- 1Université de Reims Champagne Ardenne, RIBP EA4707 USC INRAE 1488, SFR Condorcet FR CNRS 3417, Reims, France
- 2School of Plant and Environmental Sciences, Virginia Polytechnic Institute and State University, Saunders Hall, Blacksburg, VA, United States
Plasmopara viticola (P. viticola, Berk. & M. A. Curtis; Berl. & De Toni) causing grapevine downy mildew is one of the most damaging pathogens to viticulture worldwide. Since its recognition in the middle of nineteenth century, this disease has spread from America to Europe and then to all grapevine-growing countries, leading to significant economic losses due to the lack of efficient disease control. In 1885 copper was found to suppress many pathogens, and is still the most effective way to control downy mildews. During the twentieth century, contact and penetrating single-site fungicides have been developed for use against plant pathogens including downy mildews, but wide application has led to the appearance of pathogenic strains resistant to these treatments. Additionally, due to the negative environmental impact of chemical pesticides, the European Union restricted their use, triggering a rush to develop alternative tools such as resistant cultivars breeding, creation of new active ingredients, search for natural products and biocontrol agents that can be applied alone or in combination to kill the pathogen or mitigate its effect. This review summarizes data about the history, distribution, epidemiology, taxonomy, morphology, reproduction and infection mechanisms, symptoms, host-pathogen interactions, host resistance and control of the P. viticola, with a focus on sustainable methods, especially the use of biocontrol agents.
Downy Mildew in Agricultural Systems
Downy mildews are primarily foliage blights caused by obligate biotrophic plant parasites which result in considerable economic losses on numerous crops. Plant diseases caused by oomycetes are separated into two types: those affecting plant parts in, or in contact with, the soil and those affecting aerial plant parts. Downy mildews infect young, tender green leaf, twig, and fruit tissues, causing severe losses in short periods of time. These infections can destroy 40–90% of plants in the field in optimal humidity and temperature (Toffolatti et al., 2018).
Downy mildews have caused spectacular and catastrophic epidemics on crops in the past, and many of them are still difficult to control despite the discovery of systemic fungicides which improved the ability to control fungal diseases. The grapevine downy mildew decimated the European viticulture and wine industry in the late 1800s. It had been endemic within North America, where native grapevine species developed fungal resistance. In 1878 it was introduced to southwest France through imported American grapevine rootstocks resistant to the phylloxera (Gessler et al., 2011). Within 5 years of its detection by Jules Emile Planchon, P. viticola had spread to all French, Italian, German and Swiss vineyards (Planchon, 1887). Grapevine downy mildew remained out of control until Pierre-Marie Alexis Millardet discovered the antifungal properties of copper sulfate in 1882 and invented the Bordeaux mixture in 1885 (Millardet, 1885; Gessler et al., 2011). Downy mildews have significant economic impact, as represented in Figure 1, namely grapes (54% of the total host range), cucurbits (12%), lettuce (8%), leek and onion (6%), tobacco (4%), peas (3%), brassicas (3%), sugar beet (3%), soybeans (2%), corn (2%), hops (1%), and sunflower (1%) (Gisi and Sierotzki, 2008).
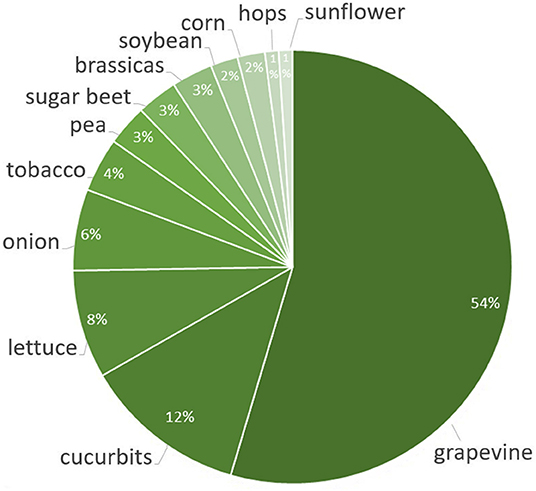
Figure 1. The most popular hosts of downy mildews. The color density corresponds to the rate of the crop damaged upon the total host range.
Together with other widespread eukaryotes, namely diatoms and seaweeds, the fungal-like oomycetes belong to the kingdom Chromista (Stramenopila). They have mycelium containing glucans and cellulose, however, they have no cross walls excluding distinct living (cytoplasmic) hyphal portions from older portions from which the cytoplasm has been withdrawn. The phylum Oomycota includes several significant plant pathogens of economically important crop plants including necrotrophs in Pythium (Pythium ultimum), obligate biotrophs responsible for downy mildew (Hyaloperonospora arabidopsidis, P. viticola) and hemibiotrophs in Phytophthora (P. sojae, P. capsica, P. infestans) (Kamoun et al., 2015). Oomycetes belong to two orders, explicitly Saprolegniales and Peronosporales. The order Peronosporales includes mainly plant parasitic species a few of which are among the most economically impactful plants pathogens. More than 700 species within this group are downy mildew pathogens on a wide range of mono- and dicotyledonous plants (Thines and Choi, 2016). The most common oomycetes and downy mildews that they cause are listed in Table 1.
Epidemics of Downy Mildews
The importance of downy mildews in agriculture has increased within the past 50 years, carried by the significant intercontinental trade and movement in grain, roots, and fruits. Downy mildew is one of the most damaging foliar diseases of grapevine, leading to organoleptic defects, productivity reduction and significant yield losses up to 75% in humid grapevine-producing areas worldwide (Darriet et al., 2002; Stummer et al., 2005; Jermini et al., 2010b; Gessler et al., 2011).
The importance of downy mildews in agriculture has increased within the past 50 years, carried by the significant intercontinental trade and movement in grain, roots, and fruits. After introduction from North America, this disease was a huge problem for European viticulture, causing epidemics in years with high humidity in the absence of sufficient control.
From 1907 to 1916, downy mildew reduced output of German vineyards by 33%, while significant periodic losses occurred in Italy in 1889, 1890, 1903, 1910, 1928, 1933 and 1934 (Müller, 1938). In 1915, 70% of the French grapevine production was destroyed by this pathogen. In 1930, 20 million liters of wine were lost in France (Cadoret, 1931).
In 1960, an epidemic of tobacco downy mildew spread to 11 countries and led to loss of 30% tobacco plants worldwide at a cost of $25 million. In the 1960s outbreaks occurred in Mexico, Israel, and the USA; in Texas alone, losses in 1969 reached $2.5 million with field incidence of disease up to 90%. In Taiwan several epidemics of sugarcane downy mildew have occurred from 1960 to 1964, leading to crop reduction of 70% (Payak, 1975).
In 1979, a devastating epidemic of this pathogen spread rapidly from Florida to Northeastern America and Canada causing losses to growers $200 million. In Cuba, the disease caused severe losses from 1978 to 1980 (Perez et al., 2003; Borrás-Hidalgo et al., 2010). Epidemics of Philippine downy mildew in 1974–1975 on maize and sugarcane cost the nation 8% of the total yield, leading to economic losses of $22.6 million (Exconde, 1974, 1976).
The first epidemics of cucurbits downy mildew occurred in 1984 on cucumbers and melon in Czechoslovakia. Damage was limited because of its appearance late in the growing season. In 1985 cucumber crops all over the Europe were heavily affected. The effect in Czechoslovakia was particularly devastating, with loss of 80–90% in cucumber yields. From 1986 to 1988, due to improved plant protection, economic losses caused by epidemics were lower, despite the high infection level. In 1989, however, losses in Czechoslovakia once again soared, reaching 80%.
African epidemics of sorghum and maize downy mildew during 1977, 1989, 1992, 1993, and 1995 led to crop losses ranging between 10 and 100% (Jeger et al., 1998). The sterility of systemically infected maize plants caused severe economic impact such as in Venezuela in the early 1970s (Frederiksen and Renfro, 1977). During the Indian epidemic in 1975, a harvest of 100,000 tons was discarded. Southern Nigeria was affected by maize downy mildew, where it caused losses of up to 15,000 tons and 12% of yield in 1991 and 1992.
In the decade since 2009, outbreaks of downy mildew in Central Europe have caused serious damage to hosts such as pumpkin, melon, watermelon, and Lagenaria, probably due to genetic changes in a virulence of P. cubensis (Lebeda, 1986; Lebeda and Schwinn, 1994; Cohen et al., 2015).
Grapevine downy mildew, caused by P. viticola, remains the most destructive downy mildew in Europe and the eastern half of the USA causing significant losses in grape production. The optimal temperature for P. viticola growth is 25°C; however rain is the main factor responsible for the epidemics. Downy mildew is one of the most damaging foliar diseases of grapevine, leading to organoleptic defects, productivity reduction and significant yield losses up to 75% in humid grapevine-producing areas worldwide (Darriet et al., 2002; Stummer et al., 2005; Jermini et al., 2010b; Gessler et al., 2011). After introduction from North America, this disease was a huge problem for European viticulture, causing epidemics in years with high humidity in the absence of sufficient control. In 1915, 70% of the French grapevine production was destroyed by this pathogen. In 1930, 20 million liters of wine were lost in France (Cadoret, 1931). From 1907 to 1916, downy mildew reduced output of German vineyards by 33%, while significant periodic losses occurred in Italy in 1889, 1890, 1903, 1910, 1928, 1933, and 1934 (Müller, 1938).
The growth of P. viticola is restricted in regions with low rainfall in the spring and summer and in northern regions, where sufficiently high temperatures are not reached in the spring. The downy mildew infects all green parts of the grapevine, including leaves, young stems and grapes, but leaves were found to be the main source of spores due to size of their surface, volume of cells for haustorial growth, stomatal amount and structure and the lack of protection against invasion of the pathogen. The disease is characterized by oily patches on the upper face of leaves that develop a dull green or yellowish color while the lower face exhibits a white growth. Infection of P. viticola causes leaf discoloration, necrosis and defoliation, which together reduce nutrient composition, sugar accumulation in berries, capacity for buds overwintering and harvest amount (Underdown et al., 2008). When infected, young berries become brown and are covered by white powder resulting from sporulation. As berries ripen, they become less susceptible to the infection, but rachis infections can spread into older berries (Gessler et al., 2011).
Rotting with subsequent mummification of inflorescences, shoots, clusters, and berries are also present if control measures are ineffective.
In this review, bibliographic data were extracted from the SCOPUS database (https://www.scopus.com/) using specific keywords “Plasmopara viticola” or “P. viticola” and “downy mildew” and “grapevine,” from which 1649 documents were obtained. The bibliometric analysis was constructed using different bibliometric indices, including the most specific keywords used in the published literature related to downy mildew, countries, and the top journals. For the network construction, bibliometric analysis was constructed using the VOSviewer processing software (v1.6.9., Leiden University, Leiden, The Netherlands). The network analysis shows the worldwide distribution of related articles to downy mildew which help to highlight the relationships between countries, authors, and keywords found to get a comprehensive perspective of the current research of this area (Figure 2A). The analysis illustrated that most research conducted on downy mildew was demonstrated in the United States, China, France, Germany, Switzerland, and Italy (Figure 2B). It was noted that most articles addressed induced disease resistance, epidemiology, and control (Figure 2A).
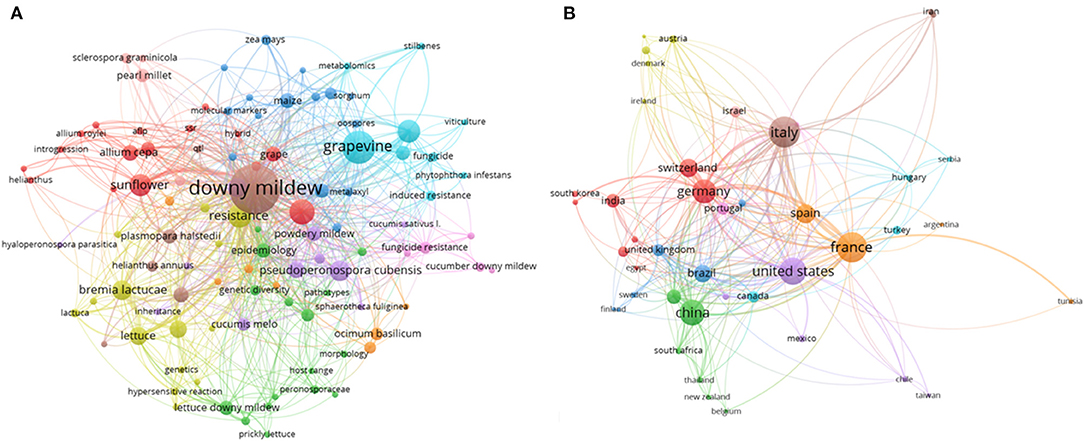
Figure 2. (A) Bibliometric analysis of the specific keywords used in the published literature related to downy mildew. Bibliographic data were extracted from the SCOPUS database using specific keywords “Plasmopara viticola” or “P. viticola” and “downy mildew” and “grapevine,” and the analysis include different bibliometric indices such as the most popular keywords, countries, and the top journals. For the network construction we used the VOSviewer processing software (https://www.vosviewer.com/ v1.6.9., Leiden University, Leiden, The Netherlands). (B) Publimetry related to downy mildew across the world. The network analysis, constructed using the VOSviewer software, includes the most popular countries.
Taxonomic Evolution of Downy Mildew
The downy mildews are similar to fungi in many ways, however, they do not constitute part of a monophyletic development of fungi within the eukaryote domain (Money, 1998). Downy mildews (Peronosporaceae) are a morphologically diverse group of oomycetes, mainly united by obligate parasitism in combination with presence of conidio- or sporangiophores with determinate growth. This group belongs to the orders Peronosporales and Sclerosporales in the class Peronosporomycetes, within the kingdom Straminipila, mainly based on morphological features of conidia/sporangia such as branching of conidio-/sporangiophores or shape of terminal branches (Figure 3) (Dick, 2002; Lebeda et al., 2008; Spencer-Phillips et al., 2016).
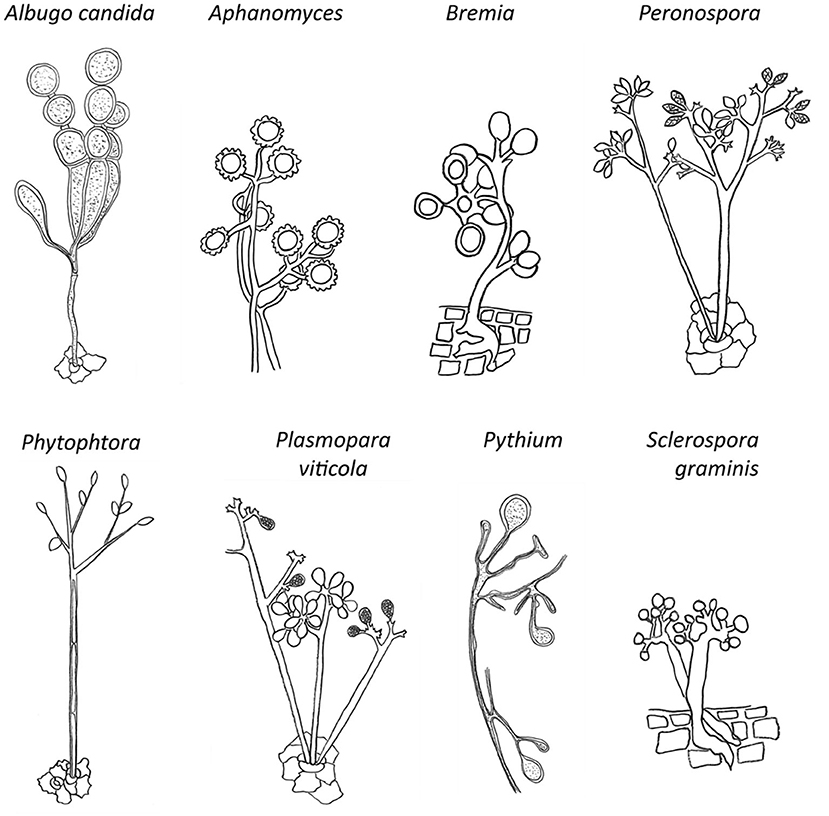
Figure 3. The most popular oomycetes that cause disease in plants. Morphological structures represented include length, branching and septation of conidio- or sporangiophores; conidial/sporangial size, shape, position, amount, and type of connection.
Varying approaches to classification have resulted in highly different numbers of accepted species depending on the criteria used, probably caused by the narrow host ranges because of an obligate parasitism, which represent genetically distinct species. In contrast, when morphology was used as a primary criterion for species definition, only few genetically heterogeneous species were defined (Hall, 1996). Morphological species concept, or Morphometric concept, is based on morphological characteristics such as size, shape, color of spores, oogonia and antheridia, the degree, kind of branching and length of sporangiophores (Figure 3). Using this phenetic approach, Oomycetes were divided into 3 subclasses: Saprolegniomycetidae, Rhipidiomycetidae, and Peronosporomycetidae, the latter including downy mildews (Dick et al., 1984; Dick, 1995).
With the availability of molecular phylogenies this subdivision of oomycetes was later confirmed (Dick et al., 1999; Riethmüller et al., 2002). Using this approach, Constantinescu showed that several Asteraceae pathogens, previously related to Peronospora, had in fact consistently different morphology and were placed in a new genus Paraperonospora (Constantinescu, 1989). In spite of an importance of morphological characteristics in classification, there is evidence that many characteristics of downy mildews can vary in the same species depending on the host, environmental conditions, nutrient media and reproductive features such as presence of heterothallism (Michelmore, 1981; Gustavsson, 1987; Johnson, 1989).
The Eco-physio-phenetic concept, introduced by Skalický, combined an ecophysiological (biological) criterion of host specialization with morphological properties. Several species of Peronospora on the host family Rosaceae were delimited based on their host subfamilies, morphology of conidiophores and conidial size: Peronospora rubi on Rubus was separated from Peronospora sparsa on Rosa, and Peronospora potentillae was separated from Peronospora potentillae-reptantis. Like in a biological species concept, Skalický did not control cross-infection experiments, so presence of host differences that had been used for species delimitation, was not proved (Skalický, 1983). Based on this approach, downy mildews have been divided into two sections, the Graminicolous and Eudicot-infecting downy mildews, based on mycelial characters, haustorial shapes and conidiophore morphology (Dick et al., 1984).
In 1992 Barr and Désaulniers conducted an ultrastructural analysis of zoospores applicable to systematics. They found a difference of zoospore flagellar apparatuses between Phytophthora infestans, Phytophthora mirabilis, and other Phytophthora spp., suggesting the presence of 2 groups, phylogenetically distinct from other Phytophthora species in a Pythiaceae family (Barr and Désaulniers, 1992). Ultrastructure of zoospores was firstly examined by transmission electron microscopy (TEM) in Sclerospora graminicola, where discharge papilla (a significant feature for identification, for example, a Peronosporales order) was found to take a part in zoosporogenesis (Lange et al., 1984). Surface ornamentation of conidia was described using scanning electron microscopy (SEM) on Peronospora sordida and Peronospora statices, where it was a distinguishing trait in addition to conidial lengths and breadths (Hall and Humphreys-Jones, 1989). A study using the SEM of three Basidiophora species demonstrated the occurrence of species-specific sporangial ornamentation, sporangiophore detachment scars and sporangiophore pedicels in B. entospora and B. kellermannii (Barreto and Dick, 1991). In 2004 Spring and Thines discovered additional ultrastructural features by SEM: using a model of downy mildews, they found classical features like conidio-/sporangiophore branching or sporangial germination are not suitable, but structure of the ultimate branchlets and haustorial shape were approved to be phylogenetically informative (Spring and Thines, 2004). Different haustorial types in downy mildews were described as far back as in 1956, but they were neglected for classification until 2000, when they started to be used as a diagnostic for several lineages as Hyaloperonospora, Pseudoperonospora, Plasmopara, and Bremia (Fraymouth, 1956; Constantinescu and Fatehi, 2002; Riethmüller et al., 2002).
A few biochemical characteristics such as fatty acids composition also were used for classification of downy mildews. Spring and Haas justified distinction of the oomycetes from true fungi and suggested that fatty acid composition together with the host specificity could be a promising diagnostic trait for species classification in obligate biotrophs in absence of clear morphological differences (Spring and Haas, 2002).
With the advent of molecular systematics, use of only morphological features for classification was deemed to be too simplistic. Molecular phylogenetic investigations have enabled the evaluation of the species problem using new perspectives and have led to the shift from a morphological to a phylogenetic species concept. In the absence of stable and correct morphological characteristics, this concept is increasingly based on molecular evidence of reproductive isolation, a general tendency within the mycology in recent decades. It became possible to recognize morphologically similar cryptic species as distinct ones if reproductive isolation and genetic distinctness can be demonstrated. However, molecular evaluation of boundaries between species requires use of several molecular markers and thorough sampling in all the distribution area which is difficult especially for biotrophic oomycetes (Lebeda et al., 2008). In published works, the most popular technique was widely used ribosomal DNA (rDNA) internal transcribed spacer region (ITS) sequencing, probably because of its simplicity and high variability compared with other genic regions of rDNA like small or large-subunit rRNA. ITS length was found to be a potential marker for species differentiation: its size originates from repetitive elements, number, and length of which seems to be taxon-specific (Voglmayr, 2008).
Riethmüller et al. analyzed nuclear large subunit ribosomal DNA sequences of the Peronosporomycetes to investigate their phylogenetic relationships (Riethmüller et al., 2002). During their study, basal division of Peronosporomycetes into Peronosporomycetidae and Saprolegniomycetidae, as proposed before, was confirmed. Also, division between the Pythiales and Peronosporales on the one hand and the Saprolegniales, Leptomitales, and Rhipidiales on the other was well-supported as well as the placement of orders Saprolegniales and Leptomitales in the Saprolegniomycetidae. The Sclerosporales, Peronosporomycetidae, Pythiales and Peronosporales were found to be polyphyletic. The Verrucalvaceae were merged within the Saprolegniales; Peronophythora was merged with Phytophthora; Bremiella was transferred to Plasmopara; and Phytophthora was found to be closer related to Peronosporaceae than to Pythiales, which was confirmed in later studies (Dick et al., 1984; Riethmüller et al., 1999, 2002; Cooke et al., 2000; Hallin et al., 2018). Monophyly of the genera Pseudoperonospora and Hyaloperonospora were supported (Voglmayr, 2003). The classification of the graminicolous downy mildews such as Sclerospora and Peronosclerospora using molecular phylogenetic data did not support this group as a separate family and order or classification within the Saprolegniomycetidae, so Graminicolous downy mildews were placed within Peronosporaceae (Riethmüller et al., 2002; Göker et al., 2003, 2007). Genera Hyaloperonospora and Perofascia were segregated from the large genus Peronospora; owing to their polyphyly, three species of Bremiella genus were embedded within Plasmopara (Constantinescu and Fatehi, 2002; Göker et al., 2007; Thines and Choi, 2016). Based on phylogenetic analyses, genus Rhysotheca, introduced by Wilson in 1907 as closely related to Peronospola viticola, was merged with P. viticola (Wilson, 1907; Riethmüller et al., 2002).
To obtain insight into the phylogenetic relationships within the different genera of Peronosporaceae family, the large subunit of ribosomal RNA (LSU) gene sequences of the different genera of this family were retrieved from the National Center for Biotechnology Information database (NCBI) (http://www.ncbi.nlm.nih.gov) and aligned to construct the phylogenetic tree (Figure 4). The tree was built using MEGA version X with neighbor-joining method. The dataset was boot-strapped 1,000 times and values at nodes indicate bootstrap values out of 1,000 resampling.
