikichi et al., 2017; Khokhani et al., 2017). Decreases in the expression of genes related to flagellum-dependent cell and twitching motility were also observed, supported by the motility trait assay results. On Xanthomonas oryzae pv. oryzae, after exposure to VOCs from a Bacillus strain, the transcriptional levels of the motA, motC (motility) and rpf (biofilm and virulence) genes were downregulated (Xie et al., 2018). Thus, VOCs regulate important genes related to survival, motility and pathogenicity.
To perform a deeper evaluation of VOC effects, omics studies have also been carried out. Proteomics analysis has shown that VOCs downregulate pathogenic proteins related not only to virulence (PhcA, proteins related to the type III and type IV secretion systems, EPS and chemotaxis-related) but also to the central metabolism of carbohydrates and amino acids, translation, protein folding and antioxidant activity (Raza et al., 2016a,b). These alterations not only negatively affected pathogen growth but also inhibited the virulence traits, root colonization, and metabolic activity of the pathogen, restricting its movements to colonize and infect plants (Tahir et al., 2017a).
In addition, other proteins seem to be important in the inhibition process. Proteins involved in antioxidant activity, such as thiol peroxidase, catalase, superoxide dismutase and polyphenol oxidase, were downregulated by more than two times, and their activity decreased by more than 60%. These enzymes not only neutralize reactive oxygen species (ROS) accumulated during the metabolism of the pathogen (Soto et al., 2006; Pfeilmeier et al., 2016) but also interact with the oxidative burst generated by their host plants during bacterial invasion and spreading (Huang and Allen, 2000; Afzal et al., 2014; Sahu et al., 2017). PhcA, which was downregulated in R. solanacearum (Raza et al., 2016a,b), also regulates endoglucanase; however, the activity of this enzyme was not affected in the same way. Controversially, another study showed that genes involved in redox reaction, as well as cell wall synthesis, were upregulated in F. oxysporum and Penicillium digitatum (Ye et al., 2020). Results also showed a burst of ROS in the pathogen cells. The authors suggested that the increase in the redox genes might be related to the attempt of neutralizing ROS.
Furthermore, it was shown that VOCs can change the synthesis of critical proteins involved in transport, genetic information processing, cellular processes, and metabolism (Fialho et al., 2016). Interestingly, several proteins related to carbohydrate and energy metabolism were downregulated, such as enolase, glyceraldehyde-3-phosphate dehydrogenase, pyruvate dehydrogenase and 6-phosphogluconate dehydrogenase. On the other hand, there was a noticeable increase in the expression of enzymes related to secondary metabolism, such as cyclase/dehydrase-like protein, ESC reductase and tetrahydroxynaphthalene reductase. These enzymes might be necessary to protect Phyllosticta citricarpa against VOCs, since they have a role in processes that stabilize the cell wall improve pathogenesis and increase stress resistance against radiation, oxidizing agents and antifungal compounds (Paolo et al., 2006; Atanasova et al., 2013).
More recently, it was demonstrated that the VOC S-methyl methanethiosulfonate has high protective potential in planta by affecting the oomycete Phytophthora infestans (Chinchilla et al., 2019). Proteomics analysis of the pathogen exposed to the pure compound revealed that 80% of the proteins were downregulated or undetectable. Massive downregulation of proteins involved in redox balance, secretion, posttranslational modification, protein turnover, signal transduction and transcription were also observed. In addition to demonstrating the anti-oomycete activity of S-methyl methanethiosulfonate through a multitarget mode of action, the study also revealed that exposure to closely related individual compounds from the same functional class (such as DMDS and DMTS) differently affects the inhibition and proteome of the pathogen.
An analysis comparing the effects of 2-phenyethanol (2-PE) and the volatilome of the yeast Candida intermedia on the global synthesis of proteins of the pathogen Aspergillus carbonarius revealed that both yeast volatilome and 2-PE treatments immediately inhibited mycelial growth and greatly reduced the production of the mycotoxin ochratoxin A (Tilocca et al., 2019). The proteomic investigation of both treatments revealed that 2-PE partly reproduced the metabolic changes promoted by C. intermedia, and the differences consisted of abundance levels of commonly identified proteins (Tilocca et al., 2019). Most global changes in protein expression patterns were identified in protein biosynthesis, proliferative activity, mitochondrial metabolism, and particularly in detoxification of toxic substances (Tilocca et al., 2019). Both treatments increased the levels of EuKaryotic Orthologous Groups (KOG) classes related to plasma membrane H+ transporting ATPase, E1-E2 ATPase, haloacid dehalogenase-like hydrolase, cation transporter/ATPase N-terminus and proteins involved in intra-and intercellular trafficking of vesicles. This shows that the single molecule and natural blend of VOCs target specific points of fungal metabolic pathways, resulting in responses that prevent fungal infections (Tilocca et al., 2019). Remarkably, both treatments stimulated stress response mechanisms in the fungal cells, with different origins for each sample. While 2-PE led to greater amounts of sphingosine phosphate lyase KOG class, which is linked to a cascade of catabolic reactions responding to “heat stress” in yeasts (Cowart and Obeid, 2007; Chen et al., 2013), the synthetic yeast mix volatilome treatment registered a higher expression of proteins with carbon-nitrogen hydrolase region KOG class, which has been matched with “resistance to chemicals” by yeasts (Bork and Koonin, 1994; Suda et al., 2003).
Remarkably, microbial VOCs can damage the DNA of phytopathogens. Ye et al. (2020) observed through fluorescence microscopy that F. oxysporum and Pe. digitatum presented phosphatidylserine externalization and DNA fragmentation after treated with the isooctanol. Recently, Freitas et al. (2022) showed that VOCs from Pseudomonas strains also caused DNA damage on the sugarcane pathogenic fungus Thielaviopsis ethacetica. Diverse genes involved in DNA damage response were upregulated, in comparison with phytopathogen cultivated in the absence of VOCs, and, interestingly, DNA cleavage and chromatin fragmentation were indicated via Fourier-transform infrared microspectroscopy, corroborating with the transcriptomic analysis. In addition, downregulation of several genes involved in metabolic pathways and morphological changes on mycelia contributed to the growth inhibition of this fungus. Therefore, DNA damage seems to be a crucial VOC-mediated mechanism of phytopathogens inhibition. These findings also demonstrate the importance of including omic approaches and other advanced technologies in order to investigate the molecular mechanisms involved in such processes.
Curiously, some mechanisms triggered by VOCs occur in the plant, helping in the disease control process. Volatiles emitted by B. subtilis and the pure compound benzothiazole not only inhibited Monilinia fructicola growth in vitro but also modulated the activity of cellulase, polygalacturonase, peroxidase, polyphenol oxidase, catalase and superoxide dismutase on infected peach fruits exposed to the volatiles (Zhou et al., 2019). These findings indicate that the damage caused by the phytopathogen on the fruit was mitigated by reducing the activity of the degrading enzymes and by increasing the activity of the antioxidant enzymes, which was also corroborated by the lower concentration of molecules that indicate oxidative stress, on treated fruits. As shown above, until now, few studies have focused on the molecular mechanisms and global analysis involved in pathogen inhibition via microbial VOCs (Fialho et al., 2016; Raza et al., 2016a,b,c; Chinchilla et al., 2019; Tilocca et al., 2019; Freitas et al., 2022). Thus, it is still too early to have a consensus on a comprehensive and well-established path by which microbial VOCs inhibit phytopathogens.
The path to a well established VOC-based bioproduct has a few challenges yet
As we perceived in the previous sections, VOCs have great biotechnological potential as a sustainable tool against phytopathogens, reducing the use of agrochemicals and, consequently, mitigating damage to the environment and human and animal health. However, many questions and uncertainties are still attributed to the control of phytopathogens using VOCs, from the search for an antagonist microorganism to the launch of a final product on the market. Some questions that need to be addressed are as follows: is the use of VOCs harmful to us? What about their impact on other living organisms and the environment? What is the minimum dose/concentration to be applied, that is also efficient? How long do they remain in the environment? Does the food absorb them? How long are they effective? Should they be applied pre, during or postharvest? These questions and others will be discussed hereafter.
In the last section, we mentioned that the molecular mechanisms by which VOCs act on phytopathogens remain poorly understood. Nevertheless, some issues about these mechanisms are frequently addressed, and one of them is whether they are biocide or biostatic. The inhibition caused by VOCs is biocidal when it results in the death of the phytopathogen or biostatic when it only ceases microorganism growth as long as the causal conditions are maintained. For instance, the phytopathogen Ralsotonia solanacearum was effectively inhibited by Bacillus amyloliquefaciens strains (Raza et al., 2016b) and Pseudomonas fluorescens (Raza et al., 2016a), but after removing the pathogen from the culture system, its growth rate was resumed, showing that both antagonists have a bacteriostatic effect. On the other hand, the phytopathogen Fusarium solani, after being inhibited by VOCs of B. velezensis, did not present mycelial growth when it was transferred to new plates of PDA medium (Cheffi et al., 2019). The damage caused to this pathogen was enough to cause a fungicidal effect. The reason that some VOCs only cease the growth of pathogens while others kill them is still unclear, but it shows that the development of an efficient bioproduct requires a careful analysis of such effects.
Another issue concerning the use of microorganisms or specific volatiles as a product is the difference of results observed with the same pathogen. We previously showed that sometimes, the results obtained with the synthetic volatiles on validation tests are not the same as those with the antagonist strain. For instance, the growth inhibition of Agrobacterium tumefaciens by VOCs from Serratia sp., mainly due to the compound DMDS, was observed by Dandurishvili et al. (2011), Chernin et al. (2013), Popova et al. (2014) and Plyuta et al. (2016), but not by Ossowicki et al. (2017). Thus, we need to expand our knowledge about which bioactive molecules act individually or in combination and what respective concentrations are necessary to cause inhibition. This can be a tough task, since in our review of the literature, we observed very distinct assays to identify microbial antagonists, several approaches to determine their volatilomes and to perform functional validation of each compound. In addition, microorganisms exhibit substantial genetic and metabolic plasticity, which can lead to different responses to those observed in previous tests or tests performed by different research groups.
One of the main questions about the formulation of a product and its application in the field concerns whether the microorganism will be able to produce the bioactive compounds and in a necessary concentration to inhibit the phytopathogens. Usually, in vitro tests are able to determine a minimum dose of compounds or microorganisms with a biocidal or a durable biostatic effect against the pathogen. Nonetheless, we have already illustrated that different cultivation conditions can influence the volatilome of a single microorganism, so in the soil, it would be no different. The physical and nutritional attributes of soil, cultivated crops, climate, human intervention, and rhizospheric microbial community change over time, directly affecting the production and composition of the volatilomes (Peñuelas et al., 2014; Kai et al., 2016; De Boer et al., 2019), as well as resulting in a dynamic and specific system. Accordingly, Bastos and Magan (2007) observed clear differences in the VOC profiles produced by the microbial community of three different soils (sandy loam, calcareous clay soil, and volcanic ash), varying water potential, temperature, and nutrient source. Given the diversity of soils worldwide, the ability of microorganisms to produce VOCs capable of inhibiting pathogens might become limited to certain specific conditions. However, a very recent study reported that a bioproduct has a high capacity to inhibit grape pathogens via VOCs in in vitro and in vivo assays (see further sections) (Besoain Canales et al., 2017; Delgado et al., 2021). It may be prepared in culture media ensuring the production of the molecules of interest (Besoain Canales et al., 2017). Besides, such results demonstrate the synergism of the two antagonists and shed light upon a path that combining different microorganisms can be an interesting alternative to formulate a highly efficient biocontrol product.
