Effects of dietary plant and animal protein sources and replacement levels on growth and feed performance and nutritional status of market-sized turbot (Scophthalmus maximus) in RAS
- 1Alfred Wegener Institute for Polar and Marine Research, Life Sciences, Shelf Sea System Ecology, Bremerhaven, Germany
- 2Alfred Wegener Institute for Polar and Marine Research, Life Sciences, Integrative Ecophysiology, Bremerhaven, Germany
- 3Norwegian Institute of Bioeconomy Research, Department of Biomarine Resource Valorisation, Bodø, Norway
- 4SPAROS Lda., Olhã, Portugal
- 5Faculty 1 Technology, University of Applied Sciences Bremerhaven, Bremerhaven, Germany
One part of aquaculture sustainability is reducing the environmental footprint of aquaculture feeds. For European aquaculture, this means finding feed ingredients that are produced within the economic community, and that are not in conflict with human consumption. This is especially challenging when formulating diets for carnivorous fish such as turbot with low tolerance to fishmeal replacement that are both nutritious and economically and environmentally sustainable. Therefore, we investigated the effects of two novel and innovative feed formulation concepts on growth and feed performance and the nutritional status of market-sized turbot in a recirculating aquaculture system. In a 16-week feeding trial, 440 turbot (300 ± 9 g) were fed twice a day with a control diet (CTRL), based on a commercial formulation, and four experimental diets. The experimental diets were designed to investigate the effects of two formulations concepts based on sustainable terrestrial plant proteins (NoPAP) or processed animal proteins (PAP) and of 30% and 60% fishmeal replacement with emerging feed ingredients (fisheries by-products, insect meal and fermentation biomass). Turbot from the CTRL group had a similar growth and feed performance than fish fed the NoPAP30 formulation, with a significant decline of performance in the fish fed both PAP formulations and the NoPAP60. Comparing the two formulation concepts with each other the voluntary feed intake and protein efficiency ratio on tank basis as well as the individual weight gain and relative growth rate was significantly higher in the fish from the NoPAP groups than PAP groups. Furthermore, the apparent digestibility of nutrients and minerals was significantly reduced in the fish fed with the diets with 30% and 60% fishmeal replacement level compared to the fish from the CTRL group. In conclusion, the performance of the fish fed the NoPAP30 formulation concept highlights the potential of the used combination of sustainable ingredients, such as fisheries by-products, insect meal, microbial biomass and plant protein for turbot. Furthermore, this study shows that turbot has a higher tolerance to the incorporation of plant and insect protein than of processed animal protein.
1 Introduction
Anthropogenic activities, such as exploiting natural resources, building infrastructures and producing food have altered and shaped our planet and its ecosystems leading us into the new Anthropocene epoch (Lewis and Maslin, 2015). Within the Anthropocene, aquaculture may pose a risk as it can have a range of environmental impacts and its reliance on terrestrial agricultural products, fished marine protein and by competing with human food consumption for fish feeds (Troell et al., 2014; Keys et al., 2019). To conserve and sustainably use the valuable ecosystems and resources, the reduction of the environmental footprint of aquaculture feeds has become a high priority for the scientific community, producers and consumers (Glencross et al., 2020; Naylor et al., 2021). Furthermore, global crises have highlighted the importance of locally sourced raw materials and the reduction of the dependence on imports in many production sectors (Folke et al., 2021; Sarà et al., 2022).