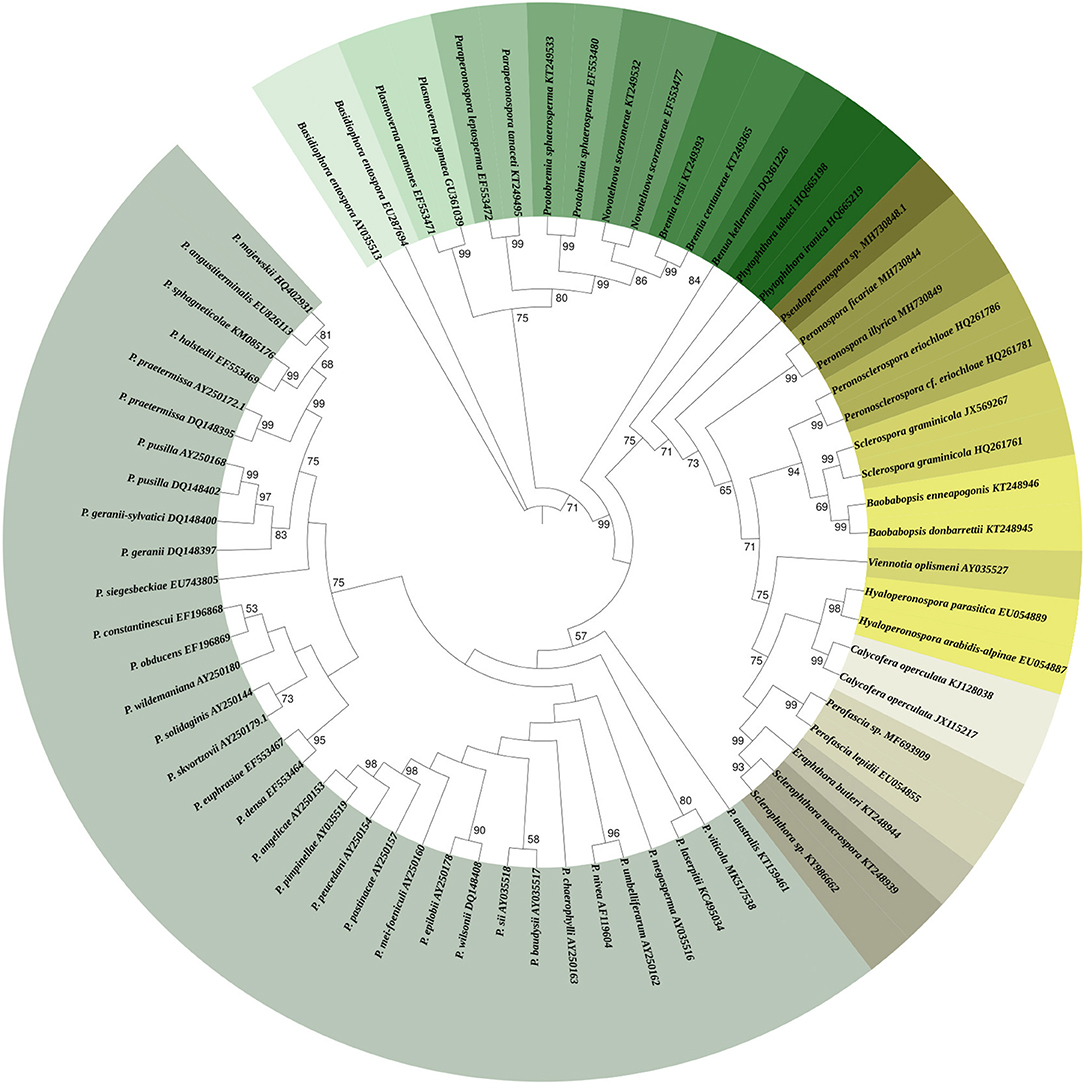
Figure 4. Phylogenetic tree represents different genera of Peronosporaceae family. For phylogenetic tree analysis, a large subunit of ribosomal RNA (LSU) gene sequences of the different genera of Peronosporaceae family were retrieved from the National Center for Biotechnology Information database (NCBI) (http://www.ncbi.nlm.nih.gov). Collected sequences were aligned to construct the phylogenetic tree. The tree was built using MEGA version X with neighbor-joining method. The dataset was boot-strapped 1,000 times. Values at nodes indicate bootstrap values out of 1,000 resampling.
Plasmopara was proved be a polyphyletic genus, so several Plasmopara and Bremia pathogens of Poaceae, Cichorieae, Ranunculaceae, and Scorzonera hosts were segregated into new genera Viennotia, Poakatesthia, Graminivora, Protobremia, Plasmoverna, and Novotelnova. Plasmopara euphrasiae was segregated from Plasmopara densa and Plasmopara centaureae-mollis was revised and synonymised with Bremia centaureae (Riethmüller et al., 2002; Göker et al., 2003; Voglmayr et al., 2004; Thines et al., 2006, 2007; Voglmayr and Constantinescu, 2008). Based on differences in partial sequence analysis of the nuclear ITS region, Spring et al. succeeded in differentiating between certain pathotypes of Plasmopara halstedii originating from different geographic regions (Spring and Zipper, 2006). Molecular studies of Pseudoperonospora proved the conspecificity of species from different hosts: Pseudoperonospora humuli was synonymized with Pseudoperonospora cubensis based on identity of ITS sequences and morphology of both pathogens; later multiple host shifts from Cannabinaceae to Cucurbitaceae confirmed the conspecificity (Gregory, 1915; Choi et al., 2005). In the current review, a phylogenetic tree (Figure 5) harboring the different validated members of Plasmopara genus was constructed using the large subunit of ribosomal RNA (LSU) gene sequences. The tree was built using he tree was built using MEGA version X and the dataset was boot-strapped 1,000 times. Values at nodes indicate bootstrap values out of 1,000 resampling.
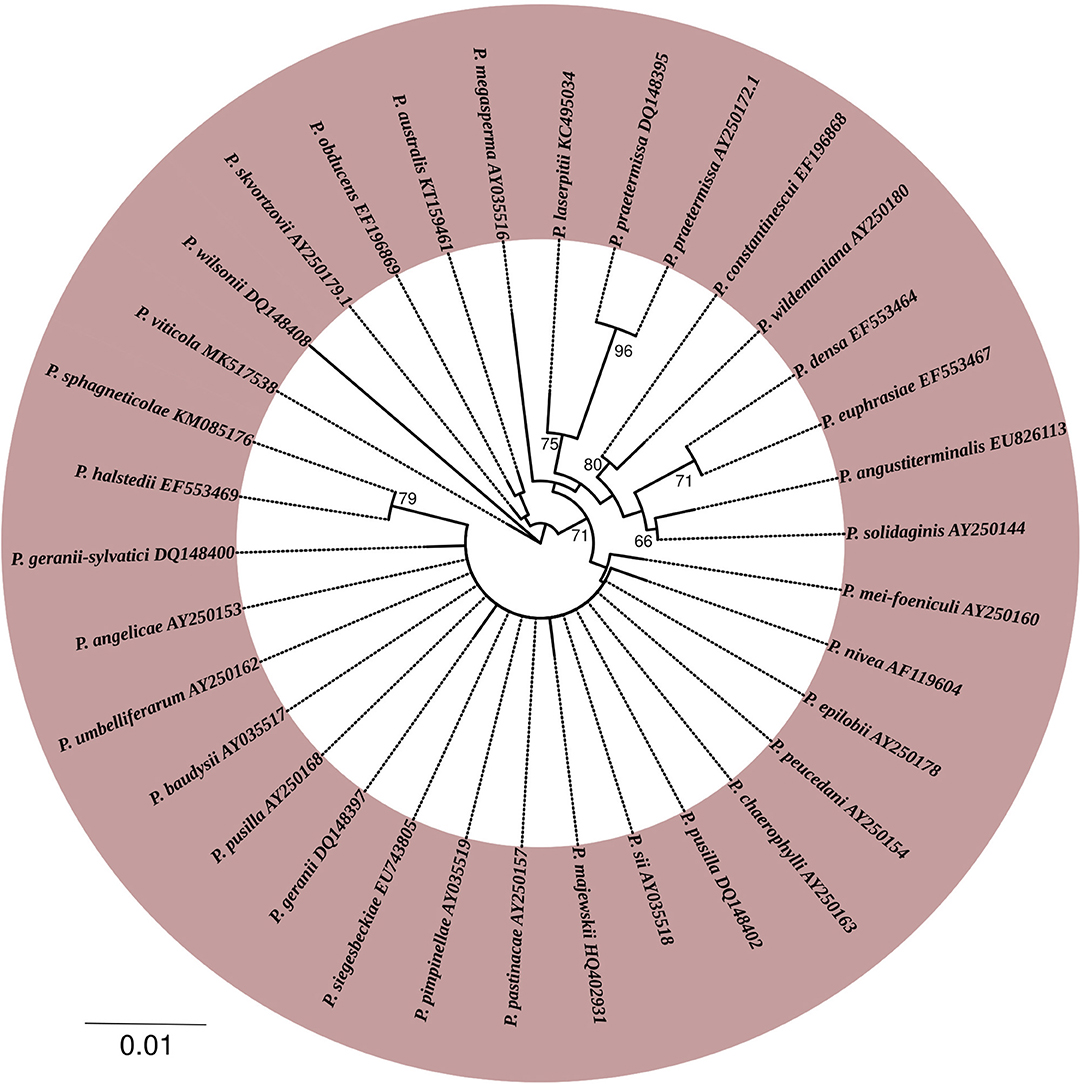
Figure 5. Phylogenetic tree represents different members of Plasmopara genus. The tree was built with MEGA version X using the large subunit of ribosomal RNA (LSU) gene sequences of the different members of Plasmopara genus. Values at nodes indicate bootstrap values out of 1,000 resampling.
Molecular phylogenetic analyses showed a close phylogenetic relationship of downy mildews to the genus Phytophthora, suggesting an origin as a stem from different Phytophthora groups (Riethmüller et al., 1999; Cooke et al., 2000; Voglmayr, 2003). Later in a multigene (five genes) study Göker et al. found downy mildews to be monophyletic (Göker et al., 2007). Analyzing this five gene dataset by different methods of phylogenetic reconstruction, Göker found indications downy mildews are not monophyletic, and one of Phytophthora group was embedded within the downy mildews clade (Göker and Stamatakis, 2006). The additional data from other gene regions and taxa seems to be necessary to achieve a correct phylogeny.
Plasmopara Viticola
Taxonomy
Genealogies in P. viticola, an oomycete endemic to North America on wild and cultivated grapevines, have suggested the existence of four cryptic species, with different levels of host plant specialization varying from mild to complete host dependence. P. viticola (Berk. & Curt.) Berl. & de Toni, belonging to the order of Peronosporales and the family of Peronosporaceae was firstly collected by American botanist and mycologist Lewis David de Schweinitz in 1834 and it was classified as Botrytis cana (Schweinitz, 1834). In 1848 Henry William Ravenel in co-authorship with Miles Joseph Berkeley, re-classified B. cana as Botrytis viticola (Hendrickx, 1948). The German microbiologist Anton De Bary described in 1863 its both reproduction cycles and placed it in a new genus Peronospora as Peronospora viticola (De Bary, 1863). Then, Schröder found in 1886 differences between several Peronospora members and introduced a new genus of Plasmopara (Schröter, 1886). In 1888 Berlese and de Toni, using Schröder's classification system, gave this pathogen its current name of Plasmopara viticola (Berlese and De Toni, 1888).
Morphology
Grapevine downy mildew, like many oomycetes, possess a filamentous vegetative body called a mycelium that is formed by dichotomously branching hyphae, which vary in diameter from 1 to 60 micrometers depending on a development stage of the pathogen. They grow in intercellular space of a plant in a coenocytic manner, i.e., they contain several nuclei without the formation of cross-walls that divide each hypha into separate cells in septate fungi. Haustoria project from the hypha to uptake nutrients from the host cells. Cell walls of oomycetes consists of cellulose, glucans, small amounts of chitin and hydroxyproline, differing from the true fungi (Bartnicki-Garcia, 1968; Werner et al., 1968; Bakshi et al., 2001). Cell wall-degrading enzymes are important in pathogen degradation; they are synthesized by the host plant specifically to target and break down cell wall components.
Mycelium produces sporangiophores that arise through stoma and produce lemon-shaped sporangia at their tips. This branching is perpendicular and monopodial, which means that the growth of the main sporangiophore branch continues while the lateral remains fixed, until 4–6 branches with 2 or 3 secondary branches are formed. Ultimate branches called sterigmata are mostly trichotomous in Plasmopara genus and have distinct annuli at their tips. Sporangia are sacs that release moving thin walled ellipsoid zoospores that are 15–30 μm in diameter and have two heterokont flagella. The anterior flagellum is longer and possesses 2 symmetric rows of tubular, tripartite hairs called mastigonemes, while posterior one is shorter and smooth (whiplash). Oomycetes also produce large, 25–50 μm in diameter, thick-walled spherical sexual spores called oospores.
P. viticola Genome
Genome Architecture
To further understand the P. viticola-host interactions at the genome level, Dussert and collaborators sequenced the genome of P. viticola strain INRA_Pvit_2, obtaining a genome size of 92.9 Mb assembled into 358 scaffolds, using PacBio long-read technology (Dussert et al., 2016). Other isolates including JL-7-2 and PvitFEM01 have been sequenced and recorded online in the NCBI (Table 2). The genomes of different strains were sequenced using the methods summarized in Table 2; their sizes span from 83 to 101 Mb. The genome characteristics as well as the project information of different isolates of P. viticola are presented in Table 2.
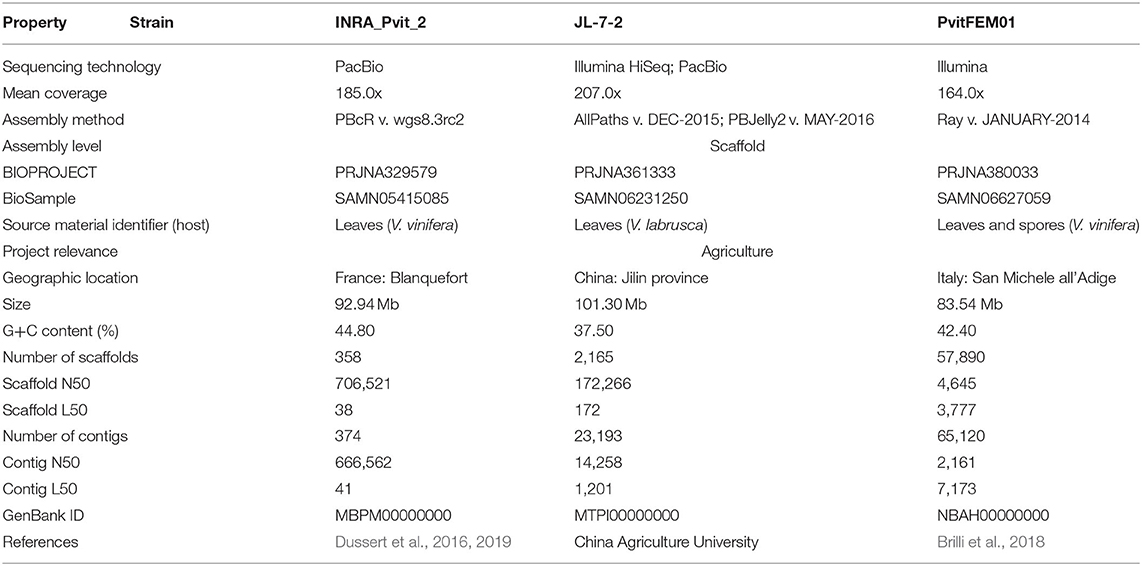
Table 2. Project information and genomic features of draft genome sequences of different isolates of P. viticola.
P. viticola Effectors
Numerous plant pathogens may release effector proteins to overcome the plant's immune defense response. The function of effector proteins in the pathogenic process has been widely dissected in bacteria. Effectors may have a crucial function at the first encounter between the plant and the pathogen to develop a compatible interaction. Oomycetes use an array of effectors translocated in intra- and extracellular plant tissues to modulate the plant metabolism to their benefit and promote infection. The ability to liberate effector proteins, which may penetrate plant cells and control host processes, is a central determinant of a host–pathogen interaction outcome (Casagrande et al., 2011). As effectors are produced by the pathogen, they elicit a host-translocation signal recognized by the host cells. Two primary classes of host translocated effectors have been identified: cytoplasmic RXLR targeting subcellular plant compartments and apoplastic LFLAK, secreted into the plant extracellular space. During independent genome sequencing of such pathogens as Phytophthora, Pythium, Hyaloperonospora arabidopsidis and P. viticola, several 100 intra- and extracellular effectors have been found (Rehmany et al., 2005; Haas et al., 2009; Mestre et al., 2012; Perazzolli et al., 2012; Chen et al., 2021).
RXLR effectors are the most studied group of oomycotic effectors; they are small AVR proteins with conserved N-terminal amino acid motifs consisting of arginine, any amino acid-leucine and leucine. Evidence points to the potential for RXLR effectors to drive plant cell death following recognition by the corresponding R proteins or unknown mechanisms (Huang et al., 2019). Many of RXLR effectors have a second EER-motif (glutamic acid-glutamic acid-arginine optional amino acid motif) participating in the transport from the pathogen to its host, and at varying distance C-terminal to RXLR motif (Stassen and Van Den Ackerveken, 2011; Liu et al., 2021). Both RXLR and EER motifs are needed to access host cells (Kale and Tyler, 2011). However, even while the RXLR motif was reported as responsible for effectors entering the host cell, its biological role remained controversial (Ellis and Dodds, 2011; Wawra et al., 2013, 2017). Enzyme inhibitors, cysteine-rich proteins and Nep1-like proteins are located in a plant apoplast and interact with surface receptors and extracellular host targets (Kamoun, 2006; Askani et al., 2021). Another group of cytoplasmic effectors include crinkling and necrosis-inducing families (CRN) that are general for many oomycete members, in a contrast to RXLR that are specific to Phytophthora and species of downy mildews (Anderson et al., 2015; Lan et al., 2019). CRN encodes proteins that are characterized by a conserved N-terminal LXLFLAK motif, a recombination site motif HVLVVVP (DWL domain) and diverse C-terminal effector domains (Yin et al., 2017; Xiang et al., 2021).
During the whole genome sequence of the Chinese P. viticola isolate JL-7-2, 100 RXLR effectors (PvRXLR) and 90 CRN effectors had been identified; 18 of these PvRXLRs were common with those obtained after the transcriptome sequencing of European P. viticola isolate containing 45 RXLR effectors (Mestre et al., 2016; Yin et al., 2017).
In a construction of a cDNA library from zoospores of P. viticola, there were 45 expressed sequence tags (EST) potentially encoding hydrolytic enzymes, protein inhibitors, elicitor-like proteins, and RXLR—fungal effectors responsible for its virulence (Mestre et al., 2012). During RNA-Seq analysis of cDNAs in a study of genes involved in a pathogenicity of Australian strain P. viticola CSIRO-L-2 and two Chinese strains P. viticola JL-7-2 and P. viticola ZJ-1-1, total 10 CRN and 51 RxLR (PvRXLR) effectors were identified according to the presence of a secretory signal sequence and an RxLR/CRN motif; all the PvRXLRs were responsible for the suppression of programmed cell death. In addition to that, putative apoplastic effectors were found using Pfam domain analysis; most were identified as glycosyl hydrolases, peptidases, and protease inhibitors (Yin et al., 2015).
The pathosystem between Italian strain of P. viticola and V. vinifera identified RxLR and CRN genes. In parallel, several RxLR protein effectors were found to be triggering an immune response to the infection of resistant Vitis riparia (Brilli et al., 2018). While several effectors of P. viticola have been recently studied, little data is available on the function of these molecules in pathogenicity (Li et al., 2015; Yin et al., 2015; Xiang et al., 2016; Brilli et al., 2018). The possible involvement of RxLR effectors on the virulence of specific strains has been further deciphered to elucidate the specific function of these genes in a successful infection on tolerant hosts (Gómez-Zeledón and Spring, 2018). In addition, Brilli et al. (2018) reported that RxLR_PVITv1008311Δsp triggers a hypersensitive response in V. riparia but not in V. vinifera, suggesting that European-grown grapevine cultivars have lost or perhaps have not yet evolved effector recognition of P. viticola to trigger defense responses.
Within the genome sequencing of P. viticola isolate INRA-PV221 (Dussert et al., 2019), found 1592 genes that encode proteins concerned with plant-pathogen interactions, while 317 effectors were identified as cytoplasmic RxLRs and several as CRNs. Aside from the two main effector classes, which represent the two dominant classes of secreted effectors, a certain range of other secreted protein families were also found to be encoded in the P. viticola genome, namely proteases, glycoside hydrolases, elicitins and elicitin-like proteins, as well as cell wall degrading enzymes, notably pectin lyases, pectin esterases, and phospholipases. Putative carbohydrate-active enzymes CAZymes have also been reported as pathogenicity factors in plant pathogens including oomycetes. Among them, 15 families of glycoside hydrolases, 6 families of carbohydrate esterases, 6 families of carbohydrate binding modules, 4 families of ancillary activities, 3 families of glycosyltransferases, and a polysaccharide lyase (Yin et al., 2017). While the number of CAZymes in the P. viticola secretome is lower than in Phytophthora species, the glycoside hydrolases family is the most abundant, suggesting that the breakdown of the plant cell wall is an integral step in the successful colonization of the pathogen (Brouwer et al., 2014; Yin et al., 2017). Recently, multiple effector-encoding genes were identified in P. viticola transcriptome during the interaction between V. vinifera cv. Mgaloblishvili and P. viticola (Toffolatti et al., 2020). The latter thus found a possible susceptibility gene, a transcription factor that was down-regulated in the resistant cv. Mgaloblishvili (Toffolatti et al., 2020).
Life Cycle and Infection Mechanisms
The early phases of plant infection by oomycetes remains not fully understood. Like many oomycetes, P. viticola pass through both sexual and asexual stages. There are two phases in asexual reproduction: sporangiogenesis, formation of multinucleate sporangia, and zoosporogenesis, formation of biflagellate zoospore that infects the grapevine host. During pathogen growth, intercellular mycelia reach the substomatal cavity and form a cushion from which sporangiophores arise on the underside of the leaves or stems through stomata and through lenticels in young fruits and form sporangia (under dark conditions with high humidity). Spores are termed sporangia if they germinate indirectly through internal production of biflagellate motile zoospores that are released, encyst and then germinate using a germ tube, or conidia if they germinate directly via formation of a germ tube. Spores are generally released by a twisting motion of the sporangiophore during warm, moist conditions. Sporangia discharge their zoospores through the rupture of the sporangium callose apical papilla. Spores stay active from a few hours to days, depending on environmental conditions, and are not able to survive in absence of water since they do not have cell walls. Therefore, mycelium of downy mildew is programmed not to sporulate until humid conditions appear. Yin et al. (2017) have reported that P. viticola has lost thiamine biosynthetic pathway encoding genes, similarly to as barley powdery mildew and flax rust. Interestingly, while five oomycetes namely P. viticola, H. arabidopsidis, P. infestans, A. laibachii, and P. sojae, have lost these thiamine biosynthetic pathway genes, but they conserved the thiamine pyrophosphokinase gene which codes for the phosphorylation of thiamine. Probably because thiamine may be more readily available from the host than other nutrients. Consequently, the thiamine biosynthetic pathway may be considered as the first to be compromised in the course of the evolutionary process toward biotrophy, compared to other metabolic pathways.