Another issue concerns the shelf life of a biological product, and some nanotechnology strategies have been suggested. For instance, the antagonist yeasts Wickerhamomyces anomalous and Aureobasidium pullulans were encapsulated in hydrogel spheres and applied to strawberry fruits (Fragaria ananassa) and tangerines (Citrus reticulata) (Parafati et al., 2017). Although the inhibitory effects were promising, the yeasts had a survival time of only 10 days. The minimum shelf life of a biocontrol formula should be approximately 8 months so it could be commercialized (Nakkeeran et al., 2006). It is important to mention that encapsulation requires proper evaluation of the process, since it can alter some physiological properties of immobilized cells and even decrease cell viability (Rathore et al., 2013). However, any formulation consists of an important developing stage that demands much knowledge, technique, time and many tests until a long-lasting output is obtained.
An alternative for a product is to produce it based on bioactive molecules without including microorganisms in the composition. First, it is necessary to know the bioactive compounds and their efficiency as either single molecules or blends to create a viable product. As we have already shown, some individual compounds perform a complete inhibition of the pathogen and, in other cases, it is the mixture of compounds that have a maximum inhibitory activity. However, it is important to mention that occasionally, not all compounds are commercially available, and usually, they have a high cost for just a tiny amount. Consequently, the ability to test many different concentrations and combinations of compounds is limited. However, when bioactive compounds are identified, it is possible to think about new delivery strategies. Recently, it was shown that lipid nanoparticles can be promising carriers for encapsulating VOCs due to their physiochemical properties and biological activity (Fincheira et al., 2020). In addition, it has already been shown that polyamide-based microcapsules with TiO2 can be successfully used to trigger the release of volatile organic compounds by UV-A irradiation (Marques et al., 2013). Recently, Mun and Townley (2020) reviewed the different types of nanoparticles to encapsulate plant VOCs, but we believe that the same can be true for microbial volatiles, which is a valuable alternative for using these compounds in the field.
Nevertheless, it is important to know if it is free of risks to human, animal, and environmental health. Even in small quantities, VOCs are extremely active molecules, and they rapidly spread into the atmosphere, thus having the possibility to trigger damage to nontarget organisms (Sharifi and Ryu, 2018). Although VOCs produced by Muscodor albus have high efficiency in controlling several fungi, one of its metabolites is toxic to human health, preventing the further use of this biocontrol agent (Braun et al., 2012; Romanazzi et al., 2012). Furthermore, the compound DMDS is a widely found and very controversial compound, since it has promoted growth of A. thaliana (Groenhagen et al., 2013) and inhibited some phytopathogens (Supplementary Table S1), but also inhibited the growth of Nicotiana attenuata plants (Meldau et al., 2013) and had toxic effects on nematodes and Drosophila melanogaster (Popova et al., 2014), as well as on mammals, such as rats, mice and humans (Korpi et al., 2009). Considering that VOCs can be noxious to humans, several in vitro and in vivo studies are necessary to obtain proper information about their safety and then to construct a robust VOCs database with this information (Korpi et al., 2009).
In this sense, it is also important to know whether VOCs are always benefic to plants, or if they can be harmful. In many cases, microbial VOCs promote changes on plant metabolism (e.g., modulation of biosynthesis and signaling pathways of phytohormones or production of plant VOCs that acts as attractor to natural enemies of the pathogens) resulting in a more effective defense response (Schausberger et al., 2012; Pineda et al., 2013; Sharifi and Ryu, 2016, 2018). Nevertheless, microbial VOCs can sometimes be harmful to plants. For instance, in a screening of 20 Trichoderma strains, although nine strains promoted Arabidopsis growth, VOCs from T. atroviride decreased the total fresh weight and the total chlorophyll content of plants and damaged the plant leaves (Lee et al., 2016). Another study that screened Trichoderma species for plant growth promoters found that plants co-cultivated with T. asperellum had a lower biomass of up to 74% (Nieto-Jacobo et al., 2017). Although Trichoderma species have biofungicidal potential, as we have already shown, not all strains or species are harmless to plants. From the same perspective, as we discussed just above, DMDS can promote or inhibit plant growth. Thus, further investigation is needed to better understand the beneficial effects of VOCs on plants, as well as their potential negative effects, since they can cause undesirable effects.
Although our focus is on the action of VOCs on phytopathogens, knowing how plants sense the molecules is important since VOCs also trigger some plant mechanisms and, in turn, can aid in the inhibition process. Therefore, how do plants sense microbial VOCs? Currently, the understanding of the perception of VOCs by plants is based on well-characterized studies of plant volatiles, which are compounds of diverse chemical classes emitted during biotic stresses and injuries, and they are responsible for plant–plant, plant–animal and plant-microbe interactions (Matsui, 2006; Maffei, 2010; Scala et al., 2013). Thus, based on studies about these volatiles and how plants sense them (Choh et al., 2004; Holopainen, 2004; Mirabella et al., 2015), we gain insights into microbial VOCs receptors in plants and their perception, but a proper investigation about them is still necessary to better understand their occurrence, their mode of action, and whether they can distinguish VOCs from beneficial and pathogenic microorganisms.
These and other questions that we did not address need to be answered to produce more sustainable biocontrol products on the market. From then on, we will reduce our dependence on agrochemicals by integrating microbial VOC-based formulas in crop management. The potential of volatile organic compounds is enormous, and with further studies, the bottlenecks that still hold back their wide usage in agriculture can be overcome.
Conclusion
By 2050, the global population is expected to reach over 10 billion, and this population increase will require doubling the level of food production. To ensure adequate global food for this growing population, we need to maximize crop production and minimize both the demand for financial resources and the impact on the environment. In this regard, the use of biotechnology with microbial products is now considered a valuable addition to sustainable agriculture. Bacteria and fungi can inhibit the growth of plant pathogens through VOCs, including those of greater scientific and economic importance. Here, we showed that Agrobacterium, Blumeria, Botrytis, Colletotrichum, Fusarium, Magnaporthe, Pectobacterium, Ralstonia and Xanthomonas species were successfully controlled in in vitro and in vivo assays. As antagonists, Bacillus (B. amyloliquefaciens, B. subtilis and B. velezensis), Pseudomonas (e.g., P. chlororaphis, P. fluorescens and P. putida), Serratia and Streptomyces species stand out, and those genera represent promise for the formulation of efficient biocontrol products, as well as several bioactive compounds that they are able to produce. However, despite this potential, their biological activities are not properly explored in the field because there are still numerous scientific questions to be addressed. In this sense, different biotechnology tools have allowed us to better understand the mechanisms by which microbial VOCs inhibit phytopathogens. We still have to learn more about these mechanisms, as well as many other aspects of biosafety, to develop a bioproduct. Despite these concerns, it is very clear that beneficial VOC-producing microorganisms have vast biotechnological potential in helping to enhance sustainable agriculture.
Author contributions
OA and JO conceived the idea of the manuscript. OA, NA, BD, CF, JO, C-MR, and LC: co-wrote the main text. NA, OA, and CF: designed the figures. OA designed the Supplementary Tables. OA, JO, and CR critically revised the manuscript. All authors approved the final draft.
Funding
This work was supported by the São Paulo Research Foundation (FAPESP; 2017/24395-5, 2019/08522-2, 2018/04184-2 and 2022/00474-1), Conselho Nacional de Desenvolvimento Científico e Tecnológico (CNPq; project 870370/1997-9), Coordenação de Aperfeiçoamento de Pessoal de Nível Superior (Capes; 88887.514087/2020-00 and 8887.144410/2017-00), and by the National Research Foundation of Korea (NRF) grant funded by the Korea government (MSIP) under Grant numbers 2021-212 and 2021-128, KRIBB Initiate Program.
Conflict of interest
The authors declare that the research was conducted in the absence of any commercial or financial relationships that could be construed as a potential conflict of interest.
Publisher’s note
All claims expressed in this article are solely those of the authors and do not necessarily represent those of their affiliated organizations, or those of the publisher, the editors and the reviewers. Any product that may be evaluated in this article, or claim that may be made by its manufacturer, is not guaranteed or endorsed by the publisher.
Supplementary material
The Supplementary material for this article can be found online at: https://www.frontiersin.org/articles/10.3389/fmicb.2022.951130/full#supplementary-material
References
Afzal, F., Khurshid, R., Ashraf, M., and Gul Kazi, A. (2014). “Reactive oxygen species and antioxidants in response to pathogens and wounding,” in Oxidative Damage to Plants: Antioxidant Networks and Signaling. ed. Paryaiz Ahmad (Elsevier Inc.), 397–424.
Agisha, V. N., Kumar, A., Eapen, S. J., Sheoran, N., and Suseelabhai, R. (2019). Broad-spectrum antimicrobial activity of volatile organic compounds from endophytic Pseudomonas putida BP25 against diverse plant pathogens. Biocontrol Sci. Tech. 29, 1069–1089. doi: 10.1080/09583157.2019.1657067
Aiello, D., Restuccia, C., Stefani, E., Vitale, A., and Cirvilleri, G. (2019). Postharvest biocontrol ability of Pseudomonas synxantha against Monilinia fructicola and Monilinia fructigena on stone fruit. Postharvest Biol. Technol. 149, 83–89. doi: 10.1016/j.postharvbio.2018.11.020
Arrarte, E., Garmendia, G., Rossini, C., Wisniewski, M., and Vero, S. (2017). Volatile organic compounds produced by Antarctic strains of Candida sake play a role in the control of postharvest pathogens of apples. Biol. Control 109, 14–20. doi: 10.1016/j.biocontrol.2017.03.002
Asari, S., Matzén, S., Petersen, M. A., Bejai, S., and Meijer, J. (2016). Multiple effects of Bacillus amyloliquefaciens volatile compounds: plant growth promotion and growth inhibition of phytopathogens. FEMS Microbiol. Ecol. 92, 1–11. doi: 10.1093/femsec/fiw070
Atanasova, L., Knox, B. P., Kubicek, C. P., Druzhinina, I. S., and Baker, S. E. (2013). The polyketide synthase gene pks4 of Trichoderma reesei provides pigmentation and stress resistance. Eukaryot. Cell 12, 1499–1508. doi: 10.1128/EC.00103-13
Bastos, A. C., and Magan, N. (2007). Soil volatile fingerprints: use for discrimination between soil types under different environmental conditions. Sensors Actuators B Chem. 125, 556–562. doi: 10.1016/j.snb.2007.03.001
Besoain Canales, X. A., Cadiz Morales, F. F., and Varas, E. S. (2017). WO2017088081A1- Gluconobacter Cerinus Plus Hanseniaspora osmophila for Controlling Fungal Infections in Plants and Fruits. 1–12. Available at: https://patents.google.com/patent/WO2017088081A1/en (Accessed May 13, 2022).
Bitas, V., Kim, H. S., Bennett, J. W., and Kang, S. (2013). Sniffing on microbes: diverse roles of microbial volatile organic compounds in plant health. Mol. Plant-Microbe Interact. 26, 835–843. doi: 10.1094/MPMI-10-12-0249-CR
Bork, P., and Koonin, E. V. (1994). A new family of carbon-nitrogen hydrolases. Protein Sci. 3, 1344–1346. doi: 10.1002/pro.5560030821
Bouhdid, S., Abrini, J., Zhiri, A., Espuny, M. J., and Manresa, A. (2009). Investigation of functional and morphological changes in Pseudomonas aeruginosa and Staphylococcus aureus cells induced by Origanum compactum essential oil. J. Appl. Microbiol. 106, 1558–1568. doi: 10.1111/j.1365-2672.2008.04124.x
Boukaew, S., Prasertsan, P., Troulet, C., and Bardin, M. (2017). Biological control of tomato gray mold caused by Botrytis cinerea by using Streptomyces spp. BioControl 62, 793–803. doi: 10.1007/s10526-017-9825-9
Braun, G., Vailati, M., Prange, R., and Bevis, E. (2012). Muscodor albus volatiles control toxigenic fungi under controlled atmosphere (CA) storage conditions. Int. J. Mol. Sci. 13, 15848–15858. doi: 10.3390/ijms131215848
Bruce, A., Verrall, S., Hackett, C. A., and Wheatley, R. E. (2004). Identification of volatile organic compounds (VOCs) from bacteria and yeast causing growth inhibition of sapstain fungi. Holzforschung 58, 193–198. doi: 10.1515/HF.2004.029
Bullerman, L. B. (2003). SPOILAGE|fungi in food – an overview. Encycl. Food Sci. Nutr., 5511–5522. doi: 10.1016/B0-12-227055-X/01129-9
Calvo, H., Mendiara, I., Arias, E., Gracia, A. P., Blanco, D., and Venturini, M. E. (2020). Antifungal activity of the volatile organic compounds produced by bacillus velezensis strains against postharvest fungal pathogens. Postharvest Biol. Technol. 166:111208. doi: 10.1016/j.postharvbio.2020.111208
Cannon, P. F., Damm, U., Johnston, P. R., and Weir, B. S. (2012). Colletotrichum - current status and future directions. Stud. Mycol. 73, 181–213. doi: 10.3114/sim0014
Chaparro, J. M., Badri, D. V., and Vivanco, J. M. (2014). Rhizosphere microbiome assemblage is affected by plant development. ISME J. 8, 790–803. doi: 10.1038/ismej.2013.196
Chaurasia, B., Pandey, A., Palni, L. M. S., Trivedi, P., Kumar, B., and Colvin, N. (2005). Diffusible and volatile compounds produced by an antagonistic Bacillus subtilis strain cause structural deformations in pathogenic fungi in vitro. Microbiol. Res. 160, 75–81. doi: 10.1016/j.micres.2004.09.013
Che, J., Liu, B., Liu, G., Chen, Q., and Lan, J. (2017). Volatile organic compounds produced by Lysinibacillus sp. FJAT-4748 possess antifungal activity against Colletotrichum acutatum. Biocontrol Sci. Tech. 27, 1349–1362. doi: 10.1080/09583157.2017.1397600
Cheffi, M., Bouket, A. C., Alenezi, F. N., Luptakova, L., Belka, M., Vallat, A., et al. (2019). Olea europaea l. root endophyte bacillus velezensis oee1 counteracts oomycete and fungal harmful pathogens and harbours a large repertoire of secreted and volatile metabolites and beneficial functional genes. Microorganisms 7:314. doi: 10.3390/microorganisms7090314
Chen, P.-H., Chen, R.-Y., and Chou, J.-Y. (2018). Screening and evaluation of yeast antagonists for biological control of Botrytis cinerea on strawberry fruits. Mycobiology 46, 33–46. doi: 10.1080/12298093.2018.1454013
Chen, P. W., Fonseca, L. L., Hannun, Y. A., and Voit, E. O. (2013). Coordination of rapid sphingolipid responses to heat stress in yeast. PLoS Comput. Biol. 9:e1003078. doi: 10.1371/journal.pcbi.1003078
Chen, J. L., Sun, S. Z., Miao, C. P., Wu, K., Chen, Y. W., Xu, L. H., et al. (2016). Endophytic Trichoderma gamsii YIM PH30019: a promising biocontrol agent with hyperosmolar, mycoparasitism, and antagonistic activities of induced volatile organic compounds on root-rot pathogenic fungi of Panax notoginseng. J. Ginseng Res. 40, 315–324. doi: 10.1016/j.jgr.2015.09.006
Chen, X., Wang, Y., Gao, Y., Gao, T., and Zhang, D. (2019). Inhibitory abilities of Bacillus isolates and their culture filtrates against the gray mold caused by Botrytis cinerea on postharvest fruit. Plant Pathol. J. 35, 425–436. doi: 10.5423/PPJ.OA.03.2019.0064
Chernin, L., Toklikishvili, N., Dandurishvili, N., Tediashvili, M., and Vainstein, A. (2013). Suppression of crown gall disease by rhizosphere bacteria and Agrobacterium-specific bacteriophages. Mol. Microb. Ecol. Rhizosph. 2, 607–613. doi: 10.1002/9781118297674.CH57
Chinchilla, D., Bruisson, S., Meyer, S., Zühlke, D., Hirschfeld, C., Joller, C., et al. (2019). A sulfur-containing volatile emitted by potato-associated bacteria confers protection against late blight through direct anti-oomycete activity. Sci. Rep. 9:18778. doi: 10.1038/s41598-019-55218-3
Choh, Y., Shimoda, T., Ozawa, R., Dicke, M., and Takabayashi, J. (2004). Exposure of lima bean leaves to volatiles from herbivore-induced conspecific plants results in emission of carnivore attractants: active or passive process? J. Chem. Ecol. 30, 1305–1317. doi: 10.1023/B:JOEC.0000037741.13402.19
Choudoir, M., Rossabi, S., Gebert, M., Helmig, D., and Fierer, N. (2019). A phylogenetic and functional perspective on volatile organic compound production by Actinobacteria. mSystems :4. doi: 10.1128/msystems.00295-18
Corcuff, R., Mercier, J., Tweddell, R., and Arul, J. (2011). Effect of water activity on the production of volatile organic compounds by Muscodor albus and their effect on three pathogens in stored potato. Fungal Biol. 115, 220–227. doi: 10.1016/j.funbio.2010.12.005
Cordovez, V., Carrion, V. J., Etalo, D. W., Mumm, R., Zhu, H., van Wezel, G. P., et al. (2015). Diversity and functions of volatile organic compounds produced by Streptomyces from a disease-suppressive soil. Front. Microbiol. 6:1081. doi: 10.3389/fmicb.2015.01081
Cowart, L. A., and Obeid, L. M. (2007). Yeast sphingolipids: recent developments in understanding biosynthesis, regulation, and function. Biochim. Biophys. Acta-Mol. Cell Biol. Lipids 1771, 421–431. doi: 10.1016/j.bbalip.2006.08.005
D’Alessandro, M., Erb, M., Ton, J., Brandenburg, A., Karlen, D., Zopfi, J., et al. (2014). Volatiles produced by soil-borne endophytic bacteria increase plant pathogen resistance and affect tritrophic interactions. Plant Cell Environ. 37, 813–826. doi: 10.1111/pce.12220
Dandurishvili, N., Toklikishvili, N., Ovadis, M., Eliashvili, P., Giorgobiani, N., Keshelava, R., et al. (2011). Broad-range antagonistic rhizobacteria Pseudomonas fluorescens and Serratia plymuthica suppress Agrobacterium crown gall tumours on tomato plants. J. Appl. Microbiol. 110, 341–352. doi: 10.1111/j.1365-2672.2010.04891.x
De Boer, W., Li, X., Meisner, A., and Garbeva, P. (2019). Pathogen suppression by microbial volatile organic compounds in soils. FEMS Microbiol. Ecol. 95, 1–10. doi: 10.1093/femsec/fiz105
Dean, R., Van Kan, J. A. L., Pretorius, Z. A., Hammond-Kosack, K. E., Di Pietro, A., Spanu, P. D., et al. (2012). The top 10 fungal pathogens in molecular plant pathology. Mol. Plant Pathol. 13, 414–430. doi: 10.1111/j.1364-3703.2011.00783.x
Delgado, N., Olivera, M., Cádiz, F., Bravo, G., Montenegro, I., Madrid, A., et al. (2021). Volatile organic compounds (VOCs) produced by Gluconobacter cerinus and Hanseniaspora osmophila displaying control effect against table grape-rot pathogens. Antibiot. 10, 663–681. doi: 10.3390/antibiotics10060663
Di Francesco, A., Di Foggia, M., and Baraldi, E. (2020). Aureobasidium pullulans volatile organic compounds as alternative postharvest method to control brown rot of stone fruits. Food Microbiol. 87:103395. doi: 10.1016/j.fm.2019.103395
Di Francesco, A., Milella, F., Mari, M., and Roberti, R. (2017). A preliminary investigation into Aureobasidium pullulans as a potential biocontrol agent against Phytophthora infestans of tomato. Biol. Control 114, 144–149. doi: 10.1016/j.biocontrol.2017.08.010
Di Francesco, A., Ugolini, L., Lazzeri, L., and Mari, M. (2015). Production of volatile organic compounds by Aureobasidium pullulans as a potential mechanism of action against postharvest fruit pathogens. Biol. Control 81, 8–14. doi: 10.1016/j.biocontrol.2014.10.004
Dias, B. H. S., Jung, S.-H., de Oliveira, J. V. C., and Ryu, C.-M. (2021). C4 bacterial volatiles improve plant health. PathoGenetics 10:682. doi: 10.3390/pathogens10060682
Dickschat, J. S., Martens, T., Brinkhoff, T., Simon, M., and Schulz, S. (2005). Volatiles released by a Streptomyces species isolated from the North Sea. Chem. Biodivers. 2, 837–865. doi: 10.1002/cbdv.200590062
Doehlemann, G., Ökmen, B., Zhu, W., and Sharon, A. (2017). Plant pathogenic fungi. Microbiol. Spectr. 5, 1–23. doi: 10.1128/microbiolspec.FUNK-0023-2016
Drenth, A., and Guest, D. I. (2016). Fungal and oomycete diseases of tropical tree fruit crops. Annu. Rev. Phytopathol. 54, 373–395. doi: 10.1146/ANNUREV-PHYTO-080615-095944
Ebbole, D. J. (2007). Magnaporthe as a model for understanding host-pathogen interactions. Annu. Rev. Phytopathol. 45, 437–456. doi: 10.1146/annurev.phyto.45.062806.094346
Elshafie, H. S., Camele, I., Racioppi, R., Scrano, L., Iacobellis, N. S., and Bufo, S. A. (2012). In vitro antifungal activity of Burkholderia gladioli pv. Agaricicola against some phytopathogenic fungi. Int. J. Mol. Sci. 13, 16291–16302. doi: 10.3390/ijms131216291
Faheem, M., Raza, W., Zhong, W., Nan, Z., Shen, Q., and Xu, Y. (2015). Evaluation of the biocontrol potential of Streptomyces goshikiensis YCXU against Fusarium oxysporum f. sp. niveum. Biol. Control 81, 101–110. doi: 10.1016/j.biocontrol.2014.11.012