Disease Cycle and Symptoms
P. viticola is strictly biotrophic, i.e., it can grow only in association with living cells of its host and not in vitro, which makes it difficult to study. Its oospores can land in the soil, where they remain inactive until favorable conditions occur. It will then transfer onto a host plant and start to grow (Agrios, 2005). The life cycle of this pathogen consists of primary and secondary infections (Chen et al., 2019). It overwinters as oospores (resting sexual spores) in leaf litter, shoots, and soil (Rossi et al., 2009) (and, in some cases, as mycelium in infected, but not dead, twigs). In the spring, oospores germinate to produce macrosporangia which, under wet conditions, release zoospores (asexual spores) which encyst and infect when they come in contact with host tissues. The encysted zoospores produce germ tubes which invade the host through stomata and colonize intercellularly the leaf parenchyma with a diploid, non-segmented tubular mycelium that uptakes nutrients from host cells using intracellular haustoria (Allègre et al., 2009).
The period of intercellular mycelium growth is latent with no visible symptoms of infection (Leroy et al., 2013). After 7–10 days, symptoms of primary infection appear on the adaxial side of leaves, typically as yellow areas called “oil spots” that later turn brown due to necrosis as the disease progresses. Under moist conditions and warm temperature (above about 25°C), masses of sporangia emerge through stomata in leaves and lenticels in young fruits at the end of latency (Fröbel and Zyprian, 2019). These sporangia form the “white downy” appearance that will progress to gray. Sporangia produce zoospores that are released through wind or raindrops, starting secondary infections. The ultimate result of leaf injuries is a premature defoliation. In shoots, enlargement of the infected cells and the large volume of mycelium in intercellular areas triggers distortion and hypertrophy, which leads to their death and collapse, generating brown and sunken shoots. After an appearance of sporangiophores in young berries, the intercellular infection causes the breakdown of chlorophyll, then cells collapse and become brown. On adult berries, the fruit's “skin” thickens and its shape distorts. A color change to a reddish brown can be noted as well as a mosaic pattern when grapes are infected. At the end of the growing season, the oomycete forms oospores in the infected leaves and, occasionally, in the shoots and berries. Depending on temperature, humidity, and varietal susceptibility, the full disease cycle takes from 5 to 18 days (Agrios, 2005).
Proposed Entry Mechanisms
After discharge, zoospores start to move in a search of a new host. The anterior flagellum bends and propagates a sinusoidal wave from its base to a tip, pulling the zoospore forward. The posterior (whiplash) flagellum helps the zoospore to turn. Zoospores detect chemical and electrical gradients (generally non-specific in terms of pathogen-host associations) that attract them to plant surfaces. Once zoospores reach a potential host, they point the flagellum toward the host surface, find a stomatal complex, and encyst. During encystment zoospores detach both flagella, secrete extracellular matrix materials and change ovoid cells to spherical cysts with cellulosic cell walls. Within 30 min of encystment, zoospores germinate using a germ tube that forms an appressorium through the stoma (Allègre et al., 2007). Cyst germination and production of a germ tube return the pathogen to a passive state before restarting reproduction.
P. viticola hyphae do not remain exterior to the host. Oomycetes produce appressoria that penetrate the plant surface using physical and chemical mechanisms: penetrating by pressure and cell wall-degrading enzymes. A penetration hypha grows from an appressorium underside, passes through the stoma and produces an intercellular, or invasive, hypha. Thin penetration hyphae degrade the plant cell wall, grow through it and invaginate the plasma membrane. This enlarges to form feed structures called haustoria. The invaginated region of a host membrane is called an extrahaustorial membrane. Successful colonization by the pathogen occurs when a susceptible host defense fails and infection culminates in a downy mildew sporulation: sporangia form on the plant surface or sexual spores form inside host tissues, and the life cycle starts again. The asexual cycle of P. viticola is schematized in Figures 6, 7 (Agrios, 2005; Tröster, 2016). In addition to asexual reproduction, downy mildews form and propagate oospores. This process provides a genetic variation mechanism and forms spores that can survive for many years in harsh conditions (e.g., lack of water, extreme temperatures or aggressive chemical environments). Normally oospores are produced under rather dry conditions in a huge quantity (up to 250 oospores per mm2). Oospores mature during the winter and germinate once exposed to water and a temperature of at least 10°C.
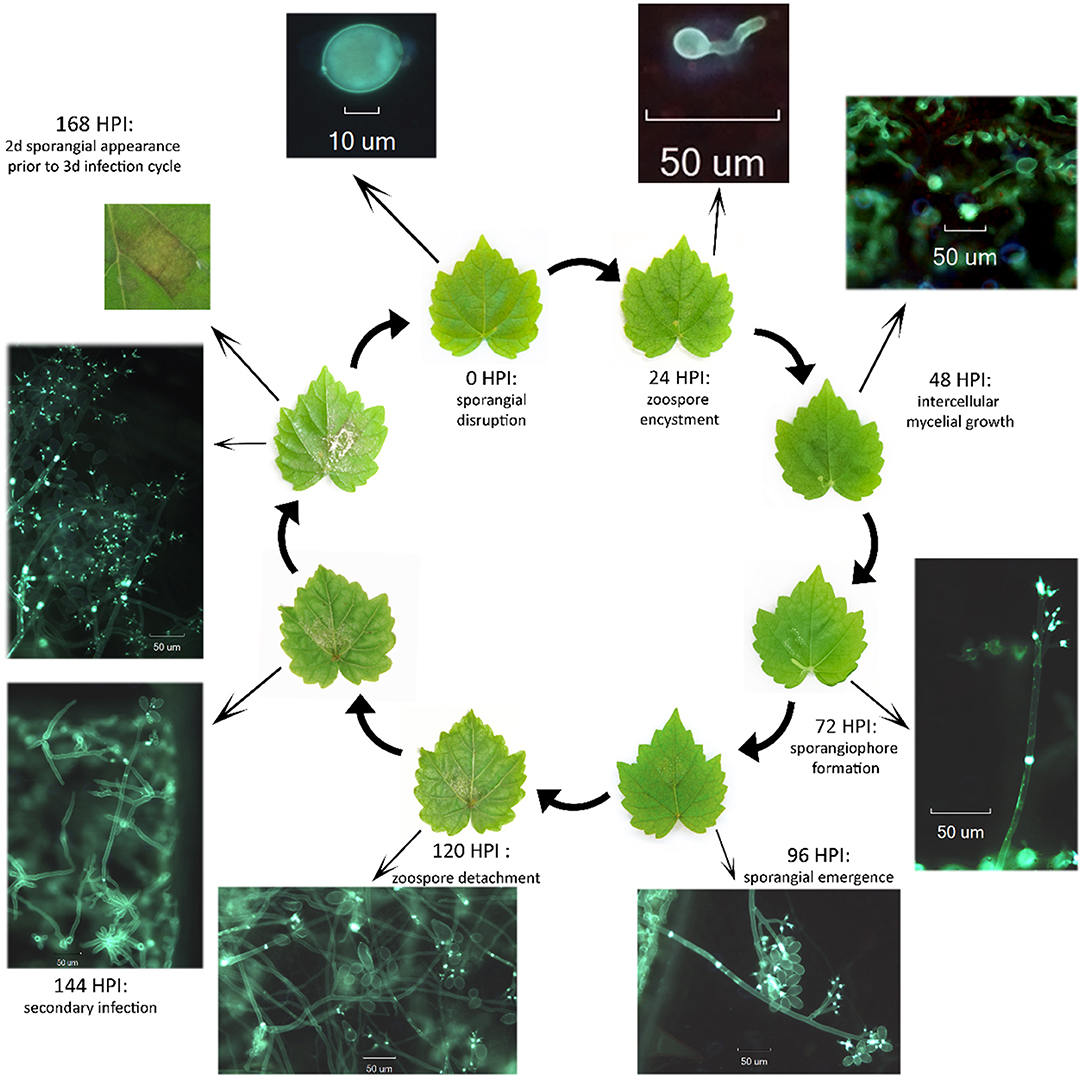
Figure 6. Life cycle of Plasmopara viticola. The represented cycle includes asexual stages of the pathogen development, disease cycle and host symptoms connected altogether. The microscopical observations of the pathogen within the plant tissues had been conducted under the epifluorescence microscope (Olympus Bx43, Japan) using a U/B/G filter and images were captured using Infinity Analyze software.
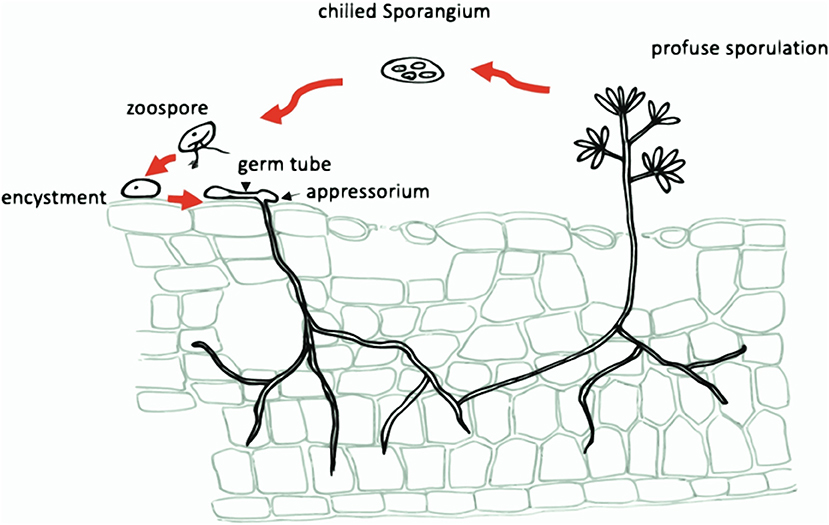
Figure 7. Cross section of leaf infected by downy mildew. The pathogen structures are colored black, and all the stages of asexual life cycle are represented in the same plane of gray-colored plant cells, abaxial surface up.
Heterothallic parents—P1 and P2 in Plasmopara—form antheridia and oogonia and differ in production and detection of hormones. This establishes a system of hormonal heterothallism, where perception of the opposite hormone establishes compatibility. Once compatibility occurs, gametangia develop and become able to form both selfed and outcrossed couplings. If both hormones are present in heterothallic oomycetes, there is no barrier to selfing, in a contrast to true fungi where sexual dimorphism or self-incompatibility inseparably linked with a heterothallism (Agrios, 1969; Wong et al., 2001).
During sexual reproduction, parental gametangia undergo meiosis to produce a haploid nucleus. Small male antheridia grow on the surface of large female oogonia (unfertilized eggs) and produce fertilization tubes which penetrate the oogonium. The single antheridial haploid nucleus passes through a fertilization tube into the oosphere and fuses with a single haploid nucleus from the oosphere, forming a diploid oospore (fertilized egg cell or zygote). Oogonia contain about 10 gametic nuclei, but only 1 oogonic nucleus fertilizes successfully, giving birth to the diploid zygotic nucleus. The remaining unfertilized oosphere nuclei migrate to the periplasmic space and degenerate, surrounded by a thick double-layered oogonium wall with inner and outer oosphere walls. Oospores mature when the previously thin inner cell wall becomes thick and then a dormancy period occurs, during which the oospore does not germinate even under optimal conditions. Subsequent germination depends on several important factors such as light, temperature, humidity, plant extracts and microbial metabolites. Germinated oospores form primary zoosporangia (macrosporangia), which release zoospores that act as described above (Burruano, 2000; Agrios, 2005; Lamour and Kamoun, 2009; Spencer-Phillips et al., 2016; Tröster, 2016).
How Grapevine Plants React
Perception of the Pathogen
During contact between pathogens and host plants a dynamic evolutionary battle rise that can be explained as a “zigzag” model (Jones and Dangl, 2006). To prevent infection by biotrophic pathogens, including the downy mildew, plants have evolved two complex signaling systems that sense pathogens or disease-related disorders. In addition to detecting pathogen molecular patterns, they are also able to sense the pressure produced by hyphae, which try to breach the plant's epidermis. During early stages of the infection, plant responses to oomycetes are identical, just less effective and slower in susceptible plants (Silvar et al., 2008; Huibers et al., 2009). Plants recognize wide conserved pathogen-associated molecular patterns (PAMPs) through pattern recognition receptors (PRRs) situated in plasma membranes, thus triggering the first barrier of innate immune system called PAMP-triggered immunity (PTI) (Jones and Dangl, 2006). Some pathogens developed a virulence through which they avoid or suppresses resistance reactions by secreting effector proteins that suppress the PTI signaling pathway, as well as modulating the host metabolism to enable the biotrophic interaction. In a response, plants developed an effector-triggered immunity (ETI), which involves the perception of specific effectors either directly or indirectly by R proteins. Once effectors are recognized, the plant will activate a second circle of defense reactions including hypersensitive response (HR) and systemic acquired resistance (SAR) (Brader et al., 2017; Esmaeel et al., 2018).
In broad terms, the compatible interaction between grapevines and P. viticola is marked by an overall down-regulation of photosynthesis-related processes and an impaired up-regulation of genes encoding pathogenesis-related (PR) proteins, enzymes of the phenylpropanoid pathways, and stimulus response regulators (Nascimento et al., 2019). In response to P. viticola, several infection genes are triggered including the stomatal movement related, BLUS1 (Takemiya et al., 2013), a growth related gene, bZIP11 (Weiste et al., 2017), a detoxification-related gene, the glutathione peroxidase gene, and an abiotic stress regulation gene ERF38 (Fu et al., 2020).
Host Resistance to Downy Mildew
Throughout their life, plants are exposed to a wide range of potential pathogens and pests. Therefore, they developed complex mechanisms to protect themselves from diseases. Normally defense mechanisms are triggered after the pathogen attack, leading to induced resistance. Depending on the inducer, resistance can be defined as either SAR and induced systemic resistance (ISR) (Pieterse et al., 1996). Besides inducible defense response, plants use preformed resistance including structural and biochemical factors. Structural aspects (e.g., properties of the leaf surface or resistant cell walls) are the first barrier that pathogens face before invading the plant. If the microorganism overcomes this line of non-specific resistance and colonizes the plant successfully, the plant becomes its host and induced resistance mechanisms then respond (Slusarenko et al., 2000; Mysore and Ryu, 2004).
Phytohormones play a crucial role as signaling molecules for disease resistance. Perception of oomycete pathogen by the plant triggers a series of signaling physiological and metabolic pathways in cells by regulating resistance-related genes and initiating the corresponding immune responses including HR (Koornneef and Pieterse, 2008; Halim et al., 2009). Effective defense against biotrophic pathogens is considered to depend largely on triggering the defense responses controlled by the SAR-dependent pathway, leading to host's programmed cell death (Glazebrook, 2005).
Plant defense responses require energy and activation of signaling molecules, supplied by primary metabolism of carbohydrates, organic acids, amines, amino acids, and lipids. Immune responses include the formation of salicylic acid (SA), jasmonic acid (JA), ethylene and abscisic acid (ABA) (Bari and Jones, 2008). Some beneficial microorganisms are able to not only directly inhibit pathogenic growth via hyperparasitism or metabolic activities and supply plant by nutrients or hormones, but also they have an ability to stimulate plant defense mechanisms, which lead to ISR (Pieterse et al., 1996; Van Loon et al., 1998). ISR is characterized by rapid molecular and cellular defense responses, priming the plant for more fast and effective reaction to pathogen infections, controlling plant energy costs. These responses differ and depend on the plant/pathogen/beneficial agent system.
Induction of systemic resistance occurs when host-specific receptors recognize pathogenic or beneficial microorganisms through pathogen-molecular patterns (PAMPs) or microbe-associated molecular patterns (MAMPs), elicitors that are produced by invasive microorganisms to suppress plant defense and include peptides, metabolites, cell wall components, enzymes, and toxins (Jones and Dangl, 2006). Damaged host cells produce damage-associated molecular patterns (DAMP), recognized by the pattern recognition receptors (PRRs) that trigger specific cell signals, leading to PAMP-triggered immunity (PTI). These cell signals induce an expression of defense genes, coding the synthesis of antimicrobial compounds and defense proteins involved in the management of oxidative stress, pathogen degradation, compounds mobilized to strengthen the host cell walls, and change plant metabolism toward defense (Kushalappa et al., 2016; Savini et al., 2018; Héloir et al., 2019).
P. viticola is known to trigger several defense responses in leaf tissues, including physical (i.g. lignin, deposition callose) and chemical (i.g. synthesis of chitinases, glucanases, peroxidases, stilbenes) barriers, and factors that are involved in lignification processes as well as necrosis that may lead to resistance development (Dai et al., 1995). For instance, peroxidase was reported as age-related resistance in a number of grapevines-pathogen interactions including P. viticola (Reuveni, 1998; Steimetz et al., 2012).
HR is a generation and accumulation of reactive oxygen species (ROS), which play a crucial role in resistance to pathogens either by directly reinforcing plant cell walls trough cross-linking of glycoproteins, lipid peroxidation or triggering the ROS-signaling networks resulting to the establishment of a local cell death around the point of infection, which blocks further development of biotrophic parasites (Govrin and Levine, 2000; Hernández et al., 2016). Local cell death is a type of programmed cell death (PCD), mechanism that is specific for all organisms from bacteria to multicellular eukaryotes (Locato and De Gara, 2018).
Systemic acquired resistance is characterized by a diffusion of hormonal signals from the invasive point through the whole plant, informing cells of all the organs, both infected and not, to activate their defenses for further attack, and launching a wide-spectrum resistance in tissues distant from the site of initial infection. SAR is usually induced after the exposure to abiotic or biotic elicitors, related to localized necrotic lesions. It is associated with an accumulation of the phytohormone SA, playing an important role in signal transfer, and activation of defense-related genes, encoding pathogenesis-related (PR) proteins with antimicrobial activity (Corina Vlot et al., 2009). An upregulation of genes involved in the biosynthesis of JA and an increase of JA levels suggest the crucial role of this hormone in V. riparia resistance toward P. viticola (Polesani et al., 2010). The up-regulation in VvWRKY1 overexpressors related to JA metabolism and signaling has been demonstrated to increase tolerance to fungal pathogens (Marchive et al., 2013).
Structural mechanisms, aimed to produce physical barriers against infection, include deposition of cutin, cuticular waxes, lignin and callose for cell wall reinforcement at infection sites (O'Connell and Panstruga, 2006; Miotto-Vilanova et al., 2016; Paris et al., 2016; Ali et al., 2018; El-Sharkawy et al., 2018). Cell wall deposition or papillae formation might result from PTI that is mediated by receptor-like kinases (RLKs) or receptor-like proteins (RLPs) localized in plant cell membrane (Boutrot and Zipfel, 2017).
Antimicrobial secondary metabolites like phytoanticipins (mainly saponins) and phytoalexins form a biochemical resistance. The main stilbenic phytoalexins in grapevine are resveratrol and its derivatives: viniferin, glycosylated piceid and pterostilbene. Stilbenic phytoalexins showed biological activity against several grapevine pathogens and are considered as markers of disease resistance (Coutos-Thévenot et al., 2001; Pezet et al., 2003; Aziz et al., 2006, 2016). Resistant grapevine cultivars react quickly to P. viticola attacks by producing high levels of stilbenes at the infection area, and have significant inhibitory impacts on the motility of P. viticola zoospores and therefore subsequent disease development (Pezet et al., 2004). These findings endorse the fundamental role and efficiency of phytoalexins in the grapevine resistance mechanisms to downy mildew (Alonso-Villaverde et al., 2011).
What Makes Plants Resistant or Susceptible?
Plants react to both biotic and abiotic factors to activate of set of genes that encode stress-related proteins for signaling, sensing, and defending the host against changes. The vulnerability of Vitis vinifera to downy mildew implies that this species does not have a specific identification system for P. viticola that would allow the triggering of an efficient defense mechanism (Di Gaspero et al., 2007). Transcriptional changes related to P. viticola infection of susceptible grapevines have been linked to a weak defense response (Polesani et al., 2010) and to an establishment of a compatible interaction (Liu et al., 2016). On the other hand, resistant genotypes exhibit a strong and fast transcriptional reprogramming of processes connected to defense, signal pathway, and a secondary metabolism (Kortekamp, 2006; Figueiredo et al., 2012). These changes include an acceleration of the expression of genes encoding PR proteins and phenylpropanoid biosynthesis, and those involved in the modulation of signal transduction components and markers of hypersensitive response in resistant grapevine cultivars (Malacarne et al., 2011; Figueiredo et al., 2012). These data imply that tolerant and susceptible cultivars not only have a separate metabolic pattern, but also react quite differently when challenged with biotic stress. Within plant-pathogen interactions, pathogens and their elicitors that are governed by AVR genes confront pathogenesis-related proteins (PR) and resistance (R) metabolites, regulated by related genes. So, disease resistance is a quantitative characteristic, which is regulated by complex of genes. To investigate differences between susceptible and resistant strains and select the most appropriate plant genotypes, analysis of R-gene expression levels had been used since the development of genomic sequencing, in addition to trait-specific germplasm identification, quantitative trait loci (QTLs) mapping and other genetic approaches (Zhao et al., 2019).
Disease Management
Breeding Approaches
The best and most sustainable tool in crop protection is the breeding of resistant grapevine cultivars. Unfortunately, the successful introgression of resistance loci inside of existing cultivars in conventional breeding techniques typically takes ~15 years and is expensive. Within crops, important traits representing productivity, stress tolerance and disease resistance are complex, polygenic, and quantitative, which means that numerous genes control genetic variation of these traits in each culture. Identification and genome mapping of QTLs, DNA sections correlating with variation of quantitative traits, is carried out using molecular markers. DNA-markers are used in gene incorporation in cultivars selected for introduction. With methods of map-based tagged genes cloning, constituting marker-assisted selection (MAS), QTL mapping allows us to understand an association between plant genotype and phenotype and use this strategy for the creation of new cultivars with optimal agronomic traits, including disease resistance (Keller et al., 2000).
The development of genome editing approaches, such as well-known CRISPR-Cas9, let scientists to change gene structure and expression in a targeted way for many purposes including disease resistance. This technology was adapted from a naturally occurring genome editing system that bacteria use as an immune defense to “remember” bacterial viruses and cut the viral DNA in subsequent attack which disables the virus. Once introduced into cells, the guide RNA cuts the DNA at the targeted location (usually using Cas9 enzyme) and then researchers use the cell's own DNA repair machinery to add or delete pieces of genetic material, or to make changes to the DNA by replacing an existing segment with a customized DNA sequence (Gupta and Musunuru, 2014; Wang et al., 2016).
While the majority of traditional European cultivars (Vitis vinifera) are mildew-susceptible, some American (V. rotundifolia, V. rupestris, V. labrusca, V. riparia, V. cinerea) and Asian (V. amurensis, V. piasezkii, V. coignetiae) grapevine cultivars exhibit partial to total resistance to downy mildew, so their germplasms are still the main source of genetic resistance to P. viticola (Jackson, 2000; Cadle-Davidson, 2008; Díez-Navajas et al., 2008). In recent decades, genetic analysis of a resistance to downy mildew resulted in identification of the most important genetic resistance factors with their corresponding loci (Schneider et al., 2019). Since the genetic identification of the first resistance locus against downy mildew, resistance to P. viticola (Rpv) 1 in Muscadinia rotundifolia (Merdinoglu et al., 2003), 31 P. viticola-resistance loci have been mapped in American and Asian wild accessions exploiting first- and second-generation molecular markers. Using backcrosses between parental lines of susceptible V. vinifera obtaining the best agronomic parameters (yield, growth, organoleptics) and genotypes carrying different Rpv loci, multiple studies on varietal selection were carried out.
Among the Rpv mapped in Vitis and Muscadinia, five (Rpv8, Rpv10, Rpv12, Rpv25, and Rpv26) were derived from the East Asian species V. amurensis (Fu et al., 2020). The locus Rpv1 was introduced from V. rotundifolia, located on its chromosome 12 and used in selection of such cultivars as Artaban, Floreal, Voltis (Merdinoglu et al., 2003; Savini et al., 2018; Schneider et al., 2019). Rpv2 from the chromosome 18 of V. rotundifolia was responsible for total resistance of its progenies (Gessler et al., 2011; Merdinoglu et al., 2018). Locus Rpv3, mapped on chromosome 18 and originated from American V. rupestris, is characterized by multiple resistance alleles (Bellin et al., 2009; Schwander et al., 2012; Schneider et al., 2019). Popular resistance haplotype Rpv3-1 was maintained in such cultivars as Regent, Bianca, Villard blanc, Cabernet blanc, Kozma 20-3 (Bellin et al., 2009; Ciubotaru et al., 2021; Wingerter et al., 2021). Rpv3-3 was found in Seyval, Merzling and Solaris grapevine. Rpv4, originated from American grapevine, was identified in Regent. Introduced from V. riparia, Rpv5 was found on a chromosome 9 in a progeny between Cabernet Sauvignon and Gloire de Montpellier and Rpv6 was identified in the same research with the only distinction that it was mapped on chromosome 12 (Marguerit et al., 2009; Schwander et al., 2012). Locus Rpv8 from V. amurensis was found to confer total resistance and belong to chromosome 14 (Blasi et al., 2011). Rpv10 originated from V. amurensis was mapped on chromosome 9 in Solaris and was introduced in cultivars Bronner, Divico, and Divona (Schwander et al., 2012; Schneider et al., 2019). Rpv11, founded on chromosome 5 in a population from Regent, was related to low level of downy mildew resistance in some cultivars of Regent, Chardonnay and Solaris (Schwander et al., 2012; Van Heerden et al., 2014). Introduced from V. amurensis, dominant Rpv12 was detected in a chromosome 14 of Kozma 20-3 cultivar together with Rpv3-1 (Venuti et al., 2013; Kim et al., 2017; Possamai et al., 2020). Recently, linkage analysis identified three QTLs for P. viticola resistance: Rpv22 on LG 02, Rpv23 on LG15, and Rpv24 on LG18 (Fu et al., 2020). P. viticola trigger the expression of the NBS-LRR-like gene RPP8, and might be considered as a candidate gene for Rpv23 (Fu et al., 2020). Additional class of R gene, LRR receptor-like serine/threonine-protein kinase (LRR-RLK), which have been characterized in the Rpv24 region, denoting that these two genes could also play a role in disease resistance mechanisms. Two stable QTLs had been located on LG 15 in a study of progeny derived from the cross between V. vinifera L. cv. Red Globe and V. amurensis Rupr. cv. Shuangyou: Rpv25 and Rpv26 (Lin et al., 2019). A QTL named Rpv27 was detected on a chromosome 18 during a mapping population study of a cross between V. aestivalis hybrid Norton and V. vinifera cv. Cabernet Sauvignon (Sapkota et al., 2019). A locus named Rpv28 had been found on a chromosome 10 during a study of the population derived from a cross between V. rupestris cv. Scheele and V. riparia Michx (Bhattarai et al., 2021). In a population mapping study of Georgian-derived accessions (Southern Caucasus), 3 novel loci Rpv29 (Chr14), Rpv30 (Chr3), and Rpv31 (Chr16) had been discovered (Sargolzaei et al., 2020).
Chemical Control
Despite cultural practices and the selection of resistant cultivars, currently, no effective biological control measures are known against P. viticola. Consequently, chemical control remains the most effective and economical strategy to protect crops from downy mildews under conditions favorable to disease development. Copper-based fungicides, the oldest products in plant protection, are still used in viticulture, in spite of long-term accumulation of Cu in soils, leading to anthropogenic pollution, negatively affecting soil fertility and microbiota (Pietrzak and McPhail, 2004; Thuerig et al., 2018a). In recent years, use of copper was reduced due to development of new fungicide formulations and safer application techniques; nevertheless, reduction or elimination of crop copper and synthetic pesticides is one of the most important aims for researchers in plant protection.
In spite of high level of protection against harmful plant diseases, chemical control faces some challenges. Firstly, oomycetes penetrate into the leaf tissues by formation of hyphae and haustoria (Toffolatti et al., 2011a). Many fungicides, especially contact types, accumulate on and in the waxy cuticle layer and do not penetrate deeper into the leaf (Vladimíra Zelená, 2007). Also, these pathogens can overwinter in soil and fallen leaves for long periods (Toffolatti et al., 2011b), while pesticides require multiple applications (Halleen et al., 2016). In addition the problem of potential harm to humans and the environment (Chitarrini et al., 2017), development of acquired fungicide resistance and emergence of new pesticide-resistant pathogenic strains are ongoing concerns (Leroux et al., 1999; Lucas et al., 2015; Campbell et al., 2021; Massi et al., 2021).
The most widely used technique to control downy mildew in vineyards, including organic ones, are multiple applications of copper compounds such as oxychloride, hydroxide, sulfate oxide (Tromp and De Klerk, 1988; Hofmann and Kauer, 2008; La Torre et al., 2008; Rusjan, 2012). Bordeaux mixture, a water solution of copper sulfate (CuSO4) and lime [Ca(OH)2], has been used since 1882, when French botanist A. Millardet found an inhibitory effect of copper against downy mildew (Millardet, 1885; Vecchione et al., 2007; Gessler et al., 2011; Zhang et al., 2017). Burgundy mixture, a water solution of copper sulfate and sodium carbonate, has been used since 1887 (Masson, 1887; Gessler et al., 2011; Rusjan, 2012).
Copper-based compounds are included in “protectant” multi-site fungicides that are used in pre-infection applications to form a protective barrier at the plant surface. This group includes dithiocarbamates such as mancozeb, zineb, maneb, propamocarb, metiram, propineb (Clifford and Bruyns-Haylett, 1978; Falk et al., 1996; Gisi, 2002; Šrobárová et al., 2007; Gisi and Sierotzki, 2008; Patil, 2015); phthalimide group, represented by captan, captafol, and folpet (O'Neill et al., 1996; Madden et al., 2000; Genet et al., 2006; Rekanovic et al., 2008; Gullino et al., 2010; Jermini et al., 2010a; Gessler et al., 2011; Barros et al., 2018); phthalonitrile chlorothalonil (Northover and Ripley, 1980; Gisi et al., 2007; Lebeda et al., 2008; Barros et al., 2018) and quinone dithianon (Corio-Costet, 2011; Nanni et al., 2016). Phenylamides and aluminum are two of the most broadly applied systemic fungicides to manage P. viticola.
Single-site fungicides provide both pre- and post-infection protection and include four main chemical classes: phenylamide compounds such as mefenoxam, metalaxyl, and benalaxyl (Magarey et al., 1991; Nicholas et al., 1994; Falk et al., 1996; Underdown et al., 2008); strobilurines such as azoxystrobin, trifloxystrobin, famoxadone, and fenamidone (Reuveni, 2001; Wong and Wilcox, 2001; Genet et al., 2006); the carboxylic acid amides like dimethomorph, iprovalicarb, flumorph, benthiavalicarb, mandipropamid (Wicks and Hall, 1990; Albert et al., 1991; Gisi et al., 2007; Reuveni, 2014; Nanni et al., 2016; Kim et al., 2017); and cyano-acetamide-based oxime carbonates like cymoxanil (Gullino et al., 1997; Genet and Vincent, 1999; Joshi, 2003). Both cymoxanil and chlorothalonil are specific, non-systemic, mildew fungicides and are curative if they are applied during the first few days post infection. Less popular single-site fungicides include phosphonates as fosetyl-Al (Magarey et al., 1991; Gisi, 2002; Rekanovic et al., 2008) and dinitroaniline fluazinam (Gisi and Sierotzki, 2008; Lebeda et al., 2008; Espinel-Ingroff, 2009).
Organic Disease Management
Crop protection agents suitable for organic agriculture include plant, animal, microbial and mineral products, and possess different modes of action: antibiosis, induction of resistance and hyperparasitism (Dagostin et al., 2011). Several products, based on inorganic materials are currently registered and applied in plant protection against grapevine downy mildew.
Potassium hydrogen carbonate showed inhibitory activity against spores of P. viticola and high level of protection in field applications under moderate to low infection levels (La Torre et al., 2008, 2019; Parveaud et al., 2010; Dagostin et al., 2011) and in greenhouse (Lukas et al., 2016). Potassium phosphonate was effective against downy mildew in field conditions, but its application leads to phosphonate residues in wine; the level of accumulation depends on the application strategy (Speiser et al., 2000; Vecchione et al., 2007; Hofmann and Kauer, 2008; Parveaud et al., 2010; Corio-Costet, 2011; Ball et al., 2014). Lime sulfur, a mixture of calcium polysulfides and thiosulfate, was effective under controlled conditions (Lukas et al., 2016). Propolis was tested in semi-controlled conditions and showed promise for suppressing pathogen growth (Dagostin et al., 2011). Oligochitosan, a deacetylated derivative of chitin, was found to promote plant defense reactions. It increased accumulation of phytoalexins, resveratrol and ε-viniferin, significantly reducing infection severity on grapevine under natural and semi-controlled conditions (Aziz et al., 2006; Vecchione et al., 2007; Dagostin et al., 2011; Romanazzi et al., 2014).
Among the synthetic materials, beta-amino-butyric acid (BABA), benzothiadiazole (BTH) and high rates of Tween 80 (250 ml/l) were effective under controlled conditions. Only BTH is registered as a commercial product, Bion (Dagostin et al., 2011; Savini et al., 2018). The application of non-protein amino acid BABA (DL-3-amino-n-butanoic acid, beta-aminobutyric acid) efficiently protected field-grown grape against P. viticola. BABA decreased sporulation when applied on leaf discs (Cohen et al., 1999; Vecchione et al., 2007; Kim Khiook et al., 2013), on detached leaves (Dubreuil-Maurizi et al., 2010), in potted plants when the root system was treated (Cohen et al., 1999), and in a greenhouse (Slaughter et al., 2008; Dagostin et al., 2011) and in field trials (Reuveni et al., 2001). BTH is a synthetic (and less phytotoxic) functional analog of salicylic acid and is registered in the EU as a crop resistance inducer. Results of test on leaf disks showed that sporal growth was inhibited up to 100% at high concentration (Cohen et al., 1999; Dufour and Corio-Costet, 2012). On the other hand, there was no direct toxic effect against sporangia germination after treatments with BTH on detached leaves. In the same study under greenhouse conditions, leaf treatments decreased disease severity even when applied 14 days before pathogen inoculation (Perazzolli et al., 2008; Burdziej et al., 2021).
Plant Extracts
Preparations obtained by the extracting of a specific solvent on a dried or fresh plant, including plant derivates (for example saponins) and oils sometimes show high level of effectiveness under controlled conditions but little success in the field. This is generally related to their high water solubility (Chapagain and Wiesman, 2006). Despite a wide range of protective properties of plant-derived products for plant protection, its usage in commercial agriculture is limited by high cost, low persistence and low-level of rainfastness.
In studies on leaf discs, extracts of Artemisia absinthium, Artemisia vulgaris, Achillea millefolium, Betula pendula, Calendula officinalis, Cannabis sativa, Chloris virgata, Daucus carota, Dalbergia hupeana, Dryopteris filix-mas, Frangula alnus, Glycyrrhiza glabra, Hibiscus trionum, Hypericum perforatum, Hyssopus officinalis, Magnolia officinalis, Origanum vulgare, Paeonia suffruticosa, Pastinaca sativa, Petasites albus, Picea abies, Pinus massoniana, Pinus pinaster, Rheum palmatum, Robinia pseudoacacia, Salix alba, Salvia officinalis, Symphytum officinale, Solidago canadensis, Tanacetum vulgare, Trigonella foenum-graecum, and Traxacum officinalae showed high inhibitory effect on sporangia germination (Kast, 2001; Chen et al., 2002; Godard et al., 2009; Harm et al., 2011; Tröster, 2016; Gabaston et al., 2017a,b; Andreu et al., 2018; James, 2018; Thuerig et al., 2018b).
Under field and greenhouse conditions, extracts of Equisetum arvense, Frangula alnus, Glycyrrhiza glabra, Inula viscosa, Larix decidua, Quillaja saponaria, and Yucca schidigera controlled grapevine downy mildew (Bennett and Lynch, 1981; Cohen et al., 2006; Dagostin et al., 2011; Arnault et al., 2012; Thuerig et al., 2016, 2018; Mulholland et al., 2017; James, 2018; La Torre et al., 2019). Extracts of Abies sibirica, Juncus effusus, Magnolia officinalis, Rheum rhabarbarum L., Rheum palmatum, Salvia officinalis, and Solidago virgaurea were able to suppress pathogen growth only under semi-controlled conditions (Kast, 2001; Godard et al., 2009; Dagostin et al., 2010, 2011; Harm et al., 2011; Toffolatti et al., 2011a; Thuerig et al., 2016; James, 2018; Ramseyer, 2018; La Torre et al., 2019).
Plant essential oils of Cymbopogon citratus, Cinnamomum zeylanicum, Eucalyptus globulus, Melaleuca alternifolia, Mentha piperita, Origanum majorana, Origanum vulgare, Thymbra spicata and citrus were effective under controlled conditions, but most studies showed decreased inhibitory activity in the field, especially with a long incubation period (Dagostin et al., 2010, 2011; La Torre et al., 2014; Maia et al., 2014; De Oliveira Fialho et al., 2016; Rienth Id et al., 2019).
Several plant derivatives significantly reduced disease expression under semi-controlled conditions. Fatty acid-based product Tecnobiol, containing oleic and linoleic acids, showed a good preventive action in greenhouse conditions but and less effectiveness in the field, where it requires serial applications (Palla, 2006; Dagostin et al., 2011).
Lecithin, a mixture of glycerophospholipids extracted from Glycine max, is applied against powdery mildew of different crops (Ramseyer, 2018). This substance showed high fungitoxic effect on sporangial germination in vitro and middle level of protection in field trials (Aveline and Chovelon, 2016; Marchand, 2018). Extract of the brown alga Ascophyllum nodosum reduced disease expression in laboratory and greenhouse experiments (Lizzi et al., 1998; Dagostin et al., 2011). Also, the brown alga L. digitata has been observed to trigger the grapevine plant defense mechanism and protect them against pathogens including P. viticola (Aziz et al., 2003).
The β-1,3-glucan laminarin derived from the brown alga Laminaria digitate is authorized for organic farming in EU (Ball et al., 2014). It effectively reduced P. viticola damages by plant defense responses in leaf disk assay (Gauthier et al., 2014), under semi-controlled conditions (Aziz et al., 2003; Trouvelot et al., 2008; Allègre et al., 2009; Romanazzi et al., 2014) and in the field when applied with a surfactant (Paris et al., 2016). The elicitor primed H2O2 production at the infection area, triggers the expression of defense genes, callose, and phenol depositions, and HR-like cell death (Trouvelot et al., 2008).
Biological Control
Like many other plants, grapevine can be colonized by huge numbers of microbial organisms in exterior (epiphytes) and interior (endophytes) surfaces of aboveground tissues and in the rhizosphere (Pinto et al., 2014; Zarraonaindia et al., 2015; Asghari et al., 2019). Biological control encompasses the use of beneficial microorganisms, such as specialized fungi and bacteria to assault plant pathogens and control diseases they cause (Table 3). Biological control offers an environmentally friendly approach to handle plant diseases as part of an integrated pest management system. In the EU Pesticide Database, 65 strains of microorganisms are currently approved in plant protection: 36 strains of fungi, yeasts, and oomycetes; 21 strains of bacteria; and 7 viruses (ec.europa.eu, actual data for June 2020). Among these agents, 32 strains possess fungicidal properties and 15 of them can be used against oomycetes.
The most popular formulation used at market is a wettable powder (WP) consisting of the lyophilized BCA cells, spores or conidia combined with wetting agents and sometimes bulking agents. Wettable powders are supplied in measured sachets to simplify premixing and reduce the contact between the product and environment or operator. Once solubilized, biocontrol products can be applied as a dilute suspension through liquid spraying equipment and drip type irrigation systems. Suspension concentrate (SC) is a liquid form of biocontrol products, more popular for bacterial agents. Less popular (nowadays) dry biocontrol formulations include dusts (DP) and powders for seed dressing (DS) when there is a need of protection against soil-born pathogens; granules (GR), micro granules (MG) and water dispersible granules (WG). Liquid formulations include emulsions, oil dispersions (OD), suspo-emulsions (SE), capsule suspensions (CS) and ultra low volume formulations (Gasic and Tanovic, 2013).
Fungal Agents
Over the last decades, several microorganisms isolated from different plant organs have been screened as BCAs to control P. viticola (Table 3). Endophytic fungi Acremonium persicinum and Acremonium sclerotigenum (the latter incorrectly identified as Acremonium byssoides), isolated from asymptomatic grapevine, showed a hyperparasitism on P. viticola. Culture filtrates, crude extracts and secondary metabolites acremines inhibited sporangial germination of P. viticola in anti-germinative assays (Assante et al., 2005; Burruano et al., 2008; Lo Piccolo et al., 2015). In a study on leaf disks, asexual reproduction of the pathogen was significantly inhibited: sporangiophores were found thin and deformed, growing only at the points of inoculation. In leaves, colonized by this endophyte, formation of sexual structures (gametangia and oospores) of the pathogen was earlier than in non-inoculated leaves, apparently an attempt to ensure its survival in a response to stress (Burruano et al., 2008).
Aureobasidium pullulans was tested outdoors (in a commercial product Aureo) on potted plants against P. viticola, where it provided very low protection; no direct effect on zoospores were observed in vitro. Under greenhouse conditions, the activity of the PR protein glucanase was weakly increased but not enough to consider this microorganism as a resistance inducer (Harm et al., 2011). The application of an inactivated suspension of apple-epiphytic yeast A. pullulans on grapevine callus culture and in vitro plantlets increased gene expression and enzyme activity of PAL and STS, demonstrating the possibility of a resistance induction mechanism.
Alternaria alternata, endophytic fungus isolated from grapevine leaves, showed high efficiency against downy mildew in pre-infection applications on leaf disks of a Pinot gris cultivar. In the presence of the endophyte in infected tissues, even without direct close contact between the 2 fungi, P. viticola indicated structural changes, possibly indicative of toxic metabolites causing antagonistic interactions: abnormal vacuolization, accumulation of electron-dense material in the vacuoles, and appearance of necrotic haustoria. Mass spectrometry confirmed presence of low-molecular-weight toxic metabolites, belonging to the diketopiperazines family (Musetti et al., 2006).
Grapevine endophytic fungus Epicoccum nigrum, isolated from P. viticola—infected leaves, inhibited sporangial germination in greenhouse conditions by hyphal surrounding. Due to the tight contact between sporangia of P. viticola and hyphae of E. nigrum, sporangia could not break off from sporangiophores, inhibiting its spread (Kortekamp, 1997).
Several Fusarium species, isolated from P. viticola—infected plants, showed a potential to control downy mildew. Fusarium delphinoides, F. brachygibbosum, 2 strains of F. pseudonygamai and non-identified Fusarium sp. strain MCC 1347 were tested in vitro, where they coiled around sporangiophores and caused sporangial distortion of the pathogen. Enzymatic activities produced extracellular lytic enzymes, potentially involved in mycoparasytic interactions, such as glucanase, chitinase, and protease (Ghule and Sawant, 2017). This was subsequently confirmed in pre-infection study on leaf discs and in a field trial. Sporangial production on leaf disks was reduced significantly as compared to untreated control (63–76%), and incidence of downy mildew in the field was also decreased on 8–10%, while chemical control showed 19% of disease reduction. Pre- and post-infection applications of Fusarium proliferatum G6, an endophytic fungus isolated from grapevine leaves, reduced sporulation and further growth of P. viticola on leaf disks and seedlings leaves of V. vinifera cultivar Riesling in a growth chamber. In field trials on a susceptible cultivar, Chancellor, incidence of downy mildew after an application of Fusarium proliferatum G6 was reduced similarly to chemical treatment with mancozeb (Falk et al., 1996). In later studies, isolate No̱1505 of this strain was found to produce extracellular hydrolytic enzymes β-glucosidase and endo-1,4-β-glucanase that can degrade cell wall components of oomycetes (Bakshi et al., 2001).
An aqueous extract of Penicillium chrysogenum dry mycelium by-product from penicillin industrial production, provided high protection of grapevine plants in a pre-infection foliar application under greenhouse conditions and in field trials with natural infection. The extract reduced disease severity under different disease pressures comparable to the copper fungicide and resistance inducers BTH or BABA. Crude extracts of an endophytic fungus Phomopsis sp. CAFT69, isolated from the medical plant Endodesmia calophylloides, inhibited motility and induced subsequent lysis of P. viticola zoospores. Zoosporicidal compounds were isolated and characterized (Talontsi et al., 2012).
Rhizophagus irregularis, an arbuscular mycorrhizal fungus (AMF), altered an expression of several P. viticola effectors which interfered with a pathogen's ability to infect the grapevine cuttings in semi-controlled conditions (Cruz-Silva et al., 2021).
In studies against several plant pathogens, Trichoderma harzianum suppressed the pathogen's growth by cell wall degradation using lytic enzymes such as proteases, glucanases, and chitinases (Haran et al., 1996; Reino et al., 2008). Trichoderma harzianum T39 (commercial product Trichodex) reduced the development of P. viticola on susceptible grapevine cultivars (Vitis vinifera cv. Pinot Gris and cv. Pinot Noir) under greenhouse conditions by the enhanced expression of defense-related genes, priming for enhanced expression of these genes, and increased induction of protective enzymes, leading to systemic resistance to downy mildew (Perazzolli et al., 2008, 2011; Palmieri et al., 2012; Kamble et al., 2021; Lazazzara et al., 2021). Also, the treatment by T. harzianum HL1, isolated from the cultivated soil, increased the POX activity and reduced disease severity in naturally infected grapevine plants under field conditions (El-Sharkawy et al., 2018). Soil-born Trichoderma viride HL5 was effective under field conditions. After natural infection, fungal treatment increased plant peroxidase level and reduced disease severity. In addition to the biocontrol effect, quality parameters of berries (TSS/acid ratio) and plant shoot length was significantly higher than in control treatment (El-Sharkawy et al., 2018). T. viride, like many members of Trichoderma genus, is known for its high cellulase production, an important mechanism involved in pathogen suppression (Li et al., 2010).
Bacterial Agents
During recent decades, beneficial endophytic bacteria have emerged as promising BCAs for their ability to enter and survive inside plant tissues (Compant et al., 2005). More recently, among 239 bacterial endophytes isolated from grapevine leaves, two endophytic strains, identified as Bacillus subtilis GLB191 and B. pumilus strain GLB197 have proved their preventive effects for P. viticola both on leaf disks and during 2-year field trials. Treatment with these BCAs produced more efficiency than chemical control (multiple application of 1% Bordeaux mixture and fungicide Polyoxin) 25 days after the natural infection (Zhang et al., 2017). Pesticide-resistant strain B. subtilis KS1, that was isolated from grape skin and produced an antifungal lipopeptide iturin A, decreased the incidence of downy mildew on berries and leaves in vineyards of sensitive cultivars Koshu and Merlot, where it was applied each week during the growing season (Furuya et al., 2011). Endophytic bacterium B. altitudinis GLB197 isolated from grapevine leaves showed significant inhibition of downy mildew both in leaf disk test and in field experiments (Zeng et al., 2021). Isolated from Vitis sp. cv.Koshu, B. velezensis strain KOF112 inhibited zoospore release from P. viticola and upregulated the expression of several pathogenesis-related genes (Hamaoka et al., 2021).