Fakruddin, M., Chowdhury, A., Hossain, M. N., and Ahmed, M. M. (2015). Characterization of aflatoxin producing Aspergillus flavus from food and feed samples. Springerplus 4, 1–6. doi: 10.1186/s40064-015-0947-1
Farbo, M. G., Urgeghe, P. P., Fiori, S., Marcello, A., Oggiano, S., Balmas, V., et al. (2018). Effect of yeast volatile organic compounds on ochratoxin A-producing Aspergillus carbonarius and A. ochraceus. Int. J. Food Microbiol. 284, 1–10. doi: 10.1016/j.ijfoodmicro.2018.06.023
Fernando, W. G. D., Ramarathnam, R., Krishnamoorthy, A. S., and Savchuk, S. C. (2005). Identification and use of potential bacterial organic antifungal volatiles in biocontrol. Soil Biol. Biochem. 37, 955–964. doi: 10.1016/j.soilbio.2004.10.021
Fialho, M. B., de Andrade, A., Bonatto, J. M. C., Salvato, F., Labate, C. A., and Pascholati, S. F. (2016). Proteomic response of the phytopathogen Phyllosticta citricarpa to antimicrobial volatile organic compounds from Saccharomyces cerevisiae. Microbiol. Res. 183, 1–7. doi: 10.1016/j.micres.2015.11.002
Fialho, M. B., Toffano, L., Pedroso, M. P., Augusto, F., and Pascholati, S. F. (2010). Volatile organic compounds produced by Saccharomyces cerevisiae inhibit the in vitro development of Guignardia citricarpa, the causal agent of citrus black spot. World J. Microbiol. Biotechnol. 26, 925–932. doi: 10.1007/s11274-009-0255-4
Fiddaman, P. J., and Rossall, S. (1993). The production of antifungal volatiles by Bacillus subtilis. J. Appl. Bacteriol. 74, 119–126. doi: 10.1111/j.1365-2672.1993.tb03004.x
Fiddaman, P. J., and Rossall, S. (1994). Effect of substrate on the production of antifungal volatiles from Bacillus subtilis. J. Appl. Bacteriol. 76, 395–405. doi: 10.1111/j.1365-2672.1994.tb01646.x
Fiedler, K., Schütz, E., and Geh, S. (2001). Detection of microbial volatile organic compounds (MVOCs) produced by moulds on various materials. Int. J. Hyg. Environ. Health 204, 111–121. doi: 10.1078/1438-4639-00094
Fincheira, P., Quiroz, A., Medina, C., Tortella, G., Hermosilla, E., Diez, M. C., et al. (2020). Plant growth induction by volatile organic compound released from solid lipid nanoparticles and nanostructured lipid carriers. Colloids Surfaces A Physicochem. Eng. Asp. 596:124739. doi: 10.1016/j.colsurfa.2020.124739
Freitas, C. S. A., Maciel, L. F., Corrêa dos Santos, R. A., Costa, O. M. M. M., Maia, F. C. B., Rabelo, R. S., et al. (2022). Bacterial volatile organic compounds induce adverse ultrastructural changes and DNA damage to the sugarcane pathogenic fungus Thielaviopsis ethacetica. Environ. Microbiol. doi: 10.1111/1462-2920.15876
Gao, H., Li, P., Xu, X., Zeng, Q., and Guan, W. (2018). Research on volatile organic compounds from Bacillus subtilis CF-3: biocontrol effects on fruit fungal pathogens and dynamic changes during fermentation. Front. Microbiol. 9:456. doi: 10.3389/fmicb.2018.00456
Gao, Z., Zhang, B., Liu, H., Han, J., and Zhang, Y. (2017). Identification of endophytic bacillus velezensis ZSY-1 strain and antifungal activity of its volatile compounds against Alternaria solani and Botrytis cinerea. Biol. Control 105, 27–39. doi: 10.1016/j.biocontrol.2016.11.007
Garbeva, P., Hordijk, C., Gerards, S., and de Boer, W. (2014). Volatiles produced by the mycophagous soil bacterium Collimonas. FEMS Microbiol. Ecol. 87, 639–649. doi: 10.1111/1574-6941.12252
Genin, S., Brito, B., Denny, T. P., and Boucher, C. (2005). Control of the Ralstonia solanacearum type III secretion system (Hrp) genes by the global virulence regulator PhcA. FEBS Lett. 579, 2077–2081. doi: 10.1016/j.febslet.2005.02.058
Giorgio, A., De Stradis, A., Lo Cantore, P., and Iacobellis, N. S. (2015). Biocide effects of volatile organic compounds produced by potential biocontrol rhizobacteria on Sclerotinia sclerotiorum. Front. Microbiol. 6:1056. doi: 10.3389/fmicb.2015.01056
Goel, S. (eds.) (2017). “Solid and hazardous waste management: an introduction,” in Advances in Solid and Hazardous Waste Management (New Dehli, India: Springer International Publishing), 1–27.
Goelen, T., Sobhy, I. S., Vanderaa, C., Wäckers, F., Rediers, H., Wenseleers, T., et al. (2020). Bacterial phylogeny predicts volatile organic compound composition and olfactory response of an aphid parasitoid. Oikos 129, 1415–1428. doi: 10.1111/oik.07301
Gómez, Á. G., Ramos, F. A., and Sinuco, D. C. (2021). Screening of Volatile Organic Compounds from Actinobacteria for the Control of Phytopathogen Colletotrichum gloeosporioides, vol. 31, 1067–1079.
Gotor-Vila, A., Teixidó, N., Di Francesco, A., Usall, J., Ugolini, L., Torres, R., et al. (2017). Antifungal effect of volatile organic compounds produced by Bacillus amyloliquefaciens CPA-8 against fruit pathogen decays of cherry. Food Microbiol. 64, 219–225. doi: 10.1016/j.fm.2017.01.006
Green, K. K., Stenberg, J. A., and Lankinen, Å. (2020). Making sense of integrated Pest management (IPM) in the light of evolution. Evol. Appl. 13, 1791–1805. doi: 10.1111/eva.13067
Groenhagen, U., Baumgartner, R., Bailly, A., Gardiner, A., Eberl, L., Schulz, S., et al. (2013). Production of bioactive volatiles by different Burkholderia ambifaria strains. J. Chem. Ecol. 39, 892–906. doi: 10.1007/s10886-013-0315-y
Grzegorczyk, M., Żarowska, B., Restuccia, C., and Cirvilleri, G. (2017). Postharvest biocontrol ability of killer yeasts against Monilinia fructigena and Monilinia fructicola on stone fruit. Food Microbiol. 61, 93–101. doi: 10.1016/j.fm.2016.09.005
Gu, Y. Q., Mo, M. H., Zhou, J. P., Zou, C. S., and Zhang, K. Q. (2007). Evaluation and identification of potential organic nematicidal volatiles from soil bacteria. Soil Biol. Biochem. 39, 2567–2575. doi: 10.1016/j.soilbio.2007.05.011
Guevara-Avendaño, E., Bejarano-Bolívar, A. A., Kiel-Martínez, A. L., Ramírez-Vázquez, M., Méndez-Bravo, A., von Wobeser, E. A., et al. (2019). Avocado rhizobacteria emit volatile organic compounds with antifungal activity against Fusarium solani, Fusarium sp. associated with Kuroshio shot hole borer, and Colletotrichum gloeosporioides. Microbiol. Res. 219, 74–83. doi: 10.1016/j.micres.2018.11.009
Guo, Y., Ghirardo, A., Weber, B., Schnitzler, J. P., Philipp Benz, J., and Rosenkranz, M. (2019b). Trichoderma species differ in their volatile profiles and in antagonism toward ectomycorrhiza Laccaria bicolor. Front. Microbiol. 10:891. doi: 10.3389/fmicb.2019.00891
Guo, H., Qin, X., Wu, Y., Yu, W., Liu, J., Xi, Y., et al. (2019a). Biocontrol of gray mold of cherry tomatoes with the volatile organic monomer from Hanseniaspora uvarum, trans-cinnamaldehyde. Food Bioprocess Technol. 12, 1809–1820. doi: 10.1007/s11947-019-02319-6
Han, S. H., Lee, S. J., Moon, J. H., Park, K. H., Yang, K. Y., Cho, B. H., et al. (2006). GacS-dependent production of 2R, 3R-butanediol by Pseudomonas chlororaphis O6 is a major determinant for eliciting systemic resistance against Erwinia carotovora but not against Pseudomonas syringae pv. Tabaci in tobacco. Mol. Plant-Microbe Interact. 19, 924–930. doi: 10.1094/MPMI-19-0924
Helander, I. M., Alakomi, H. L., Latva-Kala, K., Mattila-Sandholm, T., Pol, I., Smid, E. J., et al. (1998). Characterization of the action of selected essential oil components on gram-negative bacteria. J. Agric. Food Chem. 46, 3590–3595. doi: 10.1021/jf980154m
Herrington, P. R., Craig, J. T., and Sheridan, J. E. (1987). Methyl vinyl ketone: a volatile fungistatic inhibitor from Streptomyces griseoruber. Soil Biol. Biochem. 19, 509–512. doi: 10.1016/0038-0717(87)90092-7
Hikichi, Y., Mori, Y., Ishikawa, S., Hayashi, K., Ohnishi, K., Kiba, A., et al. (2017). Regulation involved in colonization of intercellular spaces of host plants in Ralstonia solanacearum. Front. Plant Sci. 8:967. doi: 10.3389/fpls.2017.00967
Holopainen, J. K. (2004). Multiple functions of inducible plant volatiles. Trends Plant Sci. 9, 529–533. doi: 10.1016/j.tplants.2004.09.006
Holopainen, J. K., and Blande, J. D. (2012). Molecular plant volatile communication. Adv. Exp. Med. Biol. 739, 17–31. doi: 10.1007/978-1-4614-1704-0_2
Howell, C. R. (1988). Production of ammonia by Enterobacter cloacae and its possible role in the biological control of Pythium preemergence damping-off by the bacterium. Phytopathology 78:1075. doi: 10.1094/Phyto-78-1075
Hua, S. S. T., Beck, J. J., Sarreal, S. B. L., and Gee, W. (2014). The major volatile compound 2-phenylethanol from the biocontrol yeast, Pichia anomala, inhibits growth and expression of aflatoxin biosynthetic genes of Aspergillus flavus. Mycotoxin Res. 30, 71–78. doi: 10.1007/s12550-014-0189-z
Huang, Q., and Allen, C. (2000). Polygalacturonases are required for rapid colonization and full virulence of Ralstonia solanacearum on tomato plants. Physiol. Mol. Plant Pathol. 57, 77–83. doi: 10.1006/pmpp.2000.0283
Huang, J. S., Peng, Y. H., Chung, K. R., and Huang, J. W. (2018). Suppressive efficacy of volatile compounds produced by Bacillus mycoides on damping-off pathogens of cabbage seedlings. J. Agric. Sci. 156, 795–809. doi: 10.1017/S0021859618000746
Hwang, H.-H., Yu, M., and Lai, E.-M. (2017). Agrobacterium-mediated plant transformation: biology and applications. Arab. B. 15:e0186. doi: 10.1199/tab.0186
Illana, A., Rodriguez-Romero, J., and Sesma, A. (2013). “Major plant pathogens of the Magnaporthaceae family,” in Genomics of Soil- and Plant-Associated Fungi. eds. B. A. Horwitz, P. K. Mukherjee, M. Mukherjee, and C. P. Kubiek (Springer, Berlin, Heidelberg), 45–88
Jamalizadeh, M., Etebarian, H. R., Aminian, H., and Alizadeh, A. (2010). Biological control of Botrytis Mali on apple fruit by use of Bacillus bacteria, isolated from the rhizosphere of wheat. Arch. Phytopathol. Plant Prot. 43, 1836–1845. doi: 10.1080/03235400902830960