Copper-resistant Lysobacter capsici AZ78, isolated from tobacco rhizosphere, showed efficiency against grapevine downy mildew under controlled conditions, where disease severity on leaves was reduced similarly to application of copper fungicide (Puopolo et al., 2014b; Brescia et al., 2021). Direct inhibition of the pathogen was reached by production of the secondary metabolite 2,5-diketopiperazine that was found to be toxic against sporangia of some plant pathogenic oomycetes, including P. viticola (Puopolo et al., 2014a; Cimmino et al., 2020). In later studies, results of bacterial genome sequencing showed presence of genes encoding production of lytic enzymes responsible for cellulose, chitin, laminarin, and proteins degradation (Puopolo et al., 2016).
Bacterial strain SY286 of Ochrobactrum sp., isolated from asymptomatic downy mildew—infected grapevine leaves, had a high inhibitory activity against P. viticola in the detached leaf assay and under field conditions. SEM observation showed that this strain is able to destroy mycelium and sporangia of the oomycete; in vitro analyses detected production of such metabolites as siderophores, cellulases, proteases, and amylases that are important in BCA-plant-pathogen interactions (Zang et al., 2020).
Paenibacillus sp. strain B2, isolated from the rhizosphere of Sorghum bicolor showed high antagonistic activity against P. viticola due to the secretion of hydrolytic enzymes or induction of plant defenses. The strain B2 inoculated with the vesicular-arbuscular mycorrhizal fungus Glomus mosseae, was found to produce peptide paenimyxin that significantly reduced the mobility of P. viticola zoospores and mycelium growth of B. cinerea. When an extracted peptide was applied by spraying before the infection, it was not effective, suggesting a direct action of paenimyxin (Hao et al., 2017). Endophytic bacterial strain Pseudomonas fluorescens PTA-CT2, isolated from grapevine stems, reduced downy mildew disease by induction of systemic resistance in pre-infection applications on greenhouse-grown plants (Lakkis et al., 2019). P. fluorescens Pfl, isolated from the rice rhizosphere, induced plant systemic resistance after foliar application and subsequent infection with P. viticola. Talc-based formulation of Pfl strain was tested under greenhouse conditions, where activity of defense enzymes such as peroxidase, polyphenol oxidase and phenylalanine ammonia lyase was found to be increased in a response to the pathogen attack (Archana et al., 2011).
Terrestrial bacterial strain Streptomyces atratus PY-1 significantly reduced downy mildew severity in a detached leaf assay with slightly less reduction in a field trial on a low-resistant cultivar, Centennial Seedless. SEM observations showed disruption of pathogenic sporangia and sporangiophores. Secondary metabolites inhibited P. viticola sexual reproduction through reduced protein synthesis (Liang et al., 2016). In a previous in vitro study, Streptomyces sp. ANK313 inhibited motility of P. viticola zoospores and caused its lysis by the production of secondary metabolite khatmiamycin, an ester derivative of the antibiotic juglomycin (Abdalla et al., 2011; Yoshioka et al., 2019). S. viridosporus HH1 reduced disease severity under field conditions with a natural infection. Levels of polyphenol oxidase, total phenol content and parameters of berry quality were significantly better than in non-treated or chemically treated plants (El-Sharkawy et al., 2018). Under the same conditions, S. violatus HH5 not only reduced a disease severity and increased polyphenol oxidase level, but also improved parameters of plant growth, yield, chemical composition of leaves and berries.
Future Prospects and Conclusions
Huge progress had been made in understanding of interactions between P. viticola and grapevine since the recognition of this disease. Nevertheless, continued research in many aspects of these interactions should take a place to improve current control and develop new protective strategies. Modern molecular techniques offer insights into phylogenetic affiliations, pathogen susceptibility to control treatments and genetic aspects of plant resistance. Breeding new cultivars promise improved practical usage, safety, and endurance of the resistance without environmental concerns. Resulting resistant varieties that satisfy a broad range of requirements should be widely introduced in grapevine-growing regions. Furthermore, the effects of cultural practices on survival and viability of oospores requires additional study. The potential to introduce these practices offer improved effectiveness of pathogen control.
The role of collateral hosts that can act as a source of infection and the impact of weather and soil factors on asexual and sexual reproduction of pathogens should be investigated. Studying these relationships will be helpful for epidemiology and prognosis of disease development and will allow growers to choose optimal control strategies.
Integrated disease control should select the most appropriate and cost-effective techniques, while new schemes for plant protection are created. Weather and geographic conditions, resistance of cultivars, treatment with chemical and organic pesticides, use of biocontrol agents and resistance inducers all timed to pathogen stage offer the potential for significantly reduced pathogen effects and improved crop yields.
Biocontrol measures, while inherently attractive, face several challenges in practice, making this issue one of the most important directions for research moving forward. Technical problems in production, maintenance of pure culture in the case of liquid products, the search for optimal formulations suitable for farmers and their current equipment, duration and stability of products, extraction and purification of derivatives such as resistance inducers on an industrial scale are challenging and require additional research. Product registration is often complicated, expensive, and time-consuming, but necessary for safe introduction of new products to the market. Mechanisms of interactions between microorganisms and potential eukaryotic hosts should be scrupulously investigated to understand and avoid the potential production of human and mammal pathogens. Therefore, studies of interactions between BCA and the surrounding environment, for example, beneficial insects and other crops must also be considered.
Author Contributions
KK, QE, and EA conceptualized, wrote the first draft of the manuscript, and made the figures. KK, QE, CJ, JN, CC, and EA revised and approved the final version of the manuscript. All authors contributed to the manuscript structure and discussions.
Funding
This work was supported by the University of Reims Champagne-Ardenne, FEDER and the region Grand Est, France (VitEst project).
Conflict of Interest
The authors declare that the research was conducted in the absence of any commercial or financial relationships that could be construed as a potential conflict of interest.
Publisher's Note
All claims expressed in this article are solely those of the authors and do not necessarily represent those of their affiliated organizations, or those of the publisher, the editors and the reviewers. Any product that may be evaluated in this article, or claim that may be made by its manufacturer, is not guaranteed or endorsed by the publisher.
Acknowledgments
We gratefully acknowledge the financial support provided by the University of Reims Champagne-Ardenne, FEDER and the region Grand Est, France (VitEst project obtained in the frame of the Regional Cooperation Fund for Research).
References
Abdalla, M. A., Win, H. Y., Islam, M. T., Von Tiedemann, A., Schüffler, A., and Laatsch, H. (2011). Khatmiamycin, a motility inhibitor and zoosporicide against the grapevine downy mildew pathogen Plasmopara viticola from Streptomyces sp. ANK313. J. Antibiot. 64, 655–659. doi: 10.1038/ja.2011.68
Albert, G., Schwabenheim, F. R., Thomas, A., and Guehne, M. (1991). “Fungicidal activity of dimethomorph on different stages in the life cycle of Phytophthora infestans and Plasmopara viticola,” in International Conference on Plant Diseases, December 3-5 1991 (Bordeaux).
Ali, S., Ganai, B. A., Kamili, A. N., Bhat, A. A., Mir, Z. A., Bhat, J. A., et al. (2018). Pathogenesis-related proteins and peptides as promising tools for engineering plants with multiple stress tolerance. Microbiol. Res. 212–213, 29–37. doi: 10.1016/j.micres.2018.04.008
Allègre, M., Daire, X., Héloir, M. C., Trouvelot, S., Mercier, L., Adrian, M., et al. (2007). Stomatal deregulation in Plasmopara viticola-infected grapevine leaves. New Phytol. 173, 832–840. doi: 10.1111/j.1469-8137.2006.01959.x
Allègre, M., Héloir, M.-C., Trouvelot, S., Daire, X., Pugin, A., Wendehenne, D., et al. (2009). Are grapevine stomata involved in the elicitor-induced protection against downy mildew? Mol. Plant Microbe Interact. 22, 977–986. doi: 10.1094/MPMI-22-8-0977
Alonso-Villaverde, V., Voinesco, F., Viret, O., Spring, J. L., and Gindro, K. (2011). The effectiveness of stilbenes in resistant Vitaceae: ultrastructural and biochemical events during Plasmopara viticola infection process. Plant Physiol. Biochem. 49, 265–274. doi: 10.1016/j.plaphy.2010.12.010
Anderson, R. G., Deb, D., Fedkenheuer, K., and Mcdowell, J. M. (2015). Recent progress in RXLR effector research. Mol. Plant Microbe Interact. 28, 1063–1072. doi: 10.1094/MPMI-01-15-0022-CR
Andreu, V., Levert, A., Amiot, A., Cousin, A., Aveline, N., and Bertrand, C. (2018). Chemical composition and antifungal activity of plant extracts traditionally used in organic and biodynamic farming. Environ. Sci. Pollut. Res. 25, 71–82. doi: 10.1007/s11356-018-1320-z
Archana, S., Prabakar, K., Raguchander, T., Hubballi, M., Valarmathi, P., and Prakasam, V. (2011). Defense responses of grapevine to Plasmopara viticola induced by azoxystrobin and Pseudomonas fluorescens. Int. J. Sustain. Agric. 3, 30–38.
Arnault, I., Chovelon, M., and Derridj, S. (2012). “Preliminary tests in field conditions of alternatives substances against grape downy mildew in organic farming,” in Working Group “Biological Control of Fungal and Bacterial Plant Pathogens”, Biocontrol of Plant Pathogens in Sustainable Agriculture (Reims).
Asghari, S., Harighi, B., Ashengroph, M., Clement, C., Aziz, A., Esmaeel, Q., et al. (2019). Induction of systemic resistance to Agrobacterium tumefaciens by endophytic bacteria in grapevine. Plant Pathol. 69, 827–837. doi: 10.1111/PPA.13175
Askani, L., Schumacher, S., and Fuchs, R. (2021). Sequence and gene expression analysis of recently identified NLP from Plasmopara viticola. Microorganisms 9, 1–7. doi: 10.3390/microorganisms9071453
Assante, G., Dallavalle, S., Malpezzi, L., Nasini, G., Burruano, S., and Torta, L. (2005). Acremines A-F, novel secondary metabolites produced by a strain of an endophytic Acremonium, isolated from sporangiophores of Plasmopara viticola in grapevine leaves. Tetrahedron 61, 7686–7692. doi: 10.1016/j.tet.2005.05.094
Aveline, N., and Chovelon, M. (2016). “Résultats des essais en vignoble, conditions contrôlées et au labo cas du mildiou de la vigne,” in Journees PNPP, 26-27 avril 2016 (Paris), 1–28.
Aziz, A., Poinssot, B., Daire, X., Adrian, M., Bézier, A., Lambert, B., et al. (2003). Laminarin elicits defense responses in grapevine and induces protection against Botrytis cinerea and Plasmopara viticola. Mol. Plant Microbe Interact. 16, 1118–1128. doi: 10.1094/MPMI.2003.16.12.1118
Aziz, A., Trotel-Aziz, P., Dhuicq, L., Jeandet, P., Couderchet, M., and Vernet, G. (2006). Chitosan oligomers and copper sulfate induce grapevine defense reactions and resistance to gray mold and downy mildew. Phytopathology 96, 1188–1194. doi: 10.1094/PHYTO-96-1188
Aziz, A., Verhagen, B., Magnin-Robert, M., Couderchet, M., Clément, C., Jeandet, P., et al. (2016). Effectiveness of beneficial bacteria to promote systemic resistance of grapevine to gray mold as related to phytoalexin production in vineyards. Plant Soil 405, 141–153. doi: 10.1007/s11104-015-2783-z
Bains, S. S., and Jhooty, J. S. (1982). Distribution, spread and perpetuation of Peronosclerospora philippinensis in Punjab (India). Indian Phytopathol. 35, 566–570.
Bakshi, S., Sztejnberg, A., and Yarden, O. (2001). Isolation and characterization of a cold-tolerant strain of Fusarium proliferatum, a biocontrol agent of grape downy mildew. Phytopathology 91, 1062–1068. doi: 10.1094/PHYTO.2001.91.11.1062
Ball, K., Beck, A., Bouilhol, M., Cabaret, J., Ruiz, R. G., Halberg, N., et al. (2014). EGTOP: Final Report on Plant Protection Products (II) (Brussels).
Bari, R., and Jones, J. D. G. (2008). Role of plant hormones in plant defence responses. Plant Mol. Biol. 69, 473–488. doi: 10.1007/s11103-008-9435-0
Barr, D. J. S., and Désaulniers, N. L. (1992). The flagellar apparatus in zoospores of phytophthora, pythium, and halophytophthora. Can. J. Bot. 70, 2163–2169. doi: 10.1139/b92-267
Barreto, R., and Dick, M. W. (1991). Monograph of Basidiophora (Oomycetes) with the description of a new species. Bot. J. Linn. Soc. 107, 313–332. doi: 10.1111/j.1095-8339.1991.tb00226.x
Barros, L. B., Biasi, L. A., Carisse, O., and De Mio, L. L. M. (2018). The influence of table grape rootstock and cultivar combinations on susceptibility to downy mildew. Australas. Plant Pathol. 47, 171–179. doi: 10.1007/s13313-018-0550-3
Bartnicki-Garcia, S. (1968). Cell wall chemistry, morphogenesis, and taxonomy of fungi. Annu. Rev. Microbiol. 22, 87–108. doi: 10.1146/annurev.mi.22.100168.000511
Bellin, D., Peressotti, E., Merdinoglu, D., Wiedemann-Merdinoglu, S., Adam-Blondon, A. F., Cipriani, G., et al. (2009). Resistance to Plasmopara viticola in grapevine “Bianca” is controlled by a major dominant gene causing localised necrosis at the infection site. Theor. Appl. Genet. 120, 163–176. doi: 10.1007/s00122-009-1167-2
Bennett, R. A., and Lynch, J. M. (1981). Colonization potential of bacteria in the rhizosphere. Curr. Microbiol. 6, 137–138. doi: 10.1007/BF01642386
Bhattarai, G., Fennell, A., Londo, J. P., Coleman, C., and Kovacs, L. G. (2021). A novel grape downy mildew resistance locus from Vitis rupestris. Am. J. Enol. Vitic. 72, 12–20. doi: 10.5344/ajev.2020.20030
Blasi, P., Blanc, S., Wiedemann-Merdinoglu, S., Prado, E., Rühl, E. H., Mestre, P., et al. (2011). Construction of a reference linkage map of Vitis amurensis and genetic mapping of Rpv8, a locus conferring resistance to grapevine downy mildew. Theor. Appl. Genet. 123, 43–53. doi: 10.1007/s00122-011-1565-0
Borrás-Hidalgo, O., Thomma, B. P. H. J., Silva, Y., ChacÓn, O., and Pujol, M. (2010). Tobacco blue mould disease caused by Peronospora hyoscyami f. sp. tabacina. Mol. Plant Pathol. 11, 13–18. doi: 10.1111/j.1364-3703.2009.00569.x
Boutrot, F., and Zipfel, C. (2017). Function, discovery, and exploitation of plant pattern recognition receptors for broad-spectrum disease resistance. Annu. Rev. Phytopathol. 55, 257–286. doi: 10.1146/annurev-phyto-080614-120106
Brader, G., Compant, S., Vescio, K., Mitter, B., Trognitz, F., Ma, L. J., et al. (2017). Ecology and genomic insights into plant-pathogenic and plant-nonpathogenic endophytes. Annu. Rev. Phytopathol. 55, 61–83. doi: 10.1146/annurev-phyto-080516-035641
Brescia, F., Vlassi, A., Bejarano, A., Seidl, B., Marchetti-deschmann, M., Schuhmacher, R., et al. (2021). Characterisation of the antibiotic profile of Lysobacter capsici az78, an effective biological control agent of plant pathogenic microorganisms. Microorganisms 9, 1–17. doi: 10.3390/microorganisms9061320
Brilli, M., Asquini, E., Moser, M., Bianchedi, P. L., Perazzolli, M., and Si-Ammour, A. (2018). A multi-omics study of the grapevine-downy mildew (Plasmopara viticola) pathosystem unveils a complex protein coding- and noncoding-based arms race during infection. Sci. Rep. 8, 1–12. doi: 10.1038/s41598-018-19158-8
Brouwer, H., Coutinho, P. M., Henrissat, B., and de Vries, R. P. (2014). Carbohydrate-related enzymes of important Phytophthora plant pathogens. Fungal Genet. Biol. 72, 192–200. doi: 10.1016/J.FGB.2014.08.011
Bruisson, S., Maillot, P., Schellenbaum, P., Walter, B., Gindro, K., and Deglène-Benbrahim, L. (2016). Arbuscular mycorrhizal symbiosis stimulates key genes of the phenylpropanoid biosynthesis and stilbenoid production in grapevine leaves in response to downy mildew and grey mould infection. Phytochemistry 131, 92–99. doi: 10.1016/j.phytochem.2016.09.002
Burdziej, A., Bellée, A., Bodin, E., Valls Fonayet, J., Magnin, N., Szakiel, A., et al. (2021). Three types of elicitors induce grapevine resistance against downy mildew via common and specific immune responses. J. Agric. Food Chem. 69, 1781–1795. doi: 10.1021/acs.jafc.0c06103
Burruano, S. (2000). The life-cycle of Plasmopara viticola, cause of downy mildew of vine. Mycologist 14, 179–182. doi: 10.1016/S0269-915X(00)80040-3
Burruano, S., Alfonzo, A., Lo Piccolo, S., Conigliaro, G., Mondello, V., Torta, L., et al. (2008). Interaction between Acremonium byssoides and Plasmopara viticola in Vitis vinifera. Phytopathol. Mediterr. 47, 122–131. doi: 10.14601/Phytopathol_Mediterr-2615
Cadle-Davidson, L. (2008). Variation within and between Vitis spp. for foliar resistance to the downy mildew pathogen Plasmopara viticola. Plant Dis. 92, 1577–1584. doi: 10.1094/PDIS-92-11-1577
Campbell, S. E., Brannen, P. M., Scherm, H., Eason, N., and MacAllister, C. (2021). Efficacy of fungicide treatments for Plasmopara viticola control and occurrence of strobilurin field resistance in vineyards in Georgia, USA. Crop Prot. 139, 105371. doi: 10.1016/j.cropro.2020.105371
Casagrande, K., Falginella, L., Castellarin, S. D., Testolin, R., and Di Gaspero, G. (2011). Defence responses in Rpv3-dependent resistance to grapevine downy mildew. Planta. 234, 1097–1109. doi: 10.1007/s00425-011-1461-5
Chapagain, B. P., and Wiesman, Z. (2006). Phyto-saponins as a natural adjuvant for delivery of agromaterials through plant cuticle membranes. J. Agric. Food Chem. 54, 6277–6285. doi: 10.1021/jf060591y
Chen, J., Dai, G., Gu, Z., and Miao, Y. (2002). Inhibition effect of 58 plant extracts against grape downy mildew (Plasmopara viticola). Nat. Prod. Res. Dev. 14, 9–13.
Chen, M., Brun, F., Raynal, M., Debord, C., and Makowski, D. (2019). Use of probabilistic expert elicitation for assessing risk of appearance of grape downy mildew. Crop Prot. 126, 1–12. doi: 10.1016/j.cropro.2019.104926
Chen, T., Peng, J., Yin, X., Li, M., Xiang, G., Wang, Y., et al. (2021). Importin-αs are required for the nuclear localization and function of the Plasmopara viticola effector PvAVH53. Hortic. Res. 8. doi: 10.1038/s41438-021-00482-6
Chitarrini, G., Soini, E., Riccadonna, S., Franceschi, P., Zulini, L., Masuero, D., et al. (2017). Identification of biomarkers for defense response to Plasmopara viticola in a resistant grape variety. Front. Plant Sci. 8, 1–11. doi: 10.3389/fpls.2017.01524
Choi, Y. J., Hong, S. B., and Shin, H. D. (2005). A re-consideration of Pseudoperonospora cubensis and P. humuli based on molecular and morphological data. Mycol. Res. 109, 841–848. doi: 10.1017/S0953756205002534
Cimmino, A., Bejarano, A., Masi, M., Puopolo, G., and Evidente, A. (2020). Isolation of 2,5-diketopiperazines from Lysobacter capsici AZ78 with activity against Rhodococcus fascians. Nat. Prod. Res. 0, 1–9. doi: 10.1080/14786419.2020.1756803
Ciubotaru, R. M., Franceschi, P., Zulini, L., Stefanini, M., Škrab, D., Rossarolla, M. D., et al. (2021). Mono-locus and pyramided resistant grapevine cultivars reveal early putative biomarkers upon artificial inoculation with Plasmopara viticola. Front. Plant Sci. 12, 693887. doi: 10.3389/fpls.2021.693887
Clifford, D. P., and Bruyns-Haylett, J. P. (1978). The control of Plasmopara viticola with the 1:1 complex of zineb with 2-(2-aminoethylamino)ethanol and the potential effect of the use of this complex on ethylenethiourea residues. Pestic. Sci. 9, 493–495. doi: 10.1002/ps.2780090517
Coates, M. E., and Beynon, J. L. (2010). Hyaloperonospora arabidopsidis as a pathogen model. Annu. Rev. Phytopathol. 48, 329–345. doi: 10.1146/annurev-phyto-080508-094422
Cohen, Y., Reuveni, M., and Baider, A. (1999). Local and systemic activity of BABA (DL-3-aminobutyric acid) against Plasmopara viticola in grapevines. Eur. J. Plant Pathol. 105, 351–361. doi: 10.1023/A:1008734019040
Cohen, Y., Van Den Langenberg, K. M., Wehner, T. C., Ojiambo, P. S., Hausbeck, M., Quesada-Ocampo, L. M., et al. (2015). Resurgence of Pseudoperonospora cubensis: the causal agent of cucurbit downy mildew. Phytopathology 105, 998–1012. doi: 10.1094/PHYTO-11-14-0334-FI
Cohen, Y., Wang, W., Ben-Daniel, B.-H., and Ben-Daniel, Y. (2006). Extracts of Inula viscosa control downy mildew of grapes caused by Plasmopara viticola. Phytopathology 96, 417. doi: 10.1094/PHYTO-96-0417
Compant, S., Reiter, B., Sessitsch, A., Nowak, J., Clément, C., and Ait Barka, E. (2005). Endophytic Colonization of Vitis vinifera L. by Plant Growth- Promoting Bacterium Burkholderia sp. Strain PsJN. Appl. Environ. Microbiol. 71, 1685–1693. doi: 10.1128/AEM.71.4.1685-1693.2005
Constantinescu, O., and Fatehi, J. (2002). Peronospora - like fungi (Chromista, Peronosporales) parasitic on Brassicaceae and related hosts. Nov. Hedwigia 74, 291–338. doi: 10.1127/0029-5035/2002/0074-0291
Cooke, D. E. L., Drenth, A., Duncan, J. M., Wagels, G., and Brasier, C. M. (2000). A molecular phylogeny of Phytophthora and related oomycetes. Fungal Genet. Biol. 30, 17–32. doi: 10.1006/fgbi.2000.1202
Corina Vlot, A., Dempsey, D. A., and Klessig, D. F. (2009). Salicylic acid, a multifaceted hormone to combat disease. Annu. Rev. Phytopathol. 47, 177–206. doi: 10.1146/annurev.phyto.050908.135202
Corio-Costet, M.-F. (2011). Fungicide resistance in Plasmopara viticola in France and anti-resistance measures. Fungic. Resist. Crop Prot. Risk Manag., 157–171. doi: 10.1079/9781845939052.0157
Coutos-Thévenot, P., Poinssot, B., Bonomelli, A., Yean, H., Breda, C., Buffard, D., et al. (2001). In vitro tolerance to Botrytis cinerea of grapevine 41B rootstock in transgenic plants expressing the stilbene synthase Vst1 gene under the control of a pathogen-inducible PR 10 promoter. J. Exp. Bot. 52, 901–910. doi: 10.1093/jexbot/52.358.901
Cruz-Silva, A., Figueiredo, A., and Sebastiana, M. (2021). First insights into the effect of mycorrhizae on the expression of pathogen effectors during the infection of grapevine with Plasmopara viticola. Sustain. 13, 1–12. doi: 10.3390/su13031226
Dagostin, S., Formolo, T., Giovannini, O., Pertot, I., and Schmitt, A. (2010). Salvia officinalis extract can protect grapevine against Plasmopara viticola. Plant Dis. 94. doi: 10.1094/PDIS-94-5-0575
Dagostin, S., Schärer, H. J., Pertot, I., and Tamm, L. (2011). Are there alternatives to copper for controlling grapevine downy mildew in organic viticulture? Crop Prot. 30, 776–788. doi: 10.1016/j.cropro.2011.02.031
Dai, G., Andary, C., Mondolot-Cosson, L., and Boubals, D. (1995). Histochemical studies on the interaction between three species of grapevine, Vitis vinifera, V. rupestris and V. rotundifolia and the downy mildew fungus, Plasmopara viticola. Physiol. Mol. Plant Pathol. 46, 177–188. doi: 10.1006/pmpp.1995.1014
Darriet, P., Pons, M., Henry, R., Dumont, O., Findeling, V., Cartolaro, P., et al. (2002). Impact odorants contributing to the fungus type aroma from grape berries contaminated by powdery mildew (Uncinula necator); incidence of enzymatic activities of the yeast Saccharomyces cerevisiae. J. Agric. Food Chem. 50, 3277–3282. doi: 10.1021/jf011527d
De Bary, A. (1863). Recherches sur le developpement de quelques champignons parasites. Ann. des Sci. Nat. Bot. 4, 5–148.
De Oliveira Fialho, R., De Fátima, M., Papa, S., Panosso, A. R., Maria, A., and Cassiolato, R. (2016). Fungitoxicity of essential oils on Plasmopara viticola, causal agent of grapevine downy mildew. Rev. Bras. Frutic 39, 1–14. doi: 10.1590/0100-29452017015
Di Gaspero, G., Cipriani, G., Adam-Blondon, A. F., and Testolin, R. (2007). Linkage maps of grapevine displaying the chromosomal locations of 420 microsatellite markers and 82 markers for R-gene candidates. Theor. Appl. Genet. 114, 1249–1263. doi: 10.1007/S00122-007-0516-2
Dick, M. W. (1995). Sexual reproduction in the Peronosporomycetes (chromistan fungi). Can. J. Bot. 73, 712–724. doi: 10.1139/b95-314
Dick, M. W. (2002). Towards an understanding of the evolution of the downy mildews. Adv. Downy Mildew Res. 1–57. doi: 10.1007/0-306-47914-1_1
Dick, M. W., Vick, M. C., Gibbings, J. G., Hedderson, T. A., and Lopez Lastra, C. C. (1999). 18S rDNA for species of Leptolegnia and other Peronosporomycetes: justification for the subclass taxa Saprolegniomycetidae and Peronosporomycetidae and division of the Saprolegniaceae sensu lato into the Leptolegniaceae and Saprolegniaceae. Mycol. Res. 103, 1119–1125. doi: 10.1017/S0953756299008643
Dick, M. W., Wong, P. T. W., and Clark, G. (1984). The identity of the oomycete causing 'Kikuyu Yellows', with a reclassification of the downy mildews. Bot. J. Linn. Soc. 89, 171–197. doi: 10.1111/j.1095-8339.1984.tb01008.x
Díez-Navajas, A. M., Wiedemann-Merdinoglu, S., Greif, C., and Merdinoglu, D. (2008). Nonhost versus host resistance to the grapevine downy mildew, Plasmopara viticola, studied at the tissue level. Phytopathology 98, 776–780. doi: 10.1094/PHYTO-98-7-0776
Dubreuil-Maurizi, C., Trouvelot, S., Frettinger, P., Pugin, A., Wendehenne, D., and Poinssot, B. (2010). β-Aminobutyric acid primes an NADPH oxidase-dependent reactive oxygen species production during grapevine-triggered immunity. Mol. Plant Microbe Interact. 23, 1012–1021. doi: 10.1094/MPMI-23-8-1012
Dufour, M. C., and Corio-Costet, M.-F. (2012). “Comparison of phosphonate derivatives (fosétyl-Al, PK2) efficacy to that of BTH as grapevine defence elicitors against Plasmopara viticola,” in 6th International Workshop on Grapevine Downy and Powdery Mildew (Bordeaux), 7–9.
Dussert, Y., Gouzy, J., Richart-Cervera, S., Mazet, I. D., Delière, L., Couture, C., et al. (2016). Draft genome sequence of Plasmopara viticola, the grapevine downy mildew pathogen. Genome Announc. 4, 987–1003. doi: 10.1128/genomeA.00987-16
Dussert, Y., Mazet, I. D., Couture, C., Gouzy, J., Piron, M.-C., Kuchly, C., et al. (2019). A high-quality grapevine downy mildew genome assembly reveals rapidly evolving and lineage-specific putative host adaptation genes. Genome Biol. Evol. 11, 954–969. doi: 10.1093/gbe/evz048
Ellis, J. G., and Dodds, P. N. (2011). Showdown at the RXLR motif: serious differences of opinion in how effector proteins from filamentous eukaryotic pathogens enter plant cells. Proc. Natl. Acad. Sci. U.S.A. 108, 14381–14382. doi: 10.1073/pnas.1111668108
El-Sharkawy, H. H. A., Abo-El-Wafa, T. S. A., and Ibrahim, S. A. A. (2018). Biological control agents improve the productivity and induce the resistance against downy mildew of grapevine. J. Plant Pathol. 100, 33–42. doi: 10.1007/s42161-018-0007-0
Esmaeel, Q., Miotto, L., Rondeau, M., Leclère, V., Clément, C., Jacquard, C., et al. (2018). Paraburkholderia phytofirmans PsJN-plants interaction: from perception to the induced mechanisms. Front. Microbiol. 9, 1–14. doi: 10.3389/fmicb.2018.02093
Espinel-Ingroff, A. (2009). “Antifungal Agents,” in Encyclopedia of Microbiology, ed M. Schaechter (San Diego, CA: Elsevier Inc.), 205–222. doi: 10.1016/B978-012373944-5.00331-X
Exconde, O. R. (1976). Philippine corn downy mildew: assessment of present knowledge and future research needs. Kasetsart J. Nat. Sci. 10, 94–99.
Falk, S. P., Pearson, R. C., Gadoury, D., Seem, R. C., and Sztejnberg, A. (1996). Fusarium proliferatum as a biocontrol agent against grape downy mildew. Phytopathology 86, 1010–1017. doi: 10.1094/Phyto-86-1010
Figueiredo, A., Monteiro, F., Fortes, A. M., Bonow-Rex, M., Zyprian, E., Sousa, L., et al. (2012). Cultivar-specific kinetics of gene induction during downy mildew early infection in grapevine. Funct. Integr. Genomics 12, 379–386. doi: 10.1007/s10142-012-0261-8
Fraymouth, J. (1956). Haustoria of the peronosporales. Trans. Br. Mycol. Soc. 39, 79–107. doi: 10.1016/S0007-1536(56)80057-0
Frederiksen, R. A., and Renfro, B. L. (1977). Global status of maize downy mildew. Annu. Rev. Phytopathol. 15, 249–271. doi: 10.1146/annurev.py.15.090177.001341
Fröbel, S., and Zyprian, E. (2019). Colonization of different grapevine tissues by Plasmopara viticola- a histological study. Front. Plant Sci. 10, 1–13. doi: 10.3389/fpls.2019.00951
Fu, P., Wu, W., Lai, G., Li, R., Peng, Y., Yang, B., et al. (2020). Identifying Plasmopara viticola resistance Loci in grapevine (Vitis amurensis) via genotyping-by-sequencing-based QTL mapping. Plant Physiol. Biochem. 154, 75–84. doi: 10.1016/j.plaphy.2020.05.016
Furuya, S., Mochizuki, M., Aoki, Y., Kobayashi, H., Takayanagi, T., Shimizu, M., et al. (2011). Isolation and characterization of Bacillus subtilis KS1 for the biocontrol of grapevine fungal diseases. Biocontrol Sci. Technol. 21, 705–720. doi: 10.1080/09583157.2011.574208
Gabaston, J., Richard, T., Biais, B., Waffo-Teguo, P., Pedrot, É., Jourdes, M., et al. (2017a). Stilbenes from common spruce (Picea abies) bark as natural antifungal agent against downy mildew (Plasmopara viticola). Ind. Crops Prod. 103, 267–273. doi: 10.1016/j.indcrop.2017.04.009
Gabaston, J., Richard, T., Cluzet, S., Palos-Pinto, A., Dufour, M. C., Corio-Costet, M.-F., et al. (2017b). Pinus pinaster knot: a source of polyphenols against Plasmopara viticola. J. Agric. Food Chem. 65, 8884–8891. doi: 10.1021/acs.jafc.7b04129
Gasic, S., and Tanovic, B. (2013). Biopesticide formulations, possibility of application and future trends. Pestic. i fitomedicina 28, 97–102. doi: 10.2298/PIF1302097G
Gauthier, A., Trouvelot, S., Kelloniemi, J., Frettinger, P., Wendehenne, D., Daire, X., et al. (2014). The sulfated laminarin triggers a stress transcriptome before priming the SA-and ROS-dependent defenses during grapevine's induced resistance against Plasmopara viticola. PLoS ONE 9, e0088145. doi: 10.1371/journal.pone.0088145
Genet, J. L., Jaworska, G., and Deparis, F. (2006). Effect of dose rate and mixtures of fungicides on selection for QoI resistance in populations of Plasmopara viticola. Pest Manag. Sci. 62, 188–194. doi: 10.1002/ps.1146
Genet, J. L., and Vincent, O. (1999). Sensitivity of European Plasmopara viticola populations to cymoxanil. Pestic. Sci. 55, 129–136. doi: 10.1002/ps.2780550204
Gessler, C., Pertot, I., and Perazzolli, M. (2011). Plasmopara viticola: a review of knowledge on downy mildew of grapevine and effective disease management. Phytopathol. Mediterr. 50, 3–44. doi: 10.14601/Phytopathol_Mediterr-9360
Ghule, M. R., and Sawant, I. S. (2017). Potential of Fusarium spp. for biocontrol of downy mildew of grapes. Pest Manag. Hortic. Ecosyst. 23, 147–152.
Gisi, U. (2002). Chemical control of downy mildews. Adv. Downy Mildew Res. 119–159. doi: 10.1007/0-306-47914-1_4
Gisi, U., and Sierotzki, H. (2008). Fungicide modes of action and resistance in downy mildews. Eur. J. Plant Pathol. 122, 157–167. doi: 10.1007/s10658-008-9290-5
Gisi, U., Waldner, M., Kraus, N., Dubuis, P. H., and Sierotzki, H. (2007). Inheritance of resistance to carboxylic acid amide (CAA) fungicides in Plasmopara viticola. Plant Pathol. 56, 199–208. doi: 10.1111/j.1365-3059.2006.01512.x
Glazebrook, J. (2005). Contrasting mechanisms of defense against biotrophic and necrotrophic pathogens. Annu. Rev. Phytopathol. 43, 205–227. doi: 10.1146/annurev.phyto.43.040204.135923
Godard, S., Slacanin, I., Viret, O., and Gindro, K. (2009). Induction of defence mechanisms in grapevine leaves by emodin- and anthraquinone-rich plant extracts and their conferred resistance to downy mildew. Plant Physiol. Biochem. 47, 827–837. doi: 10.1016/j.plaphy.2009.04.003
Göker, M., and Stamatakis, A. (2006). “Maximum likelihood phylogenetic inference: an empirical comparison on a multi-locus dataset,” in The German Conference on Bioinformatics (Tübingen).
Göker, M., Voglmayr, H., Riethmüller, A., and Oberwinkler, F. (2007). How do obligate parasites evolve? A multi-gene phylogenetic analysis of downy mildews. Fungal Genet. Biol. 44, 105–122. doi: 10.1016/j.fgb.2006.07.005
Göker, M., Voglmayr, H., Riethmüller, A., Weiß, M., and Oberwinkler, F. (2003). Taxonomic aspects of Peronosporaceae inferred from Bayesian molecular phylogenetics. Can. J. Bot. 81, 672–683. doi: 10.1139/b03-066
Gómez-Zeledón, J., and Spring, O. (2018). Up-regulated RxLR effector genes of Plasmopara viticola in synchronized host-free stages and infected leaves of hosts with different susceptibility. Fungal Biol. 122, 1125–1133. doi: 10.1016/j.funbio.2018.07.006
Govrin, E. M., and Levine, A. (2000). The hypersensitive response facilitates plant infection by the necrotrophic pathogen Botrytis cinerea. Curr. Biol. 10, 751–757. doi: 10.1016/S0960-9822(00)00560-1
Gregory, C. (1915). “Studies on Plasmopara viticola (downey mildew of grapes),” in International Congress of Viticulture, July 12-13, 1915 (San Francisco, CA), 126–150.
Gullino, M. L., Tinivella, F., Garibaldi, A., Kemmitt, G. M., Bacci, L., and Sheppard, B. (2010). Mancozeb: past, present and future. Plant Dis. 94, 1077–1087. doi: 10.1094/PDIS-94-9-1076
Gullino, M. L., Mescalchin, E., and Mezzalama, M. (1997). Sensitivity to cymoxanil in populations of Plasmopara viticola in northern Italy. Plant Pathol. 46, 729–736. doi: 10.1046/j.1365-3059.1997.d01-68.x
Gupta, R. M., and Musunuru, K. (2014). Expanding the genetic editing tool kit: ZFNs, TALENs, and CRISPR-Cas9. J. Clin. Invest. 124, 4154–4161. doi: 10.1172/JCI72992
Gustavsson, A. (1987). Peronospora on two Cerastium species in northern Europe. Mycotaxon 30, 133–148.
Haas, B. J., Kamoun, S., Zody, M. C., Jiang, R. H. Y., Handsaker, R. E., Cano, L. M., et al. (2009). Genome sequence and analysis of the Irish potato famine pathogen Phytophthora infestans. Nature 461, 393–398. doi: 10.1038/nature08358
Halim, V. A., Altmann, S., Ellinger, D., Eschen-Lippold, L., Miersch, O., Scheel, D., et al. (2009). PAMP-induced defense responses in potato require both salicylic acid and jasmonic acid. Plant J. 57, 230–242. doi: 10.1111/j.1365-313X.2008.03688.x
Hall, G. S. (1996). Modern approaches to species concepts in downy mildews. Plant Pathol. 45, 1009–1026. doi: 10.1046/j.1365-3059.1996.d01-191.x
Hall, G. S., and Humphreys-Jones, D. R. (1989). Peronospora on Phygelius aequalis compared with Peronospora sordida and Peronospora verbasci. Plant Pathol. 38, 103–110. doi: 10.1111/j.1365-3059.1989.tb01435.x
Halleen, F., Fourie, P. H., and Lombard, P. J. (2016). Protection of grapevine pruning wounds against Eutypa lata by biological and chemical methods. South Afr. J. Enol. Vitic. 31, 125–132. doi: 10.21548/31-2-1409
Hallin, S., Philippot, L., Löffler, F. E., Sanford, R. A., and Jones, C. M. (2018). Genomics and ecology of novel N2O-reducing microorganisms. Trends Microbiol. 26, 43–55. doi: 10.1016/j.tim.2017.07.003
Hamaoka, K., Aoki, Y., and Suzuki, S. (2021). Isolation and characterization of endophyte Bacillus velezensis KOF112 from grapevine shoot xylem as biological control agent for fungal Diseases. 10, 1–18. doi: 10.3390/plants10091815
Hao, Z., Van Tuinen, D., Wipf, D., Fayolle, L., Chataignier, O., Li, X., et al. (2017). Biocontrol of grapevine aerial and root pathogens by Paenibacillus sp. strain B2 and paenimyxin in vitro and in planta. Biol. Control 109, 42–50. doi: 10.1016/j.biocontrol.2017.03.004
Haran, S., Schickler, H., and Chet, I. (1996). Molecular mechanisms of lytic enzymes involved in the biocontrol activity of Trichoderma harzianum. Microbiology 142, 2321–2331. doi: 10.1099/00221287-142-9-2321
Harm, A., Kassemeyer, H.-H., Seibicke, T., and Regner, F. (2011). Evaluation of chemical and natural resistance inducers against downy mildew (Plasmopara viticola) in grapevine. Am. J. Enol. Vitic. 62, 184–192. doi: 10.5344/ajev.2011.09054
Héloir, M.-C., Adrian, M., Brulé, D., Claverie, J., Cordelier, S., Daire, X., et al. (2019). Recognition of elicitors in grapevine: from MAMP and DAMP perception to induced resistance. Front. Plant Sci. 10, 1–17. doi: 10.3389/fpls.2019.01117
Hendrickx, F. L. (1948). Sylloge ungorum congensium. Catalogue des champignons signales au Congo Belge et au Ruanda-Urundi. Ser. Sci. 35, 1–224.
Hernández, J. A., Gullner, G., Clemente-Moreno, M. J., Künstler, A., Juhász, C., Díaz-Vivancos, P., et al. (2016). Oxidative stress and antioxidative responses in plant-virus interactions. Physiol. Mol. Plant Pathol. 94, 134–148. doi: 10.1016/j.pmpp.2015.09.001
Hofmann, U. J. H., and Kauer, R. (2008). Can inorganic-Potassium-phosphonate reduce the use of copper in organic viticulture without causing residue problems? Int. Consult. Org. Vitic. 1–20.
Holub, E. B. (2008). Natural history of Arabidopsis thaliana and oomycete symbioses. Eur. J. Plant Pathol. 122, 91–109. doi: 10.1007/978-1-4020-8973-2_8
Huang, G., Liu, Z., Gu, B., Zhao, H., Jia, J., Fan, G., et al. (2019). An RXLR effector secreted by Phytophthora parasitica is a virulence factor and triggers cell death in various plants. Mol. Plant Pathol. 20, 356–371. doi: 10.1111/MPP.12760
Huibers, R. P., De Jong, M., Dekter, R. W., and Van Den Ackerveken, G. (2009). Disease-specific expression of host genes during downy mildew infection of arabidopsis. Mol. Plant Microbe Interact. 22, 1104–1115. doi: 10.1094/MPMI-22-9-1104
Jackson, R. S. (2000). Wine Science: Principles, Practice, Perception, 2nd Edn. Elsevier. doi: 10.1016/B978-0-12-373646-8.50004-4
James, E. E. (2018). Development of an organic botanical plant protection product from Larix by-products (dissertation). Natural Products Research Group, University of Surrey, Surrey, Guildford, United Kingdom.
Javed, M. R., Ijaz, A., Shahid, M., Nadeem, H., Shokat, Z., and Raziq, A. (2021). “Chapter 16 - Fungal genome editing using CRISPR-Cas nucleases: a new tool for the management of plant diseases,” in Nanobiotechnology for Plant Protection, eds K. A. Abd-Elsalam and K.-T. B. T.-C. and Rna. S. Lim (Elsevier), 333–360. doi: 10.1016/B978-0-12-821910-2.00001-1
Jeger, M., Gilijamse, E., Bock, C. H., and Frinking, H. D. (1998). The epidemiology, variability and control of the downy mildews of pearl millet and sorghum, with particular reference to Africa. Plant Pathol. 47, 544–569. doi: 10.1046/j.1365-3059.1998.00285.x
Jermini, M., Blaise, P., and Gessler, C. (2010a). Influence of Plasmopara viticola on gas exchange parameters on field-grown Vitis vinifera “Merlot.” Vitis 49, 87–93.
Jermini, M., Blaise, P., and Gessler, C. (2010b). Quantification of the influence of the downy mildew (Plasmopara viticola) epidemics on the compensatory capacities of Vitis vinifera “Merlot” to limit the qualitative yield damage. Vitis J. Grapevine Res. 49, 153–160.
Johnson, G. I. (1989). Peronospora hyoscyami de Bary: taxonomic history, strains, and host range. Blue Mold Tob. 1–18.
Jones, J. D. G., and Dangl, J. L. (2006). The plant immune system. Nature 444, 323–329. doi: 10.1038/nature05286
Joshi, M. M. (2003). “Fungicides, cymoxanil.,” in Encyclopedia of Agrochemicals, Vol. 1, ed J. R. Plimmer (Hoboken, NJ: John Wiley & Sons, Inc.), 554–555. doi: 10.1002/047126363X.agr095
Kale, S. D., and Tyler, B. M. (2011). Entry of oomycete and fungal effectors into plant and animal host cells. Cell. Microbiol. 13, 1839–1848. doi: 10.1111/j.1462-5822.2011.01659.x
Kamble, M. V., Joshi, S. M., Hadimani, S., and Jogaiah, S. (2021). Biopriming with rhizosphere Trichoderma harzianum elicit protection against grapevine downy mildew disease by triggering histopathological and biochemical defense responses. Rhizosphere 19, 100398. doi: 10.1016/j.rhisph.2021.100398
Kamoun, S. (2006). A catalogue of the effector secretome of plant pathogenic oomycetes. Annu. Rev. Phytopathol. 44, 41–60. doi: 10.1146/annurev.phyto.44.070505.143436
Kamoun, S., Furzer, O., Jones, J. D. G., Judelson, H. S., Ali, G. S., Dalio, R. J. D., et al. (2015). The Top 10 oomycete pathogens in molecular plant pathology. United States Mol. PLANT Pathol. 16, 413–434. doi: 10.1111/mpp.12190
Kandel, S. L., Mou, B., Shishkoff, N., Shi, A., Subbarao, K. V., and Klosterman, S. J. (2019). Spinach downy mildew: advances in our understanding of the disease cycle and prospects for disease management. Plant Dis. 103, 791–803. doi: 10.1094/PDIS-10-18-1720-FE
Kast, W. K. (2001). “Untersuchungen zur Wirksamkeit von Pflanzenextrakten gegen den Erreger des Falschen Mehltaus der Weinrebe (Plasmopara viticola),” in Probleme und Lösungsansätze - Siebtes Fachgespräch “Alternativen zur Anwendung von Kupfer als Pflanzenschutzmittel” (Berlin), 44–47.
Keller, B., Feuillet, C., and Messmer, M. (2000). “Genetics of disease resistance: basic concepts and application in resistance breeding,” in Mechanisms of Resistance to Plant Diseases 101–160. doi: 10.1007/978-94-011-3937-3_5
Kim Khiook, I. L., Schneider, C., Heloir, M. C., Bois, B., Daire, X., Adrian, M., et al. (2013). Image analysis methods for assessment of H2O2 production and Plasmopara viticola development in grapevine leaves: application to the evaluation of resistance to downy mildew. J. Microbiol. Methods 95, 235–244. doi: 10.1016/j.mimet.2013.08.012
Kim, J. Y., Moon, J.-C., Kim, H. C., Shin, S., Song, K., Kim, K.-H., et al. (2017). Identification of downy mildew resistance gene candidates by positional cloning in maize (Zea mays subsp. mays; Poaceae). Appl. Plant Sci. 5, 1–7. doi: 10.3732/apps.1600132
Koornneef, A., and Pieterse, C. M. J. (2008). Cross talk in defense signaling. Plant Physiol. 146, 839–844. doi: 10.1104/pp.107.112029
Kortekamp, A. (1997). Epicoccum nigrum link: a biological control agent of Plasmopara viticola (Berk et Curt.) Berl. et De Toni? Vitis 36, 215–216.