Jeleń, H. H. (2002). Volatile sesquiterpene hydrocarbons characteristic for Penicillium roqueforti strains producing PR toxin. J. Agric. Food Chem. 50, 6569–6574. doi: 10.1021/jf020311o
Jeong, J. J., Moon, H. J., Pathiraja, D., Park, B., Choi, I. G., and Kim, K. D. (2018). Draft genome sequences of Bacillus megaterium KU143, Microbacterium testaceum KU313, and Pseudomonas protegens AS15, isolated from stored rice grains. Genome Announc. 6, 468–486. doi: 10.1128/genomeA.00468-18
Jiang, C. H., Liao, M. J., Wang, H. K., Zheng, M. Z., Xu, J. J., and Guo, J. H. (2018). Bacillus velezensis, a potential and efficient biocontrol agent in control of pepper gray mold caused by Botrytis cinerea. Biol. Control 126, 147–157. doi: 10.1016/j.biocontrol.2018.07.017
Kaddes, A., Fauconnier, M.-L., Sassi, K., Nasraoui, B., and Jijakli, M. H. (2019). Antifungal properties of two volatile organic compounds on barley pathogens and introduction to their mechanism of action. Int. J. Environ. Res. Public Health 16:2866. doi: 10.3390/ijerph16162866
Kai, M., Effmert, U., Berg, G., and Piechulla, B. (2007). Volatiles of bacterial antagonists inhibit mycelial growth of the plant pathogen Rhizoctonia solani. Arch. Microbiol. 187, 351–360. doi: 10.1007/s00203-006-0199-0
Kai, M., Effmert, U., and Piechulla, B. (2016). Bacterial-plant-interactions: approaches to unravel the biological function of bacterial volatiles in the rhizosphere. Front. Microbiol. 7:108. doi: 10.3389/fmicb.2016.00108
Kamoun, S., Furzer, O., Jones, J. D. G., Judelson, H. S., Ali, G. S., Dalio, R. J. D., et al. (2015). The top 10 oomycete pathogens in molecular plant pathology. Mol. Plant Pathol. 16, 413–434. doi: 10.1111/mpp.12190
Kanchiswamy, C. N., Malnoy, M., and Maffei, M. E. (2015). Chemical diversity of microbial volatiles and their potential for plant growth and productivity. Front. Plant Sci. 6:151. doi: 10.3389/fpls.2015.00151
Kandasamy, D., Gershenzon, J., Andersson, M. N., and Hammerbacher, A. (2019). Volatile organic compounds influence the interaction of the Eurasian spruce bark beetle (Ips typographus) with its fungal symbionts. ISME J. 13, 1788–1800. doi: 10.1038/s41396-019-0390-3
Kannan, V., Bastas, K., and Devi, R. (2015). “Scientific and economic impact of plant pathogenic bacteria” in Sustainable Approaches to Controlling Plant Pathogenic Bacteria. eds. V. R. Kannan and K. K. Bastas (Boca Raton, FL, CRC Press), 369–392.
Khokhani, D., Lowe-Power, T. M., Tran, T. M., and Allen, C. (2017). A single regulator mediates strategic switching between attachment/spread and growth/virulence in the plant pathogen Ralstonia solanacearum. mBio 8:e00895-17. doi: 10.1128/mBio.00895-17
Koitabashi, M., Iwano, M., and Tsushima, S. (2002). Aromatic substances inhibiting wheat powdery mildew produced by a fungus detected with a new screening method for phylloplane fungi. J. Gen. Plant Pathol. 68, 183–188. doi: 10.1007/PL00013074
Koitabashi, M., Kajitani, Y., and Hirashima, K. (2004). Antifungal substances produced by fungal strain Kyu-W63 from wheat leaf and its taxonomic position. J. Gen. Plant Pathol. 702, 124–130. doi: 10.1007/S10327-003-0095-2
Kong, H. G., Song, G. C., Sim, H. J., and Ryu, C. M. (2021). Achieving similar root microbiota composition in neighbouring plants through airborne signalling. ISME J. 15, 397–408. doi: 10.1038/s41396-020-00759-z
Korpi, A., Järnberg, J., and Pasanen, A. L. (2009). Microbial volatile organic compounds. Crit. Rev. Toxicol. 39, 139–193. doi: 10.1080/10408440802291497
Kumar, D., and Kalita, P. (2017). Reducing postharvest losses during storage of grain crops to strengthen food security in developing countries. Foods 6:8. doi: 10.3390/foods6010008
Lee, S., Yap, M., Behringer, G., Hung, R., and Bennett, J. W. (2016). Volatile organic compounds emitted by Trichoderma species mediate plant growth. Fungal Biol. Biotechnol. 3, 1–14. doi: 10.1186/s40694-016-0025-7
Li, N., Alfiky, A., Vaughan, M. M., and Kang, S. (2016). Stop and smell the fungi: fungal volatile metabolites are overlooked signals involved in fungal interaction with plants. Fungal Biol. Rev. 30, 134–144. doi: 10.1016/j.fbr.2016.06.004
Li, X. Y., Mao, Z. C., Wu, Y. X., Ho, H. H., and He, Y. Q. (2015). Comprehensive volatile organic compounds profiling of Bacillus species with biocontrol properties by head space solid-phase microextraction with gas chromatography-mass spectrometry. Biocontrol Sci. Tech. 25, 132–143. doi: 10.1080/09583157.2014.960809
Liu, W. W., Mu, W., Zhu, B. Y., Du, Y. C., and Liu, F. (2008). Antagonistic activities of volatiles from four strains of Bacillus spp. and Paenibacillus spp. against soil-borne plant pathogens. Agric. Sci. China 7, 1104–1114. doi: 10.1016/S1671-2927(08)60153-4
Liu, R., Xu, C., Zhang, Q., Wang, S., and Fang, W. (2017). Evolution of the chitin synthase gene family correlates with fungal morphogenesis and adaption to ecological niches. Sci. Rep. 7:44527. doi: 10.1038/srep44527
Ma, B., Hibbing, M. E., Kim, H. S., Reedy, R. M., Yedidia, I., Breuer, J., et al. (2007). Host range and molecular phylogenies of the soft rot enterobacterial genera Pectobacterium and Dickeya. Phytopathology 97, 1150–1163. doi: 10.1094/PHYTO-97-9-1150
Macías-Rubalcava, M. L., Hernández-Bautista, B. E., Oropeza, F., Duarte, G., González, M. C., Glenn, A. E., et al. (2010). Allelochemical effects of volatile compounds and organic extracts from Muscodor yucatanensis, a tropical Endophytic fungus from Bursera simaruba. J. Chem. Ecol. 36, 1122–1131. doi: 10.1007/s10886-010-9848-5
Macías-Rubalcava, M. L., Sánchez-Fernández, R. E., Roque-Flores, G., Lappe-Oliveras, P., and Medina-Romero, Y. M. (2018). Volatile organic compounds from Hypoxylon anthochroum endophytic strains as postharvest mycofumigation alternative for cherry tomatoes. Food Microbiol. 76, 363–373. doi: 10.1016/j.fm.2018.06.014
Maffei, M. E. (2010). Sites of synthesis, biochemistry and functional role of plant volatiles. South African J. Bot. 76, 612–631. doi: 10.1016/j.sajb.2010.03.003
Makovitzki, A., Viterbo, A., Brotman, Y., Chet, I., and Shai, Y. (2007). Inhibition of fungal and bacterial plant pathogens in vitro and in planta with ultrashort cationic lipopeptides. Appl. Environ. Microbiol. 73, 6629–6636. doi: 10.1128/AEM.01334-07
Mansfield, J., Genin, S., Magori, S., Citovsky, V., Sriariyanum, M., Ronald, P., et al. (2012). Top 10 plant pathogenic bacteria in molecular plant pathology. Mol. Plant Pathol. 13, 614–629. doi: 10.1111/j.1364-3703.2012.00804.x
Marques, J., Oliveira, L. F., Pinto, R. T., Coutinho, P. J. G., Parpot, P., Góis, J. R., et al. (2013). Release of volatile compounds from polymeric microcapsules mediated by photocatalytic nanoparticles. Int. J. Photoenergy 2013:712603. doi: 10.1155/2013/712603
Martins, S. J., Faria, A. F., Pedroso, M. P., Cunha, M. G., Rocha, M. R., and Medeiros, F. H. V. (2019). Microbial volatiles organic compounds control anthracnose (Colletotrichum lindemuthianum) in common bean (Phaseolus vulgaris L.). Biol. Control 131, 36–42. doi: 10.1016/j.biocontrol.2019.01.003
Martins, P. M. M., Merfa, M. V., Takita, M. A., and De Souza, A. A. (2018). Persistence in phytopathogenic bacteria: do we know enough? Front. Microbiol. 9:1099. doi: 10.3389/fmicb.2018.01099
Masoud, W., Poll, L., and Jakobsen, M. (2005). Influence of volatile compounds produced by yeasts predominant during processing of Coffea arabica in East Africa on growth and ochratoxin A (OTA) production by Aspergillus ochraceus. Yeast 22, 1133–1142. doi: 10.1002/yea.1304
Matsui, K. (2006). Green leaf volatiles: hydroperoxide lyase pathway of oxylipin metabolism. Curr. Opin. Plant Biol. 9, 274–280. doi: 10.1016/j.pbi.2006.03.002
Medina-Romero, Y. M., Roque-Flores, G., and Macías-Rubalcava, M. L. (2017). Volatile organic compounds from endophytic fungi as innovative postharvest control of Fusarium oxysporum in cherry tomato fruits. Appl. Microbiol. Biotechnol. 101, 8209–8222. doi: 10.1007/s00253-017-8542-8
Meldau, D. G., Meldau, S., Hoang, L. H., Underberg, S., Wünsche, H., and Baldwin, I. T. (2013). Dimethyl disulfide produced by the naturally associated bacterium Bacillus sp. B55 promotes Nicotiana attenuata growth by enhancing sulfur nutrition. Plant Cell 25, 2731–2747. doi: 10.1105/tpc.113.114744
Mewa-Ngongang, M., Du Plessis, H. W., Ntwampe, S. K. O., Chidi, B. S., Hutchinson, U. F., Mekuto, L., et al. (2019). The use of Candida pyralidae and Pichia kluyveri to control spoilage microorganisms of raw fruits used for beverage production. Foods 8:454. doi: 10.3390/foods8100454
Minerdi, D., Bossi, S., Gullino, M. L., and Garibaldi, A. (2009). Volatile organic compounds: a potential direct long-distance mechanism for antagonistic action of Fusarium oxysporum strain MSA 35. Environ. Microbiol. 11, 844–854. doi: 10.1111/j.1462-2920.2008.01805.x
Minerdi, D., Bossi, S., Maffei, M. E., Gullino, M. L., and Garibaldi, A. (2011). Fusarium oxysporum and its bacterial consortium promote lettuce growth and expansin A5 gene expression through microbial volatile organic compound (MVOC) emission. FEMS Microbiol. Ecol. 76, 342–351. doi: 10.1111/j.1574-6941.2011.01051.x
Mirabella, R., Rauwerda, H., Allmann, S., Scala, A., Spyropoulou, E. A., de Vries, M., et al. (2015). WRKY40 and WRKY6 act downstream of the green leaf volatile E-2-hexenal in Arabidopsis. Plant J. 83, 1082–1096. doi: 10.1111/tpj.12953