Kortekamp, A. (2006). Expression analysis of defence-related genes in grapevine leaves after inoculation with a host and a non-host pathogen. Plant Physiol. Biochem. 44, 58–67. doi: 10.1016/j.plaphy.2006.01.008
Kushalappa, A. C., Yogendra, K. N., and Karre, S. (2016). Plant innate immune response: qualitative and quantitative resistance. CRC Crit. Rev. Plant Sci. 35, 38–55. doi: 10.1080/07352689.2016.1148980
La Torre, A., Mandalà, C., Pezza, L., Caradonia, F., and Battaglia, V. (2014). Evaluation of essential plant oils for the control of Plasmopara viticola. J. Essent. Oil Res. 26, 282–291. doi: 10.1080/10412905.2014.889049
La Torre, A., Righi, L., Iovino, V., and Battaglia, V. (2019). Evaluation of copper alternative products to control grape downy mildew in organic farming. J. Plant Pathol. 101, 1005–1012. doi: 10.1007/s42161-019-00330-6
La Torre, A., Talocci, S., Spera, G., and Valori, R. (2008). Control of downy mildew on grapes in organic viticulture. Commun. Agric. Appl. Biol. Sci. 73, 169–178.
Lakkis, S., Trotel-Aziz, P., Rabenoelina, F., Schwarzenberg, A., Nguema-Ona, E., Clément, C., et al. (2019). Strengthening grapevine resistance by Pseudomonas fluorescens PTA-CT2 relies on distinct defense pathways in susceptible and partially resistant genotypes to downy mildew and gray mold diseases. Front. Plant Sci. 10, 1–18. doi: 10.3389/fpls.2019.01112
Lamour, K., and Kamoun, S. (2009). Oomycete Genetics and Genomics: Diversity, Interactions and Research Tools. Hoboken, NJ: John Wiley & Sons, Inc. p. 1–574. doi: 10.1002/9780470475898
Lan, X., Liu, Y., Song, S., Yin, L., Xiang, J., Qu, J., et al. (2019). Plasmopara viticola effector PvRXLR131 suppresses plant immunity by targeting plant receptor-like kinase inhibitor BKI1. Mol. Plant Pathol. 20, 765–783. doi: 10.1111/mpp.12790
Lange, L., Olson, L. W., and Safeeulla, K. M. (1984). Pearl millet downy mildew (Sclerospora graminicola): zoosporogenesis. Protoplasma 119, 178–187. doi: 10.1007/BF01288872
Lazazzara, V., Vicelli, B., Bueschl, C., Parich, A., Pertot, I., Schuhmacher, R., et al. (2021). Trichoderma spp. volatile organic compounds protect grapevine plants by activating defense-related processes against downy mildew. Physiol. Plant. 172, 1950–1965. doi: 10.1111/ppl.13406
Lebeda, A. (1986). Epidemic occurrence of Pseudoperonospora cubensis in Czechoslovakia. Temp. Downy Mildews Newsl. 4, 15–17.
Lebeda, A. (2002). Occurrence and variation in virulence of Bremia Lactucae in natural populations of Lactuca Serriola. Adv. Downy Mildew Res. 179–183. doi: 10.1007/0-306-47914-1_8
Lebeda, A., and Schwinn, F. J. (1994). The downy mildews - an overview of recent research progress. J. Plant Dis. Prot. 101, 225–254.
Lebeda, A., Spencer-Phillips, P. T. N., and Cooke, B. M. (2008). The downy mildews - genetics, molecular biology and control. Euro. J. Plant Pathol. 122, 1–207. doi: 10.1007/s10658-008-9324-z
Leroux, P., Chapeland, F., Desbrosses, D., and Gredt, M. (1999). Patterns of cross-resistance to fungicides in Botryotinia fuckeliana (Botrytis cinerea) isolates from French vineyards. Crop Prot. 18, 687–697. doi: 10.1016/S0261-2194(99)00074-5
Leroy, P., Smits, N., Cartolaro, P., Delière, L., Goutouly, J.-P., Raynal, M., et al. (2013). A bioeconomic model of downy mildew damage on grapevine for evaluation of control strategies. Crop Prot. 53, 58–71. doi: 10.1016/j.cropro.2013.05.024
Li, X., Wu, J., Yin, L., Zhang, Y., Qu, J., and Lu, J. (2015). Comparative transcriptome analysis reveals defense-related genes and pathways against downy mildew in Vitis amurensis grapevine. Plant Physiol. Biochem. 95, 1–14. doi: 10.1016/j.plaphy.2015.06.016
Li, X., Yang, H., Roy, B., Park, E. Y., Jiang, L., Wang, D., et al. (2010). Enhanced cellulase production of the Trichoderma viride mutated by microwave and ultraviolet. Microbiol. Res. 165, 190–198. doi: 10.1016/j.micres.2009.04.001
Liang, C., Zang, C., McDermott, M. I., Zhao, K., Yu, S., and Huang, Y. (2016). Two imide substances from a soil-isolated Streptomyces atratus strain provide effective biocontrol activity against grapevine downy mildew. Biocontrol Sci. Technol. 26, 1337–1351. doi: 10.1080/09583157.2016.1199014
Lim, S. M. (1989). Inheritance of resistance to Peronospora manshurica Races 2 and 33 in soybean. Phytopathology 79, 877–879. doi: 10.1094/Phyto-79-877
Lin, H., Leng, H., Guo, Y., Kondo, S., Zhao, Y., Shi, G., et al. (2019). QTLs and candidate genes for downy mildew resistance conferred by interspecific grape (V. vinifera L. × V. amurensis Rupr.) crossing. Sci. Hortic. 244, 200–207. doi: 10.1016/j.scienta.2018.09.045
Liu, R., Chen, T., Yin, X., Xiang, G., Peng, J., Fu, Q., et al. (2021). A Plasmopara viticola RXLR effector targets a chloroplast protein PsbP to inhibit ROS production in grapevine. Plant J. 106, 1557–1570. doi: 10.1111/tpj.15252
Liu, S., li, Wu, J., Zhang, P., Hasi, G., Huang, Y., Lu, J., et al. (2016). Response of phytohormones and correlation of SAR signal pathway genes to the different resistance levels of grapevine against Plasmopara viticola infection. Plant Physiol. Biochem. 107, 56–66. doi: 10.1016/j.plaphy.2016.05.020
Lizzi, Y., Coulomb, C., Claude, P., Coulomb, P. J., and Coulomb, P. O. (1998). L'algue face au mildiou: quel avenir? Des résultats de laboratoire très encourageants. Phytoma la défense des végétaux 508, 29–30.
Lo Piccolo, S., Alfonzo, A., Giambra, S., Conigliaro, G., Lopez-Llorca, L. V., and Burruano, S. (2015). Identification of Acremonium isolates from grapevines and evaluation of their antagonism towards Plasmopara viticola. Ann. Microbiol. 65, 2393–2403. doi: 10.1007/s13213-015-1082-5
Locato, V., and De Gara, L. (2018). Programmed cell death in plants: an overview. Methods Mol. Biol. 1743, 1–8. doi: 10.1007/978-1-4939-7668-3_1
Lucas, J. A., Hawkins, N. J., and Fraaije, B. A. (2015). The evolution of fungicide resistance. Adv. Appl. Microbiol. 90, 29–92. doi: 10.1016/bs.aambs.2014.09.001
Lukas, K., Innerebner, G., Kelderer, M., Finckh, M. R., and Hohmann, P. (2016). Efficacy of copper alternatives applied as stop-sprays against Plasmopara viticola in grapevine. J. Plant Dis. Prot. 123, 171–176. doi: 10.1007/s41348-016-0024-1
Lyon, R., Correll, J., Feng, C., Bluhm, B., Shrestha, S., Shi, A., et al. (2016). Population structure of peronospora effusa in the southwestern United States. PLoS ONE 11, 1–10. doi: 10.1371/journal.pone.0148385
Madden, L. V., Ellis, M. A., and Wilson, L. L. (2000). Evaluation of a disease warning system for downy mildew of grapes. Plant Dis. 84, 549–554. doi: 10.1094/PDIS.2000.84.5.549
Magarey, P. A., Wachtel, M. F., and Newton, M. R. (1991). Evaluation of phosphonate, fosetyl-Al and several phenylamide fungicides for post-infection control of grapevine downy mildew caused by Plasmopara viticola. Australas. Plant Pathol. 20, 34–40. doi: 10.1071/APP9910034
Maia, A. J., Oliveira, J. S. B., Schwan-Estrada, K. R. F., Faria, C. M. R., Batista, A. F., Costa, W. F., et al. (2014). The control of isariopsis leaf spot and downy mildew in grapevine cv. Isabel with the essential oil of lemon grass and the activity of defensive enzymes in response to the essential oil. Crop Prot. 63, 57–67. doi: 10.1016/j.cropro.2014.05.005
Malacarne, G., Vrhovsek, U., Zulini, L., Cestaro, A., Stefanini, M., Mattivi, F., et al. (2011). Resistance to Plasmopara viticola in a grapevine segregating population is associated with stilbenoid accumulation and with specific host transcriptional responses. BMC Plant Biol. 11, 1–13. doi: 10.1186/1471-2229-11-114
Marchand, P. A. (2018). Lecithins: a food additive valuable for antifungal crop protection. Int. J. Econ. Plants 5, 104–107. doi: 10.23910/IJEP/2018.5.3.0243
Marchive, C., Léon, C., Kappel, C., Coutos-Thévenot, P., Corio-Costet, M. F., Delrot, S., et al. (2013). Over-expression of VvWRKY1 in grapevines induces expression of jasmonic acid pathway-related genes and confers higher tolerance to the downy mildew. PLoS ONE 8, 1–8. doi: 10.1371/journal.pone.0054185
Marguerit, E., Boury, C., Manicki, A., Donnart, M., Butterlin, G., Némorin, A., et al. (2009). Genetic dissection of sex determinism, inflorescence morphology and downy mildew resistance in grapevine. Theor. Appl. Genet. 118, 1261–1278. doi: 10.1007/s00122-009-0979-4
Massi, F., Torriani, S. F. F., Borghi, L., and Toffolatti, S. L. (2021). Fungicide resistance evolution and detection in plant pathogens: Plasmopara viticola as a case study. Microorganisms 9, 1–18. doi: 10.3390/microorganisms9010119
Merdinoglu, D., Schneider, C., Prado, E., Wiedemann-Merdinoglu, S., and Mestre, P. (2018). Breeding for durable resistance to downy and powdery mildew in grapevine. Oeno One 52, 189–195. doi: 10.20870/oeno-one.2018.52.3.2116
Merdinoglu, D., Wiedemann-Merdinoglu, S., Coste, P., Dumas, V., Haetty, S., Butterlin, G., et al. (2003). “Genetic analysis of downy mildew resistance derived from Muscadinia rotundifolia,” in Eighth International Conference on Grape Genetics and Breeding (Kecskemet), 1–6. doi: 10.17660/ActaHortic.2003.603.57
Mestre, P., Carrere Cd, S., Gouzy, J., Piron, M.-C., Tourvieille De Labrouhe, D., Vincourt, P., et al. (2016). Comparative analysis of expressed CRN and RXLR effectors from two Plasmopara species causing grapevine and sunflower downy mildew. Plant Pathol. 65, 767–781. doi: 10.1111/ppa.12469
Mestre, P., Piron, M. C., and Merdinoglu, D. (2012). Identification of effector genes from the phytopathogenic Oomycete Plasmopara viticola through the analysis of gene expression in germinated zoospores. Fungal Biol. 116, 825–835. doi: 10.1016/j.funbio.2012.04.016
Michelmore, R. W. (1981). Sexual and Asexual Sporulation in the Downy Mildews (Pathogenic Fungi). Available online at: https://agris.fao.org/agris-search/search.do?recordID=US8244895 (accessed August 19, 2020).
Millardet, A. (1885). Traitement du mildiou par le mélange de sulphate de cuivre et chaux. J. Agric. Prat. 49, 707–710.
Miotto-Vilanova, L., Jacquard, C., Courteaux, B., Wortham, L., Michel, J., Clément, C., et al. (2016). Burkholderia phytofirmans PsJN confers grapevine resistance against Botrytis cinerea via a direct antimicrobial effect combined with a better resource mobilization. Front. Plant Sci. 7, 1–15. doi: 10.3389/fpls.2016.01236
Money, N. P. (1998). Why oomycetes have not stopped being fungi. Mycol. Res. 102, 767–768. doi: 10.1017/S095375629700556X
Mulholland, D. A., Thuerig, B., Langat, M. K., Tamm, L., Nawrot, D. A., James, E., et al. (2017). Efficacy of extracts from eight economically important forestry species against grapevine downy mildew (Plasmopara viticola) and identification of active constituents. Crop Prot. 102, 104–109. doi: 10.1016/j.cropro.2017.08.018
Müller, K. (1938). Entwicklung der Reben-Peronospora- bekämpfung in Baden. Nachrichtenblatt des Dtsch. Pflanzenschutzdienstes 12, 195–205.
Musetti, R., Vecchione, A., Stringher, L., Borselli, S., Zulini, L., Marzani, C., et al. (2006). Inhibition of sporulation and ultrastructural alterations of grapevine downy mildew by the endophytic fungus Alternaria alternata. Phytopathology 96, 689. doi: 10.1094/PHYTO-96-0689
Mysore, K. S., and Ryu, C. M. (2004). Nonhost resistance: how much do we know? Trends Plant Sci. 9, 97–104. doi: 10.1016/j.tplants.2003.12.005
Nanni, I. M., Pirondi, A., Contaldo, N., and Collina, M. (2016). Screening of sensitivity to mandipropamid of Plasmopara viticola populations from Italian vineyards by molecular and biological methods. Lett. Appl. Microbiol. 63, 268–273. doi: 10.1111/lam.12613
Nascimento, R., Maia, M., Ferreira, A. E. N., Silva, A. B., Freire, A. P., Cordeiro, C., et al. (2019). Early stage metabolic events associated with the establishment of Vitis vinifera—Plasmopara viticola compatible interaction. Plant Physiol. Biochem. doi: 10.1016/j.plaphy.2019.01.026
Nicholas, P., Magarey, P. A., and Wachtel, M. F. (1994). Diseases and Pests. Marleston, SA: Winetitles.
Northover, J., and Ripley, B. D. (1980). Persistence of chlorothalonil on grapes and its effect on disease control and fruit quality. J. Agric. Food Chem. 28, 971–974. doi: 10.1021/jf60231a005
O'Connell, R. J., and Panstruga, R. (2006). Tete a tete inside a plant cell: establishing compatibility between plants and biotrophic fungi and oomycetes. New Phytol. 171, 699–718. doi: 10.1111/j.1469-8137.2006.01829.x
O'Neill, T. M., Elad, Y., Shtienberg, D., and Cohen, A. (1996). Control of grapevine grey mould with Trichoderma harzianum T39. Biocontrol Sci. Technol. 6, 139–146. doi: 10.1080/09583159650039340
Palla, O. (2006). A new formulation based on fatty acids coming from vegetal oils effective against grapevine downy mildew. ATTI Giornate Fitopatol. II, 2, 17–20.
Palmieri, M. C., Perazzolli, M., Matafora, V., Moretto, M., Bachi, A., and Pertot, I. (2012). Proteomic analysis of grapevine resistance induced by Trichoderma harzianum T39 reveals specific defence pathways activated against downy mildew. J. Exp. Bot. 63, 6237–6251. doi: 10.1093/jxb/ers279
Palti, J., and Cohen, Y. (1980). Downy mildew of Cucurbits (Pseudoperonospora cubensis): the fungus and its hosts, distribution, epidemiology and control. Phytoparasitica 8, 109–147. doi: 10.1007/BF02994506
Paris, F., Krzyzaniak, Y., Gauvrit, C., Jamois, F., Domergue, F., Joubès, J., et al. (2016). An ethoxylated surfactant enhances the penetration of the sulfated laminarin through leaf cuticle and stomata, leading to increased induced resistance against grapevine downy mildew. Physiol. Plant. 156, 338–350. doi: 10.1111/ppl.12394
Parveaud, C. E., Gomez, C., Lambion, J., Dagostin, S., and Pertot, I. (2010). “Alternatives to copper-based treatments for the control of grapevine downy mildew (Plasmopara viticola): 5-year synthesis of trials in France and Italy,” in 28th International Horticultural Congress (Lisbon).
Patil, N. P. (2015). Management of Downy Mildew of Grape Caused by Plasmopara viticola With New Formulation of Fungicides and Plant Strengtheners. Available online at: https://krishikosh.egranth.ac.in/handle/1/5810051087
Payak, M. M. (1975). Epidemiology of maize downy mildews with special reference to those occurring in Asia. Trop. Agric. Res. 8, 81–91.
Perazzolli, M., Dagostin, S., Ferrari, A., Elad, Y., and Pertot, I. (2008). Induction of systemic resistance against Plasmopara viticola in grapevine by Trichoderma harzianum T39 and benzothiadiazole. Biol. Control 47, 228–234. doi: 10.1016/j.biocontrol.2008.08.008
Perazzolli, M., Moretto, M., Fontana, P., Ferrarini, A., Velasco, R., Moser, C., et al. (2012). Downy mildew resistance induced by Trichoderma harzianum T39 in susceptible grapevines partially mimics transcriptional changes of resistant genotypes. BMC Genom. 13, 1–19. doi: 10.1186/1471-2164-13-660
Perazzolli, M., Roatti, B., Bozza, E., and Pertot, I. (2011). Trichoderma harzianum T39 induces resistance against downy mildew by priming for defense without costs for grapevine. Biol. Control 58, 74–82. doi: 10.1016/j.biocontrol.2011.04.006
Perez, L., Rodriguez, M. E., Rodriguez, F., and Roson, C. (2003). Efficacy of acibenzolar-S-methyl, an inducer of systemic acquired resistance against tobacco blue mould caused by Peronospora hyoscyami f. sp. tabacina. Crop Prot. 22, 405–413. doi: 10.1016/S0261-2194(02)00198-9
Petatán-Sagahón, I., Anducho-Reyes, M. A., Silva-Rojas, H. V., Arana-Cuenca, A., Tellez-Jurado, A., Cárdenas-Álvarez, I. O., et al. (2011). Isolation of bacteria with antifungal activity against the phytopathogenic fungi Stenocarpella maydis and Stenocarpella macrospora. Int. J. Mol. Sci. 12, 5522–5537. doi: 10.3390/ijms12095522
Pezet, R., Gindro, K., Viret, O., and Richter, H. (2004). Effects of resveratrol, viniferins and pterostilbene on Plasmopara viticola zoospore mobility and disease development. Vitis 43, 145–148.
Pezet, R., Perret, C., Jean-Denis, J. B., Tabacchi, R., Gindro, K., and Viret, O. (2003). δ-viniferin, a resveratrol dehydrodimer: one of the major stilbenes synthesized by stressed grapevine leaves. J. Agric. Food Chem. 51, 5488–5492. doi: 10.1021/jf030227o
Pieterse, C. M. J., Van Wees, S. C. M., Hoffland, E., Van Pelt, J. A., and Van Loon, L. C. (1996). Systemic resistance in Arabidopsis induced by biocontrol bacteria is independent of salicylic acid accumulation and pathogenesis-related gene expression. Plant Cell 8, 1225–1237. doi: 10.1105/tpc.8.8.1225
Pietrzak, U., and McPhail, D. C. (2004). Copper accumulation, distribution and fractionation in vineyard soils of Victoria, Australia. Geoderma 151–166. doi: 10.1016/j.geoderma.2004.01.005
Pinto, C., Pinho, D., Sousa, S., Pinheiro, M., and Egas, C. (2014). Unravelling the diversity of grapevine microbiome. PLoS ONE 9, 85622. doi: 10.1371/journal.pone.0085622
Polesani, M., Bortesi, L., Ferrarini, A., Zamboni, A., Fasoli, M., Zadra, C., et al. (2010). General and species-specific transcriptional responses to downy mildew infection in a susceptible (Vitis vinifera) and a resistant (V. riparia) grapevine species. BMC Genomics 11, 1–16. doi: 10.1186/1471-2164-11-117
Possamai, T., Migliaro, D., Gardiman, M., Velasco, R., and De Nardi, B. (2020). Rpv mediated defense responses in grapevine offspring resistant to Plasmopara viticola. Plants 9, 1–10. doi: 10.3390/plants9060781
Puopolo, G., Cimmino, A., Palmieri, M. C., Giovannini, O., Evidente, A., and Pertot, I. (2014a). Lysobacter capsici AZ78 produces cyclo(l-Pro-l-Tyr), a 2,5-diketopiperazine with toxic activity against sporangia of Phytophthora infestans and Plasmopara viticola. J. Appl. Microbiol. 117, 1168–1180. doi: 10.1111/jam.12611
Puopolo, G., Giovannini, O., and Pertot, I. (2014b). Lysobacter capsici AZ78 can be combined with copper to effectively control Plasmopara viticola on grapevine. Microbiol. Res. 169, 633–642. doi: 10.1016/j.micres.2013.09.013
Puopolo, G., Tomada, S., Sonego, P., Moretto, M., Engelen, K., Perazzolli, M., et al. (2016). The Lysobacter capsici AZ78 genome has a gene pool enabling it to interact successfully with phytopathogenic microorganisms and environmental factors. Front. Microbiol. 7, 1–15. doi: 10.3389/fmicb.2016.00096
Putnam, M. L. (2007). Brown stripe downy mildew (Sclerophthora rayssiae var. zeae) of Maize. Plant Heal. Prog. 8, 1–6. doi: 10.1094/PHP-2007-1108-01-DG
Ramseyer, J. (2018). Natural products with antifungal properties as alternative to copper in agriculture [dissertation]. Faculty of Philosophy and Natural Sciences, University of Basel, Basel, Switzerland. p. 1–303.