Mishra, V. K., Passari, A. K., Chandra, P., Leo, V. V., Kumar, B., Uthandi, S., et al. (2017). Determination and production of antimicrobial compounds by Aspergillus clavatonanicus strain MJ31, an endophytic fungus from Mirabilis Jalapa L. using UPLC-ESI-MS/MS and TD-GC-MS analysis. PLoS One 12, 1–24. doi: 10.1371/journal.pone.0186234
Misztal, P. K., Lymperopoulou, D. S., Adams, R. I., Scott, R. A., Lindow, S. E., Bruns, T., et al. (2018). Emission factors of microbial volatile organic compounds from environmental bacteria and fungi. Environ. Sci. Technol. 52, 8272–8282. doi: 10.1021/acs.est.8b00806
Mitchell, A. M., Strobel, G. A., Moore, E., Robison, R., and Sears, J. (2010). Volatile antimicrobials from Muscodor crispans, a novel endophytic fungus. Microbiology 156, 270–277. doi: 10.1099/mic.0.032540-0
Morita, T., Tanaka, I., Ryuda, N., Ikari, M., Ueno, D., and Someya, T. (2019). Antifungal spectrum characterization and identification of strong volatile organic compounds produced by Bacillus pumilus TM-R. Heliyon 5:e01817. doi: 10.1016/j.heliyon.2019.e01817
Mun, H., and Townley, H. E. (2020). Nanoencapsulation of plant volatile organic compounds to improve their biological activities. Planta Med. 87, 236–251. doi: 10.1055/A-1289-4505
Munjal, V., Nadakkakath, A. V., Sheoran, N., Kundu, A., Venugopal, V., Subaharan, K., et al. (2016). Genotyping and identification of broad spectrum antimicrobial volatiles in black pepper root endophytic biocontrol agent, Bacillus megaterium BP17. Biol. Control 92, 66–76. doi: 10.1016/j.biocontrol.2015.09.005
Myo, E. M., Liu, B., Ma, J., Shi, L., Jiang, M., Zhang, K., et al. (2019). Evaluation of Bacillus velezensis NKG-2 for bio-control activities against fungal diseases and potential plant growth promotion. Biol. Control 134, 23–31. doi: 10.1016/j.biocontrol.2019.03.017
Nakkeeran, S., Fernando, W. G. D., and Siddiqui, Z. A. (2006). “Plant growth promoting rhizobacteria formulations and its scope in commercialization for the management of pests and diseases” in PGPR: Biocontrol and Biofertilization. ed. Z. A. Siddiqui (Netherlands: Springer), 257–296.
Naznin, H. A., Kimura, M., Miyazawa, M., and Hyakumachi, M. (2013). Analysis of volatile organic compounds emitted by plant growth-promoting fungus Phoma sp. GS8-3 for growth promotion effects on Tobacco. Microbes Environ. 28, 42–49. doi: 10.1264/jsme2.ME12085
Naznin, H. A., Kiyohara, D., Kimura, M., Miyazawa, M., Shimizu, M., and Hyakumachi, M. (2014). Systemic resistance induced by volatile organic compounds emitted by plant growth-promoting fungi in Arabidopsis thaliana. PLoS One 9:e86882. doi: 10.1371/journal.pone.0086882
Nieto-Jacobo, M. F., Steyaert, J. M., Salazar-Badillo, F. B., Vi Nguyen, D., Rostás, M., Braithwaite, M., et al. (2017). Environmental growth conditions of Trichoderma spp. affects indole acetic acid derivatives, volatile organic compounds, and plant growth promotion. Front. Plant Sci. 8:102. doi: 10.3389/fpls.2017.00102
Ossowicki, A., Jafra, S., and Garbeva, P. (2017). The antimicrobial volatile power of the rhizospheric isolate pseudomonas donghuensis P482. PLoS One 12:e0174362. doi: 10.1371/journal.pone.0174362
Paolo, W. F., Dadachova, E., Mandal, P., Casadevall, A., Szaniszlo, P. J., and Nosanchuk, J. D. (2006). Effects of disrupting the polyketide synthase gene WdPKS1 in Wangiella [Exophiala] dermatitidis on melanin production and resistance to killing by antifungal compounds, enzymatic degradation, and extremes in temperature. BMC Microbiol. 6:55. doi: 10.1186/1471-2180-6-55
Papaleo, M. C., Fondi, M., Maida, I., Perrin, E., Lo Giudice, A., Michaud, L., et al. (2012). Sponge-associated microbial Antarctic communities exhibiting antimicrobial activity against Burkholderia cepacia complex bacteria. Biotechnol. Adv. 30, 272–293. doi: 10.1016/j.biotechadv.2011.06.011
Parafati, L., Vitale, A., Restuccia, C., and Cirvilleri, G. (2015). Biocontrol ability and action mechanism of food-isolated yeast strains against Botrytis cinerea causing post-harvest bunch rot of table grape. Food Microbiol. 47, 85–92. doi: 10.1016/j.fm.2014.11.013
Parafati, L., Vitale, A., Restuccia, C., and Cirvilleri, G. (2017). Performance evaluation of volatile organic compounds by antagonistic yeasts immobilized on hydrogel spheres against gray, green and blue postharvest decays. Food Microbiol. 63, 191–198. doi: 10.1016/j.fm.2016.11.021
Park, H. B., Lee, B., Kloepper, J. W., and Ryu, C.-M. (2013). One shot-two pathogens blocked. Plant Signal. Behav. 8:e24619. doi: 10.4161/psb.24619
Peeters, N., Guidot, A., Vailleau, F., and Valls, M. (2013). Ralstonia solanacearum, a widespread bacterial plant pathogen in the post-genomic era. Mol. Plant Pathol. 14, 651–662. doi: 10.1111/mpp.12038
Peñuelas, J., Asensio, D., Tholl, D., Wenke, K., Rosenkranz, M., Piechulla, B., et al. (2014). Biogenic volatile emissions from the soil. Plant Cell Environ. 37, 1866–1891. doi: 10.1111/pce.12340
Peres, N. A., Timmer, L. W., Adaskaveg, J. E., and Correll, J. C. (2005). Lifestyles of Colletotrichum acutatum. Plant Dis. 89, 784–796. doi: 10.1094/PD-89-0784
Perfect, S. E., Hughes, H. B., O’Connell, R. J., and Green, J. R. (1999). Colletotrichum: A model genus for studies on pathology and fungal-plant interactions. Fungal Genet. Biol. 27, 186–198. doi: 10.1006/fgbi.1999.1143
Pfeilmeier, S., Caly, D. L., and Malone, J. G. (2016). Bacterial pathogenesis of plants: future challenges from a microbial perspective. Mol. Plant Pathol. 17, 1298–1313. doi: 10.1111/mpp.12427
Pimenta, R. S., Moreira da Silva, J. F., Buyer, J. S., and Janisiewicz, W. J. (2012). Endophytic fungi from plums (Prunus domestica) and their antifungal activity against Monilinia fructicola. J. Food Prot. 75, 1883–1889. doi: 10.4315/0362-028X.JFP-12-156
Pineda, A., Soler, R., Weldegergis, B. T., Shimwela, M. M., Van Loon, J. J. A., and Dicke, M. (2013). Non-pathogenic rhizobacteria interfere with the attraction of parasitoids to aphid-induced plant volatiles via jasmonic acid signalling. Plant Cell Environ. 36, 393–404. doi: 10.1111/j.1365-3040.2012.02581.x
Plyuta, V., Lipasova, V., Popova, A., Koksharova, O., Kuznetsov, A., Szegedi, E., et al. (2016). Influence of volatile organic compounds emitted by pseudomonas and Serratia strains on agrobacterium tumefaciens biofilms. APMIS 124, 586–594. doi: 10.1111/apm.12547
Popova, A. A., Koksharova, O. A., Lipasova, V. A., Zaitseva, J. V., Katkova-Zhukotskaya, O. A., Eremina, S. I., et al. (2014). Inhibitory and toxic effects of volatiles emitted by strains of Pseudomonas and Serratia on growth and survival of selected microorganisms, Caenorhabditis elegans, and Drosophila melanogaster. Biomed. Res. Int. 2014:125704. doi: 10.1155/2014/125704
Preston, G. M. (2000). Pseudomonas syringae pv. Tomato: the right pathogen, of the right plant, at the right time. Mol. Plant Pathol. 1, 263–275. doi: 10.1046/j.1364-3703.2000.00036.x
Prior, P., Ailloud, F., Dalsing, B. L., Remenant, B., Sanchez, B., and Allen, C. (2016). Genomic and proteomic evidence supporting the division of the plant pathogen Ralstonia solanacearum into three species. BMC Genomics 17:90. doi: 10.1186/s12864-016-2413-z
Qadri, M., Deshidi, R., Shah, B. A., Bindu, K., Vishwakarma, R. A., and Riyaz-Ul-Hassan, S. (2015). An endophyte of Picrorhiza kurroa Royle ex. Benth, producing menthol, phenylethyl alcohol and 3-hydroxypropionic acid, and other volatile organic compounds. World J. Microbiol. Biotechnol. 31, 1647–1654. doi: 10.1007/s11274-015-1910-6
Qin, X., Xiao, H., Cheng, X., Zhou, H., and Si, L. (2017). Hanseniaspora uvarum prolongs shelf life of strawberry via volatile production. Food Microbiol. 63, 205–212. doi: 10.1016/j.fm.2016.11.005
Rajani, P., Rajasekaran, C., Vasanthakumari, M. M., Olsson, S. B., Ravikanth, G., and Uma Shaanker, R. (2021). Inhibition of plant pathogenic fungi by endophytic Trichoderma spp. through mycoparasitism and volatile organic compounds. Microbiol. Res. 242:126595. doi: 10.1016/j.micres.2020.126595
Rajer, F. U., Wu, H., Xie, Y., Xie, S., Raza, W., Tahir, H. A. S., et al. (2017). Volatile organic compounds produced by a soil-isolate, Bacillus subtilis FA26 induce adverse ultra-structural changes to the cells of Clavibacter michiganensis ssp. Sepedonicus, the causal agent of bacterial ring rot of potato. Microbiol. (United Kingdom) 163, 523–530. doi: 10.1099/mic.0.000451
Rathore, S., Desai, P. M., Liew, C. V., Chan, L. W., and Heng, P. W. S. (2013). Microencapsulation of microbial cells. J. Food Eng. 116, 369–381. doi: 10.1016/j.jfoodeng.2012.12.022
Raza, W., Ling, N., Liu, D., Wei, Z., Huang, Q., and Shen, Q. (2016a). Volatile organic compounds produced by Pseudomonas fluorescens WR-1 restrict the growth and virulence traits of Ralstonia solanacearum. Microbiol. Res. 192, 103–113. doi: 10.1016/j.micres.2016.05.014
Raza, W., Ling, N., Yang, L., Huang, Q., and Shen, Q. (2016b). Response of tomato wilt pathogen Ralstonia solanacearum to the volatile organic compounds produced by a biocontrol strain Bacillus amyloliquefaciens SQR-9. Sci. Rep. 6:24856. doi: 10.1038/srep24856
Raza, W., Wang, J., Wu, Y., Ling, N., Wei, Z., Huang, Q., et al. (2016c). Effects of volatile organic compounds produced by Bacillus amyloliquefaciens on the growth and virulence traits of tomato bacterial wilt pathogen Ralstonia solanacearum. Appl. Microbiol. Biotechnol. 100, 7639–7650. doi: 10.1007/s00253-016-7584-7
Raza, W., Yuan, J., Ling, N., Huang, Q., and Shen, Q. (2015). Production of volatile organic compounds by an antagonistic strain Paenibacillus polymyxa WR-2 in the presence of root exudates and organic fertilizer and their antifungal activity against Fusarium oxysporum f. sp. niveum. Biol. Control 80, 89–95. doi: 10.1016/j.biocontrol.2014.09.004
Reverchon, F., García-Quiroz, W., Guevara-Avendaño, E., Solís-García, I. A., Ferrera-Rodríguez, O., and Lorea-Hernández, F. (2019). Antifungal potential of Lauraceae rhizobacteria from a tropical montane cloud forest against Fusarium spp. Brazilian J. Microbiol. 50, 583–592. doi: 10.1007/s42770-019-00094-2
Riera, N., Handique, U., Zhang, Y., Dewdney, M. M., and Wang, N. (2017). Characterization of antimicrobial-producing beneficial bacteria isolated from Huanglongbing escape citrus trees. Front. Microbiol. 8:2415. doi: 10.3389/fmicb.2017.02415
Rojas-Solís, D., Zetter-Salmón, E., Contreras-Pérez, M., Rocha-Granados, C., Macías-Rodríguez, L., and Santoyo, G. (2018). Pseudomonas stutzeri E25 and Stenotrophomonas maltophilia CR71 endophytes produce antifungal volatile organic compounds and exhibit additive plant growth-promoting effects. Biocatal. Agric. Biotechnol. 13, 46–52. doi: 10.1016/j.bcab.2017.11.007
Romanazzi, G., Lichter, A., Gabler, F. M., and Smilanick, J. L. (2012). Recent advances on the use of natural and safe alternatives to conventional methods to control postharvest gray mold of table grapes. Postharvest Biol. Technol. 63, 141–147. doi: 10.1016/j.postharvbio.2011.06.013
Roncero, M. I. G., Hera, C., Ruiz-Rubio, M., García Maceira, F. I., Madrid, M. P., Caracuel, Z., et al. (2003). Fusarium as a model for studying virulence in soilborne plant pathogens. Physiol. Mol. Plant Pathol. 62, 87–98. doi: 10.1016/S0885-5765(03)00043-2
Ryu, C. M., Farag, M. A., Hu, C. H., Reddy, M. S., Kloepper, J. W., and Paré, P. W. (2004). Bacterial volatiles induce systemic resistance in Arabidopsis. Plant Physiol. 134, 1017–1026. doi: 10.1104/pp.103.026583
Ryu, C. M., Farag, M. A., Hu, C. H., Reddy, M. S., Wei, H. X., Paré, P. W., et al. (2003). Bacterial volatiles promote growth in Arabidopsis. Proc. Natl. Acad. Sci. U. S. A. 100, 4927–4932. doi: 10.1073/pnas.0730845100
Safni, I., Cleenwerck, I., De Vos, P., Fegan, M., Sly, L., and Kappler, U. (2014). Polyphasic taxonomic revision of the Ralstonia solanacearum species complex: proposal to emend the descriptions of Ralstonia solanacearum and Ralstonia syzygii and reclassify current R. syzygii strains as Ralstonia syzygii subsp. syzygii subsp. nov., R. solanacearum phylotype IV strains as Ralstonia syzygii subsp. indonesiensis subsp. nov., banana blood disease bacterium strains as Ralstonia syzygii subsp. celebesensis subsp. nov. and R. solanacearum phylotype I and III strains as Ralstonia pseudosolanacearum sp. nov. Int. J. Syst. Evol. Microbiol. 64, 3087–3103. doi: 10.1099/ijs.0.066712-0
Sahu, P. K., Gupta, A., Kedarnath Kumari, P., Lavanya, G., and Yadav, A. K. (2017). “Attempts for biological control of Ralstonia solanacearum by using beneficial microorganisms” in Agriculturally Important Microbes for Sustainable Agriculture. eds. V. S. Meen, P. K. Mishra, J. K. Bisht, and A. Pattanayak (Singapore: Springer), 315–342.
Sánchez-Fernández, R. E., Diaz, D., Duarte, G., Lappe-Oliveras, P., Sánchez, S., and Macías-Rubalcava, M. L. (2016). Antifungal volatile organic compounds from the endophyte Nodulisporium sp. strain GS4d2II1a: a qualitative change in the intraspecific and interspecific interactions with Pythium aphanidermatum. Microb. Ecol. 71, 347–364. doi: 10.1007/s00248-015-0679-3
Scala, A., Allmann, S., Mirabella, R., Haring, M., and Schuurink, R. (2013). Green leaf volatiles: a plant’s multifunctional weapon against herbivores and pathogens. Int. J. Mol. Sci. 14, 17781–17811. doi: 10.3390/ijms140917781
Schausberger, P., Peneder, S., Jürschik, S., and Hoffmann, D. (2012). Mycorrhiza changes plant volatiles to attract spider mite enemies. Funct. Ecol. 26, 441–449. doi: 10.1111/j.1365-2435.2011.01947.x
Schulz, S., and Dickschat, J. S. (2007). Bacterial volatiles: the smell of small organisms. Nat. Prod. Rep. 24, 814–842. doi: 10.1039/b507392h
Schulz-Bohm, K., Gerards, S., Hundscheid, M., Melenhorst, J., de Boer, W., and Garbeva, P. (2018). Calling from distance: attraction of soil bacteria by plant root volatiles. ISME J. 12, 1252–1262. doi: 10.1038/s41396-017-0035-3
Sharifi, R., and Ryu, C. M. (2016). Are bacterial volatile compounds poisonous odors to a fungal pathogen Botrytis cinerea, alarm signals to arabidopsis seedlings for eliciting induced resistance, or both? Front. Microbiol. 7:196. doi: 10.3389/fmicb.2016.00196
Sharifi, R., and Ryu, C. M. (2018). Sniffing bacterial volatile compounds for healthier plants. Curr. Opin. Plant Biol. 44, 88–97. doi: 10.1016/j.pbi.2018.03.004
Sheoran, N., Valiya Nadakkakath, A., Munjal, V., Kundu, A., Subaharan, K., Venugopal, V., et al. (2015). Genetic analysis of plant endophytic Pseudomonas putida BP25 and chemo-profiling of its antimicrobial volatile organic compounds. Microbiol. Res. 173, 66–78. doi: 10.1016/j.micres.2015.02.001
Sim, C. S. F., Yue, C. S., Cheow, Y. L., and Ting, A. S. Y. (2019). Influence of metal stress on production of volatile inhibitory compounds by endophytes against Ganoderma boninense. Biocontrol Sci. Tech. 29, 860–876. doi: 10.1080/09583157.2019.1611735
Singh, B. K., Trivedi, P., Egidi, E., Macdonald, C. A., and Delgado-Baquerizo, M. (2020). Crop microbiome and sustainable agriculture. Nat. Rev. Microbiol. 18, 601–602. doi: 10.1038/s41579-020-00446-y
Smith, E. F., and Townsend, C. O. (1907). A plant-tumor of bacterial origin. Science 80, 671–673. doi: 10.1126/science.25.643.671
Soto, M. J., Sanjuán, J., and Olivares, J. (2006). Rhizobia and plant-pathogenic bacteria: common infection weapons. Microbiology 152, 3167–3174. doi: 10.1099/mic.0.29112-0
Spence, C., Alff, E., Johnson, C., Ramos, C., Donofrio, N., Sundaresan, V., et al. (2014). Natural rice rhizospheric microbes suppress rice blast infections. BMC Plant Biol. 14:130. doi: 10.1186/1471-2229-14-130
Stahl, P. D., and Parkin, T. B. (1996). Microbial production of volatile organic compounds in soil microcosms. Soil Sci. Soc. Am. J. 60, 821–828. doi: 10.2136/sssaj1996.03615995006000030020x
Strobel, G., Singh, S. K., Riyaz-Ul-Hassan, S., Mitchell, A. M., Geary, B., and Sears, J. (2011). An endophytic/pathogenic Phoma sp. from creosote bush producing biologically active volatile compounds having fuel potential. FEMS Microbiol. Lett. 320, 87–94. doi: 10.1111/j.1574-6968.2011.02297.x
Suda, Y., Tachikawa, H., Yokota, A., Nakanishi, H., Yamashita, N., Miura, Y., et al. (2003). Saccharomyces cerevisiae QNS1 codes for NAD+ synthetase that is functionally conserved in mammals. Yeast 20, 995–1005. doi: 10.1002/yea.1008
Syed-Ab-Rahman, S. F., Carvalhais, L. C., Chua, E. T., Chung, F. Y., Moyle, P. M., Eltanahy, E. G., et al. (2019). Soil bacterial diffusible and volatile organic compounds inhibit Phytophthora capsici and promote plant growth. Sci. Total Environ. 692, 267–280. doi: 10.1016/j.scitotenv.2019.07.061
Tahir, H. A. S., Gu, Q., Wu, H., Niu, Y., Huo, R., and Gao, X. (2017a). Bacillus volatiles adversely affect the physiology and ultra-structure of Ralstonia solanacearum and induce systemic resistance in tobacco against bacterial wilt. Sci. Rep. 7, 1–15. doi: 10.1038/srep40481
Tahir, H. A. S., Gu, Q., Wu, H., Raza, W., Hanif, A., Wu, L., et al. (2017b). Plant growth promotion by volatile organic compounds produced by Bacillus subtilis SYST2. Front. Microbiol. 8:171. doi: 10.3389/fmicb.2017.00171
Tenorio-Salgado, S., Tinoco, R., Vazquez-Duhalt, R., Caballero-Mellado, J., and Perez-Rueda, E. (2013). Identification of volatile compounds produced by the bacterium Burkholderia tropica that inhibit the growth of fungal pathogens. Bioengineered 4, 236–243. doi: 10.4161/bioe.23808
Thakeow, P., Angeli, S., Weißbecker, B., and Schütz, S. (2008). Antennal and behavioral responses of Cis boleti to fungal odor of Trametes gibbosa. Chem. Senses 33, 379–387. doi: 10.1093/chemse/bjn005
Thakur, N., Kaur, S., Tomar, P., Thakur, S., and Yadav, A. N. (2020). Microbial biopesticides: current status and advancement for sustainable agriculture and environment. New Futur. Dev. Microb. Biotechnol. Bioeng., 243–282. doi: 10.1016/B978-0-12-820526-6.00016-6
Tilocca, B., Balmas, V., Hassan, Z. U., Jaoua, S., and Migheli, Q. (2019). A proteomic investigation of Aspergillus carbonarius exposed to yeast volatilome or to its major component 2-phenylethanol reveals major shifts in fungal metabolism. Int. J. Food Microbiol. 306:108265. doi: 10.1016/j.ijfoodmicro.2019.108265
Tilocca, B., Cao, A., and Migheli, Q. (2020). Scent of a killer: microbial volatilome and its role in the biological control of plant pathogens. Front. Microbiol. 11:41. doi: 10.3389/FMICB.2020.00041/BIBTEX
Tjou-Tam-Sin, N. N. A., van de Bilt, J. L. J., Westenberg, M., Gorkink-Smits, P. P. M. A., Landman, N. M., and Bergsma-Vlami, M. (2017). Assessing the pathogenic ability of Ralstonia pseudosolanacearum (Ralstonia solanacearum phylotype I) from ornamental Rosa spp. plants. Front. Plant Sci. 8:1895. doi: 10.3389/fpls.2017.01895
Toth, I. K., and Birch, P. R. J. (2005). Rotting softly and stealthily. Curr. Opin. Plant Biol. 8, 424–429. doi: 10.1016/j.pbi.2005.04.001
United Nations (2022). World Population Prospects 2022: Summary of Results. New York. Available at: https://www.un.org/development/desa/pd/sites/www.un.org.development.desa.pd/files/wpp2022_summary_of_results.pdf (Accessed August 18, 2022).