Rehmany, A. P., Gordon, A., Rose, L. E., Allen, R. L., Armstrong, M. R., Whisson, S. C., et al. (2005). Differential recognition of highly divergent downy mildew avirulence gene alleles by RPP1 resistance genes from two Arabidopsis lines. Plant Cell 17, 1839–1850. doi: 10.1105/tpc.105.031807
Reino, J. L., Guerrero, R. F., Hernández-Galán, R., and Collado, I. G. (2008). Secondary metabolites from species of the biocontrol agent Trichoderma. Phytochem Rev 7, 89–123. doi: 10.1007/s11101-006-9032-2
Rekanovic, E., Potocnik, I., Stepanovic, M., Milijasevic, S., and Todorovic, B. (2008). Field efficacy of fluopicolide and fosetyl-Al fungicide combination (Profiler®) for control of Plasmopara viticola (Berk. & Curt.) Berl. & Toni. in grapevine. Pestic. i fitomedicina 23, 183–187. doi: 10.2298/PIF0803183R
Reuveni, M. (1998). Relationships between leaf age, peroxidase and p-1,3-glucanase activity, and resistance to downy mildew in grapevines. Phytopathol. Zeitschrift 146, 525–530. doi: 10.1111/j.1439-0434.1998.tb04615.x
Reuveni, M. (2001). Activity of trifloxystrobin against powdery and downy mildew diseases of grapevines. Can. J. Plant Pathol. 23, 52–59. doi: 10.1080/07060660109506909
Reuveni, M. (2014). Post-infection applications of K3PO3, phosphorous acid and dimethomorph inhibit development of downy mildew caused by Plasmopara viticola on grapevines. J. Small Fruit Vitic. 5, 27–38. doi: 10.1300/J065v05n02_03
Reuveni, M., Zahavi, T., and Cohen, Y. (2001). Controlling downy mildew (Plasmopara viticola) in field-grown grapevine with β-aminobutyric acid (BABA). Phytoparasitica 29, 125–133. doi: 10.1007/BF02983956
Rienth Id, M., Crovadore, J., Ghaffari, S., and Ois Lefort, F. (2019). Oregano essential oil vapour prevents Plasmopara viticola infection in grapevine (Vitis vinifera) and primes plant immunity mechanisms. PLoS ONE 14, 1–29. doi: 10.1371/journal.pone.0222854
Riethmüller, A., Voglmayr, H., Göker, M., Weiß, M., and Oberwinkler, F. (2002). Phylogenetic relationships of the downy mildews (Peronosporales) and related groups based on nuclear large subunit ribosomal DNA sequences. Mycologia 94, 834–849. doi: 10.2307/3761698
Riethmüller, A., Weiß, M., and Oberwinkler, F. (1999). Phylogenetic studies of Saprolegniomycetidae and related groups based on nuclear large subunit ribosomal DNA sequences. Can. J. Bot. 77, 1790–1800. doi: 10.1139/cjb-77-12-1790
Romanazzi, G., Mancini, V., Feliziani, E., Bastianelli, M., Servili, A., Nardi, S., et al. (2014). Efficacia di prodotti alternativi nella difesa antiperonosporica della vite. ATTI Giornate Fitopatol. 2, 247–254.
Rossi, V., Caffi, T., and Giosuè, S. (2009). Modelling the dynamics of infections caused by sexual and asexual spores during Plasmopara viticola epidemics. J. Plant Pathol. 91, 615–627. doi: 10.4454/JPP.V91I3.553
Sapkota, S., Chen, L. L., Yang, S., Hyma, K. E., Cadle-Davidson, L., and Hwang, C. F. (2019). Construction of a high-density linkage map and QTL detection of downy mildew resistance in Vitis aestivalis-derived 'Norton.' Theor. Appl. Genet. 132, 137–147. doi: 10.1007/s00122-018-3203-6
Sargolzaei, M., Maddalena, G., Bitsadze, N., Maghradze, D., Bianco, P. A., Failla, O., et al. (2020). Rpv29, Rpv30 and Rpv31: Three Novel Genomic Loci Associated With Resistance to Plasmopara viticola in Vitis vinifera. Front. Plant Sci. 11, 1–16. doi: 10.3389/fpls.2020.562432
Savini, I., Bardin, M., Bertrand, C., Brun, L., Daire, X., Fabre, F., et al. (2018). Can Organic Agriculture Cope Without Copper for Disease Control? Paris: Synthesis of the collective Scientific Assessment Report, INRA (France).
Savory, E. A., Granke, L. L., Quesada-Ocampo, L. M., Varbanova, M., Hausbeck, M. K., and Day, B. (2011). The cucurbit downy mildew pathogen Pseudoperonospora cubensis. Mol. Plant Pathol. 12, 217–226. doi: 10.1111/j.1364-3703.2010.00670.x
Schneider, C., Onimus, C., Prado, E., Dumas, V., Wiedemann-Merdinoglu, S., Dorne, M. A., et al. (2019). INRA-ResDur: the French grapevine breeding programme for durable resistance to downy and powdery mildew. Acta Hortic. 1248, 207–213. doi: 10.17660/ActaHortic.2019.1248.30
Schröter, J. (1886). “Fam. Peronosporaceae.,” in Kryptogamen flora von Schlesien (Breslau: J. U. Kern), 483–484.
Schwander, F., Eibach, R., Fechter, I., Hausmann, L., Zyprian, E., and Töpfer, R. (2012). Rpv10: a new locus from the Asian Vitis gene pool for pyramiding downy mildew resistance loci in grapevine. Theor. Appl. Genet. 124, 163–176. doi: 10.1007/s00122-011-1695-4
Schweinitz, L. D. (1834). Synopsis fungorum in America Boreali media degentium. Trans. Am. Philos. Soc. 4, 141–316. doi: 10.2307/1004834
Sharma, R., Xia, X., Cano, L. M., Evangelisti, E., Kemen, E., Judelson, H., et al. (2015). Genome analyses of the sunflower pathogen Plasmopara halstedii provide insights into effector evolution in downy mildews and Phytophthora. BMC Genomics 16, 1–23. doi: 10.1186/s12864-015-1904-7
Silvar, C., Merino, F., and Díaz, J. (2008). Differential activation of defense-related genes in susceptible and resistant pepper cultivars infected with Phytophthora capsici. J. Plant Physiol. 165, 1120–1124. doi: 10.1016/j.jplph.2007.11.008
Sireesha, Y., and Velazhahan, R. (2016). Biological control of downy mildew of maize caused by Peronosclerospora sorghi under environmentally controlled conditions. J. Appl. Nat. Sci. 8, 279–283. doi: 10.31018/jans.v8i1.786
Skalický, V. (1983). The revision of species of the genus Peronospora on host plants of the family Rosaceae with respect to Central European species. Folia Geobot. Phytotaxon. 18, 71–101. doi: 10.1007/BF02855638
Slaughter, A. R., Md Hamiduzzaman, M., Gindro, K., Neuhaus, J.-M., and Mauch-Mani, B. (2008). Beta-aminobutyric acid-induced resistance in grapevine against downy mildew: involvement of pterostilbene. Eur. J. Plant Pathol. 122, 185–195. doi: 10.1007/s10658-008-9285-2
Slusarenko, A., Fraser, R. S. S., and Van Loon, L. C. (2000). Mechanisms of Resistance to Plant Diseases. Springer Science and Business Media B.V. doi: 10.1016/0885-5765(87)90073-7
Speiser, B., Berner, A., Häseli, A., and Tamm, L. (2000). Control of downy mildew of grapevine with potassium phosphonate: effectivity and phosphonate residues in wine. Biol. Agric. Hortic. 17, 305–312. doi: 10.1080/01448765.2000.9754851
Spencer-Phillips, P. T. N., and Jeger, M. (2016). Advances in Downy Mildew Research, Vol. 2. Dordrecht: Springer Science and Business Media B.V. doi: 10.1007/978-1-4020-2658-4
Spring, O., and Haas, K. (2002). The fatty acid composition of Plasmopara halstedii and its taxonomic significance. Eur. J. Plant Pathol. 108, 263–267. doi: 10.1023/A:1015173900047
Spring, O., and Thines, M. (2004). On the necessity of new characters for classification and systematics of biotrophic Peronosporomycetes. Planta 219, 910–914. doi: 10.1007/s00425-004-1341-3
Spring, O., and Zipper, R. (2006). Evidence for asexual genetic recombination in sunflower downy mildew, Plasmopara halstedii. Mycol. Res. 110, 657–663. doi: 10.1016/j.mycres.2006.03.009
Šrobárová, A., and Kakalíková, U. (2007). Fungal disease of grapevines. Eur. J. Plant Sci. Biotechnol. 1, 84–90.
Stassen, J. H., and Van Den Ackerveken, G. (2011). How do oomycete effectors interfere with plant life? Curr. Opin. Plant Biol. 14, 407–414. doi: 10.1016/j.pbi.2011.05.002
Steimetz, E., Trouvelot, S., Gindro, K., Bordier, A., Poinssot, B., Adrian, M., et al. (2012). Influence of leaf age on induced resistance in grapevine against Plasmopara viticola. Physiol. Mol. Plant Pathol. 79, 89–96. doi: 10.1016/j.pmpp.2012.05.004
Stummer, B. E., Francis, I. L., Zanker, T., Lattey, K. A., and Scott, E. S. (2005). Effects of powdery mildew on the sensory properties and composition of Chardonnay juice and wine when grape sugar ripeness is standardised. Aust. J. Grape Wine Res. 11, 66–76. doi: 10.1111/j.1755-0238.2005.tb00280.x
Sullivan, M., MacKinnon, D., Price, T., Wright, R. J., and Jackson, T. J. (2010). Corn commodity-based survey reference. A guideline paper from Cooperative Agricultural Pest Survey (CAPS). Plant Protection and Quarantine Center for Plant Health Science and Technology, North Carolina State University. Raleigh, NC, United States.
Takemiya, A., Sugiyama, N., Fujimoto, H., Tsutsumi, T., Yamauchi, S., Hiyama, A., et al. (2013). Phosphorylation of BLUS1 kinase by phototropins is a primary step in stomatal opening. Nat. Commun. 4, 1–8. doi: 10.1038/ncomms3094
Talontsi, F. M., Islam, M. T., Facey, P., Douanla-Meli, C., Von Tiedemann, A., and Laatsch, H. (2012). Depsidones and other constituents from Phomopsis sp. CAFT69 and its host plant Endodesmia calophylloides with potent inhibitory effect on motility of zoospores of grapevine pathogen Plasmopara viticola. Phytochem. Lett. 5, 657–664. doi: 10.1016/j.phytol.2012.06.017
Thines, M., and Choi, Y. J. (2016). Evolution, diversity, and taxonomy of the Peronosporaceae, with focus on the genus Peronospora. Phytopathology 106, 6–18. doi: 10.1094/PHYTO-05-15-0127-RVW
Thines, M., Göker, M., Oberwinkler, F., and Spring, O. (2007). A revision of Plasmopara penniseti, with implications for the host range of the downy mildews with pyriform haustoria. Mycol. Res. 111, 1377–1385. doi: 10.1016/j.mycres.2007.09.006
Thines, M., Göker, M., Spring, O., and Oberwinkler, F. (2006). A revision of Bremia graminicola. Mycol. Res. 110, 646–656. doi: 10.1016/j.mycres.2006.04.001
Thuerig, B., Binder, A., Boller, T., Guyer, U., Jimenez, S., Rentsch, C., et al. (2006). An aqueous extract of the dry mycelium of Penicillium chrysogenum induces resistance in several crops under controlled and field conditions. Eur. J. Plant Pathol. 114, 185–197. doi: 10.1007/s10658-005-4512-6
Thuerig, B., James, E., Schärer, H. J., Langat, M. K., Mulholland, D. A., Treutwein, J., et al. (2018a). Reducing copper use in the environment: the use of larixol and larixyl acetate to treat downy mildew caused by Plasmopara viticola in viticulture. Pest Manag. Sci. 74, 477–488. doi: 10.1002/ps.4733
Thuerig, B., Ramseyer, J., Hamburger, M., Ludwig, M., Oberhänsli, T., Potterat, O., et al. (2018b). Efficacy of a Magnolia officinalis bark extract against grapevine downy mildew and apple scab under controlled and field conditions. Crop Prot. 114, 97–105. doi: 10.1016/j.cropro.2018.08.011
Thuerig, B., Ramseyer, J., Hamburger, M., Oberhänsli, T., Potterat, O., Schärer, H. J., et al. (2016). Efficacy of a Juncus effusus extract on grapevine and apple plants against Plasmopara viticola and Venturia inaequalis and identification of the major active constituent. Pest Manag. Sci. 72, 1718–1726. doi: 10.1002/ps.4199
Thuerig, B., Schärer, H., Ludwig, M., James, E. E., Langat, M. K., Mulholland, D. A., et al. (2018). Development of a botanical plant protection product from Larix by-products to protect grapevine from Plasmopara viticola. Pest Manag. Sci. 74, 477–488.
Toffolatti, S. L., De Lorenzis, G., Brilli, M., Moser, M., Shariati, V., Tavakol, E., et al. (2020). Novel aspects on the interaction between grapevine and Plasmopara viticola: dual-RNA-seq analysis highlights gene expression dynamics in the pathogen and the plant during the battle for infection. Genes 11, 261. doi: 10.3390/genes11030261
Toffolatti, S. L., Maffi, D., Serrati, L., and Vercesi, A. (2011a). Histological and ultrastructural studies on the curative effects of mandipropamid on Plasmopara viticola. J. Phytopathol. 159, 201–207. doi: 10.1111/j.1439-0434.2010.01748.x
Toffolatti, S. L., Prandato, M., Serrati, L., Sierotzki, H., Gisi, U., and Vercesi, A. (2011b). Evolution of Qol resistance in Plasmopara viticola oospores. Eur. J. Plant Pathol. 129, 331–338. doi: 10.1007/s10658-010-9677-y
Toffolatti, S. L., Russo, G., Campia, P., Bianco, P. A., Borsa, P., Coatti, M., et al. (2018). A time-course investigation of resistance to the carboxylic acid amide mandipropamid in field populations of Plasmopara viticola treated with anti-resistance strategies. Pest Manag. Sci. 74, 2822–2834. doi: 10.1002/ps.5072
Tromp, A., and De Klerk, C. A. (1988). Effect of copperoxychloride on the fermentation of must and on wine quality. S. Afr. J. Enol. Vitic. 9, 31–36. doi: 10.21548/9-1-2307
Tröster, V. (2016). Achilles' heel of grapevine downy mildew - the contractile vacuole as target for potential control strategies in viticulture [dissertation]. Faculty of Chemistry and Biosciences, Karlsruhe Institute of Technology, Karlsruhe, Germany. p. 1–91.
Trouvelot, S., Varnier, A. L., Allègre, M., Mercier, L., Baillieul, F., Arnould, C., et al. (2008). A β-1,3 glucan sulfate induces resistance in grapevine against Plasmopara viticola through priming of defense responses, including HR-like cell death. Mol. Plant Microbe Interact. 21, 232–243. doi: 10.1094/MPMI-21-2-0232
Underdown, R. S., Sivasithamparam, K., and Barbetti, M. J. (2008). Inhibition of the pre- and postinfection processes of Plasmopara viticola on Vitis vinifera leaves by one protectant and four systemic fungicides. Australas. Plant Pathol. 37, 335–343. doi: 10.1071/AP08031
Van Heerden, C. J., Burger, P., Vermeulen, A., and Prins, R. (2014). Detection of downy and powdery mildew resistance QTL in a 'Regent' × 'RedGlobe' population. Euphytica 200, 281–295. doi: 10.1007/s10681-014-1167-4
Van Loon, L. C., Bakker, P. A. H. M., and Pieterse, C. M. J. (1998). Systemic resistance induced by rhizosphere bacteria. Annu. Rev. Phytopathol. 36, 453–483. doi: 10.1146/annurev.phyto.36.1.453
Vecchione, A., Zulini, L., Pertot, I., and Musetti, R. (2007). Biological control of Plasmopara viticola: a multisite approach. Acta Hortic. 754, 361–366. doi: 10.17660/ActaHortic.2007.754.47
Venuti, S., Copetti, D., Foria, S., Falginella, L., Hoffmann, S., Bellin, D., et al. (2013). Historical introgression of the downy mildew resistance gene Rpv12 from the Asian species Vitis amurensis into grapevine varieties. PLoS ONE 8, e0061228. doi: 10.1371/journal.pone.0061228
Vladimíra, Zelená, and Veverka, K. (2007). Effect of surfactants and liquid fertilisers on transcuticular penetration of fungicides. Plant Prot. Sci. 43, 151–156. doi: 10.17221/2236-PPS
Voglmayr, H. (2003). Phylogenetic relationships of Peronospora and related genera based on nuclear ribosomal ITS sequences. Mycol. Res. 107, 1132–1142. doi: 10.1017/S0953756203008438
Voglmayr, H. (2008). Progress and challenges in systematics of downy mildews and white blister rusts: new insights from genes and morphology. Eur. J. Plant Pathol. 122, 3–18. doi: 10.1007/s10658-008-9341-y
Voglmayr, H., and Constantinescu, O. (2008). Revision and reclassification of three Plasmopara species based on morphological and molecular phylogenetic data. Mycol. Res. 112, 487–501. doi: 10.1016/j.mycres.2007.10.009
Voglmayr, H., Fatehi, J., and Constantinescu, O. (2006). Revision of Plasmopara (Chromista, Peronosporales) parasitic on Geraniaceae. Mycol. Res. 110, 633–645. doi: 10.1016/j.mycres.2006.03.005
Voglmayr, H., Riethmüller, A., Göker, M., Weiss, M., and Oberwinkler, F. (2004). Phylogenetic relationships of Plasmopara, Bremia and other genera of downy mildew pathogens with pyriform haustoria based on Bayesian analysis of partial LSU rDNA sequence data. Mycol. Res. 108, 1011–1024. doi: 10.1017/S0953756204000954
Wang, Q., Lu, Y., Xin, Y., Wei, L., Huang, S., and Xu, J. (2016). Genome editing of model oleaginous microalgae Nannochloropsis spp. by CRISPR/Cas9. Plant J. 88, 1071–1081. doi: 10.1111/tpj.13307
Wawra, S., Djamei, A., Albert, I., Nürnberger, T., Kahmann, R., and Van West, P. (2013). In vitro translocation experiments with RxLR-reporter fusion proteins of avr1b from Phytophthora sojae and AVR3a from Phytophthora infestans fail to demonstrate specific autonomous uptake in plant and animal cells. Mol. Plant Microbe Interact. 26, 528–536. doi: 10.1094/MPMI-08-12-0200-R
Wawra, S., Trusch, F., Matena, A., Apostolakis, K., Linne, U., Zhukov, I., et al. (2017). The RxLR motif of the host targeting effector AVR3a of Phytophthora infestans is cleaved before secretion. Plant Cell 29, 1184–1195. doi: 10.1105/tpc.16.00552
Weiste, C., Pedrotti, L., Selvanayagam, J., Muralidhara, P., Fröschel, C., Novák, O., et al. (2017). The Arabidopsis bZIP11 transcription factor links low-energy signalling to auxin-mediated control of primary root growth. PLoS Genet. 13, e1006607. doi: 10.1371/journal.pgen.1006607
Werner, S., Steiner, U., Becher, R., Kortekamp, A., Zyprian, E., and Deising, H. B. (1968). Chitin synthesis during in planta growth and asexual propagation of the cellulosic oomycete and obligate biotrophic grapevine pathogen Plasmopara viticola. FEMS Microbiol. Lett. 208, 169–173. doi: 10.1111/j.1574-6968.2002.tb11077.x
Wicks, T., and Hall, B. (1990). Efficacy of dimethomorph (CME 151) against downy mildew of grapevines. Plant Dis. 74, 114–116. doi: 10.1094/PD-74-0114
Wilson, G. W. (1907). Studies in North American Peronosporales - II. Phytophthoreae and Rhysotheceae. Bull. Torrey Bot. Club 34, 387–416. doi: 10.2307/2479202
Wingerter, C., Eisenmann, B., Weber, P., Dry, I., and Bogs, J. (2021). Grapevine Rpv3-, Rpv10- and Rpv12-mediated defense responses against Plasmopara viticola and the impact of their deployment on fungicide use in viticulture. BMC Plant Biol. 21, 1–17. doi: 10.1186/s12870-021-03228-7
Wong, F. P., Burr, H. N., and Wilcox, W. F. (2001). Heterothallism in Plasmopara viticola. Plant Pathol. 50, 427–432. doi: 10.1046/j.1365-3059.2001.00573.x
Wong, F. P., and Wilcox, W. F. (2001). Comparative physical modes of action of Azoxystrobin, Mancozeb, and Metalaxyl against Plasmopara viticola (Grapevine Downy Mildew). Plant Dis. 85, 649–656. doi: 10.1094/PDIS.2001.85.6.649
Wyenandt, C. A., Simon, J. E., Pyne, R. M., Homa, K., Mcgrath, M. T., Zhang, S., et al. (2015). Basil Downy Mildew (Peronospora belbahrii): discoveries and challenges relative to its control. Phytopathology 105, 885–894. doi: 10.1094/PHYTO-02-15-0032-FI
Xiang, G., Yin, X., Niu, W., Chen, T., Liu, R., Shang, B., et al. (2021). Characterization of CRN-like genes from Plasmopara viticola: searching for the most virulent ones. Front. Microbiol. 12, 632047. doi: 10.3389/fmicb.2021.632047
Xiang, J., Li, X., Wu, J., Yin, L., Zhang, Y., and Lu, J. (2016). Studying the Mechanism of Plasmopara viticola RxLR Effectors on Suppressing Plant Immunity. Front. Microbiol. 7, 709. doi: 10.3389/fmicb.2016.00709
Yin, L., An, Y., Qu, J., Li, X., Zhang, Y., Dry, I., et al. (2017). Genome sequence of Plasmopara viticola and insight into the pathogenic mechanism. Sci. Rep. 7, 1–12. doi: 10.1038/srep46553
Yin, L., Li, X., Xiang, J., Qu, J., Zhang, Y., Dry, I. B., et al. (2015). Characterization of the secretome of Plasmopara viticola by de novo transcriptome analysis. Physiol. Mol. Plant Pathol. 91, 1–10. doi: 10.1016/j.pmpp.2015.05.002
Yoshioka, K., Kamo, S., Hosaka, K., Sato, R., Miikeda, Y., Manabe, Y., et al. (2019). Unified approach toward syntheses of juglomycins and their derivatives. ACS Omega 4, 11737–11748. doi: 10.1021/acsomega.9b01376
Zang, C., Lin, Q., Xie, J., Lin, Y., Zhao, K., and Liang, C. (2020). The biological control of the grapevine downy mildew disease using Ochrobactrum sp. Plant Prot. Sci. 56, 52–61. doi: 10.17221/87/2019-PPS
Zarraonaindia, C., Owens, I. M., Weisenhorn, S. M., West, P., Hampton-Marcell, K., Lax, J., et al. (2015). The soil microbiome influences grapevine-associated microbiota. MBio 6, 1–14. doi: 10.1128/mBio.02527-14
Zeng, Q., Xie, J., Li, Y., Gao, T., Zhang, X., and Wang, Q. (2021). Comprehensive Genomic Analysis of the endophytic bacillus altitudinis strain GLB197, a potential biocontrol agent of grape downy mildew. Front. Genet. 12, 729603. doi: 10.3389/fgene.2021.729603
Zhang, X., Zhou, Y., Li, Y., Fu, X., and Wang, Q. (2017). Screening and characterization of endophytic Bacillus for biocontrol of grapevine downy mildew. Crop Prot. 96, 173–179. doi: 10.1016/j.cropro.2017.02.018
Keywords: Plasmopara viticola, grapevine, downy mildew, disease management, Vitis vinifera
Citation: Koledenkova K, Esmaeel Q, Jacquard C, Nowak J, Clément C and Ait Barka E (2022) Plasmopara viticola the Causal Agent of Downy Mildew of Grapevine: From Its Taxonomy to Disease Management. Front. Microbiol. 13:889472. doi: 10.3389/fmicb.2022.889472
Received: 04 March 2022; Accepted: 19 April 2022;
Published: 11 May 2022.
Edited by:
Sabrina Sarrocco, University of Pisa, ItalyReviewed by:
Yi Xu, Nanjing Agricultural University, ChinaSugitha Thankappan, Karunya Institute of Technology and Sciences, India
Ashutosh Kumar Rai, Imam Abdulrahman Bin Faisal University, Saudi Arabia
Copyright © 2022 Koledenkova, Esmaeel, Jacquard, Nowak, Clément and Ait Barka. This is an open-access article distributed under the terms of the Creative Commons Attribution License (CC BY). The use, distribution or reproduction in other forums is permitted, provided the original author(s) and the copyright owner(s) are credited and that the original publication in this journal is cited, in accordance with accepted academic practice. No use, distribution or reproduction is permitted which does not comply with these terms.
*Correspondence: Qassim Esmaeel, qassim.esmaeel@univ-reims.fr; Essaid Ait Barka, ea.barka@univ-reims.fr