Vaishnav, A., Kumari, S., Jain, S., Varma, A., and Choudhary, D. K. (2015). Putative bacterial volatile-mediated growth in soybean (Glycine max L. Merrill) and expression of induced proteins under salt stress. J. Appl. Microbiol. 119, 539–551. doi: 10.1111/jam.12866
Vespermann, A., Kai, M., and Piechulla, B. (2007). Rhizobacterial volatiles affect the growth of fungi and Arabidopsis thaliana. Appl. Environ. Microbiol. 73, 5639–5641. doi: 10.1128/AEM.01078-07
Vinale, F., Sivasithamparam, K., Ghisalberti, E. L., Marra, R., Barbetti, M. J., Li, H., et al. (2008). A novel role for Trichoderma secondary metabolites in the interactions with plants. Physiol. Mol. Plant Pathol. 72, 80–86. doi: 10.1016/j.pmpp.2008.05.005
Vlassi, A., Nesler, A., Perazzolli, M., Lazazzara, V., Büschl, C., Parich, A., et al. (2020). Volatile organic compounds from Lysobacter capsici AZ78 as potential candidates for biological control of soilborne plant pathogens. Front. Microbiol. 0:1748. doi: 10.3389/FMICB.2020.01748
Wallace, R. L., Hirkala, D. L., and Nelson, L. M. (2017). Postharvest biological control of blue mold of apple by Pseudomonas fluorescens during commercial storage and potential modes of action. Postharvest Biol. Technol. 133, 1–11. doi: 10.1016/j.postharvbio.2017.07.003
Wan, M., Li, G., Zhang, J., Jiang, D., and Huang, H. C. (2008). Effect of volatile substances of Streptomyces platensis F-1 on control of plant fungal diseases. Biol. Control 46, 552–559. doi: 10.1016/j.biocontrol.2008.05.015
Weise, T., Kai, M., Gummesson, A., Troeger, A., Von Reuß, S., Piepenborn, S., et al. (2012). Volatile organic compounds produced by the phytopathogenic bacterium Xanthomonas campestris pv. Vesicatoria 85-10. Beilstein J. Org. Chem. 8, 579–596. doi: 10.3762/bjoc.8.65
Wenke, K., Weise, T., Warnke, R., Valverde, C., Wanke, D., Kai, M., et al. (2012). “Bacterial volatiles mediating information between bacteria and plants” in Biocommunication of Plants. eds. G. Witzany and F. Baluška (Berlin, Heidelberg: Springer), 327–347.
Wheatley, R., Hackett, C., Bruce, A., and Kundzewicz, A. (1997). Effect of substrate composition on production of volatile organic compounds from Trichoderma spp. inhibitory to wood decay fungi. Int. Biodeterior. Biodegrad. 39, 199–205. doi: 10.1016/S0964-8305(97)00015-2
Williamson, B., Tudzynski, B., Tudzynski, P., and Van Kan, J. A. L. (2007). Botrytis cinerea: the cause of gray mould disease. Mol. Plant Pathol. 8, 561–580. doi: 10.1111/j.1364-3703.2007.00417.x
Wonglom, P., Daengsuwan, W., Ito, S., and Sunpapao, A. (2019). Biological control of Sclerotium fruit rot of snake fruit and stem rot of lettuce by Trichoderma sp. T76-12/2 and the mechanisms involved. Physiol. Mol. Plant Pathol. 107, 1–7. doi: 10.1016/j.pmpp.2019.04.007
Wu, Y., Yuan, J. E. Y., Raza, W., Shen, Q., and Huang, Q. (2015). Effects of volatile organic compounds from Streptomyces albulus NJZJSA2 on growth of two fungal pathogens. J. Basic Microbiol. 55, 1104–1117. doi: 10.1002/jobm.201400906
Xie, S., Zang, H., Jun Wu, H., Uddin Rajer, F., and Gao, X. (2018). Antibacterial effects of volatiles produced by Bacillus strain D13 against Xanthomonas oryzae pv. Oryzae. Mol. Plant Pathol. 19, 49–58. doi: 10.1111/mpp.12494
Xin, X. F., Kvitko, B., and He, S. Y. (2018). Pseudomonas syringae: what it takes to be a pathogen. Nat. Rev. Microbiol. 16, 316–328. doi: 10.1038/nrmicro.2018.17
Xing, M., Zheng, L., Deng, Y., Xu, D., Xi, P., Li, M., et al. (2018). Antifungal activity of natural volatile organic compounds against litchi downy blight pathogen Peronophythora litchii. Molecules 23:358. doi: 10.3390/molecules23020358
Yalage Don, S. M., Schmidtke, L. M., Gambetta, J. M., and Steel, C. C. (2020). Aureobasidium pullulans volatilome identified by a novel, quantitative approach employing SPME-GC-MS, suppressed Botrytis cinerea and Alternaria alternata in vitro. Sci. Rep. 10, 1–13. doi: 10.1038/s41598-020-61471-8
Yang, M., Lu, L., Pang, J., Hu, Y., Guo, Q., Li, Z., et al. (2019). Biocontrol activity of volatile organic compounds from Streptomyces alboflavus TD-1 against Aspergillus flavus growth and aflatoxin production. J. Microbiol. 57, 396–404. doi: 10.1007/s12275-019-8517-9
Ye, X., Chen, Y., Ma, S., Yuan, T., Wu, Y., Li, Y., et al. (2020). Biocidal effects of volatile organic compounds produced by the myxobacterium Corrallococcus sp. EGB against fungal phytopathogens. Food Microbiol. 91:103502. doi: 10.1016/j.fm.2020.103502
Yuan, J., Raza, W., Shen, Q., and Huang, Q. (2012). Antifungal activity of bacillus amyloliquefaciens NJN-6 volatile compounds against Fusarium oxysporum f. sp. cubense. Appl. Environ. Microbiol. 78, 5942–5944. doi: 10.1128/AEM.01357-12
Zhang, X., Li, B., Wang, Y., Guo, Q., Lu, X., Li, S., et al. (2013b). Lipopeptides, a novel protein, and volatile compounds contribute to the antifungal activity of the biocontrol agent Bacillus atrophaeus CAB-1. Appl. Microbiol. Biotechnol. 97, 9525–9534. doi: 10.1007/s00253-013-5198-x
Zhang, B. Y., Samapundo, S., Pothakos, V., Sürengil, G., and Devlieghere, F. (2013a). Effect of high oxygen and high carbon dioxide atmosphere packaging on the microbial spoilage and shelf-life of fresh-cut honeydew melon. Int. J. Food Microbiol. 166, 378–390. doi: 10.1016/j.ijfoodmicro.2013.08.002
Zhang, J., Xie, J., Zhou, Y., Deng, L., Yao, S., and Zeng, K. (2017). Inhibitory effect of Pichia membranaefaciens and Kloeckera apiculata against Monilinia fructicola and their biocontrol ability of brown rot in postharvest plum. Biol. Control 114, 51–58. doi: 10.1016/j.biocontrol.2017.07.013
Zhao, L. J., Yang, X. N., Li, X. Y., Mu, W., and Liu, F. (2011). Antifungal, insecticidal and herbicidal properties of volatile components from Paenibacillus polymyxa strain BMP-11. Agric. Sci. China 10, 728–736. doi: 10.1016/S1671-2927(11)60056-4
Zheng, L., Situ, J. J., Zhu, Q. F., Xi, P. G., Zheng, Y., Liu, H. X., et al. (2019). Dataset on collecting volatile compounds produced by three bacteria and testing their efficacy against the pathogen Peronophythora litchii. Data Br. 25:104345. doi: 10.1016/j.dib.2019.104345
Zhou, M., Li, P., Wu, S., Zhao, P., and Gao, H. (2019). Bacillus subtilis CF-3 volatile organic compounds inhibit Monilinia fructicola growth in peach fruit. Front. Microbiol. 10:1804. doi: 10.3389/fmicb.2019.01804
Keywords: microbial volatile organic compounds, sustainability, phytopathogens, bioactive compounds, biotechnology, biological control
Citation: Almeida OAC, de Araujo NO, Dias BHS, de Sant’Anna Freitas C, Coerini LF, Ryu C-M and de Castro Oliveira JV (2022) The power of the smallest: The inhibitory activity of microbial volatile organic compounds against phytopathogens. Front. Microbiol. 13:951130. doi: 10.3389/fmicb.2022.951130
Edited by:
Luciano Beneduce, University of Foggia, ItalyReviewed by:
Valentina Lazazzara, Fondazione Edmund Mach, ItalyMiguel Portillo-Estrada, University of Antwerp, Belgium
Copyright © 2022 Almeida, de Araujo, Dias, de Sant’Anna Freitas, Coerini, Ryu and de Castro Oliveira. This is an open-access article distributed under the terms of the Creative Commons Attribution License (CC BY). The use, distribution or reproduction in other forums is permitted, provided the original author(s) and the copyright owner(s) are credited and that the original publication in this journal is cited, in accordance with accepted academic practice. No use, distribution or reproduction is permitted which does not comply with these terms.
*Correspondence: Juliana Velasco de Castro Oliveira, juliana.velasco@lnbr.cnpem.br