For European aquaculture, this means to utilize sustainable feed ingredients that are produced within the economic community, such as plant protein from lupines and peas, and that are used for human consumption, such as insect meal and by-products from food processing of marine and terrestrial animals. Research efforts focused on the identification of major nutritional requirements for important farmed fish such as trout, salmon, sea bass or seabream (FAO, 2020; Naylor et al., 2021), resulting in diet formulations with reduced fish content that improve growth and feed performance (Olsen and Hasan, 2012; Hua et al., 2019). However, in many carnivorous farmed fish, a total replacement of fish products in the diets is still not feasible. The needed fish protein can be sourced from side-streams of the aquaculture production sector (Vazquez et al., 2020; Malcorps et al., 2021), which are a good alternative for sustainable aquafeeds (Forster et al., 2005; Whiteman and Gatlin, 2005; Bendiksen et al., 2011; Hua et al., 2019). Processed raw materials such as fish protein hydrolysates are more energy efficient than fishmeal from by-products and were shown to improve growth and feed performance in farmed fish (Siddik et al., 2020). Other alternative feed ingredient sources are processed animal proteins from poultry and pork by-products of the human food industry. The availability in large amounts in the EU and elsewhere as a byproduct from food production and its nutritional value qualifies processed animal proteins (PAPs) as a sustainable feed ingredient for fish (Tacon et al., 2011; Lu et al., 2015; Wang et al., 2015; Campos et al., 2017; Wu et al., 2018; Karapanagiotidis et al., 2019). Insect meal derived from the black soldier fly (Hermetia illucens) or mealworm (Tenebrio molitor) is also a promising feed ingredient for carnivorous fish such as Atlantic salmon (Salmo salar) (Li et al., 2020), rainbow trout (Oncorhynchus mykiss) (Stadtlander et al., 2017; Jozefiak et al., 2019; Rema et al., 2019). Insect larvae can valorize unused plant material, not suitable for human consumption, and transform it into valuable nutrients (Newton et al., 2005; Van Huis, 2013). Microbial biomass, which is produced as a by-product from food, beer and biogas production, can be a valuable ingredient in aquafeeds (Oliva-Teles and Goncalves, 2001; Aas et al., 2006; Bendiksen et al., 2011; Tacon et al., 2011; Olsen and Hasan, 2012; San Martin et al., 2020). Life-cycle assessment revealed that insect meal and poultry by-product meal are the most sustainable alternatives for partial fishmeal replacement (Maiolo et al., 2020).
The European fish consumers prefer high value carnivorous species such as salmon, trout, sea bass, and turbot (Petereit et al., 2022b). Our approach within the GAIN2020 project (EU H2020 grant no. 773330) was to develop alternative feed formulation concepts for a range of European aquaculture species such as salmon, trout (Maiolo et al., 2021), sea bream (Naya-Català et al., 2021), sea bass (Petereit et al., 2022a) and turbot (Hoerterer et al., 2022b) based on various proteins sourced through circular economy tapping by-products and side-streams from food production sectors.
The life cycle of a fish in aquaculture includes many different stages with different needs for nutrition. Focusing on the life stages that are fed with compound diets, the protein and energy requirements of carnivorous fish generally decrease with increasing size (Kousoulaki et al., 2015). Most feeding studies concentrate on the juvenile stage of fish, since they respond quicker to nutritional changes due to their fast growth, making studies more cost efficient since statistically relevant growth differences appear in a shorter time span (Charles Bai et al., 2022). However, it is also important to investigate the effects of alternative feed ingredients and formulation on fish in the grow-out phase. The grow-out phase is for many larger marketed fish such as salmon and turbot, the longest phase accounting of 1-2 years (FAO, 2022b; FAO, 2022a). Turbot is usually transferred at 4-6 month of age and with a body weight of approximately 100g into land-based flow-through or recirculation aquaculture systems (RAS) to start the grow-out phase (FAO, 2022b; EUMOFA, 2022). Feeding costs account for 50%-60% of the overall production costs in carnivorous fish species making feed quality an important issue for a successful farming operation in European aquaculture production (Davis and Hardy, 2022). Here, alternative feed ingredients, which are often cheaper, offer the potential of reducing feed costs. However, compared to traditional feed ingredients such as fishmeal and soybean products, they differ often in quality, nutritional composition, digestibility of nutrients and availability of minerals (Glencross, 2016; Hua et al., 2019). This may affect growth, nutrient utilization and whole body composition (Bonaldo et al., 2015; Aragao et al., 2020) of carnivorous fish and lead to an altered energy metabolism and energy allocation (Palma et al., 2020).
In this study, we used a variety of key performance indicators for growth, feed utilization and nutritional status to find an alternative formulation for marked-sized turbot to the currently used commercial formulation, which has a similar performance and is economically attractive. In a bi-directional approach, we investigated (1) the effects of two novel and innovative feed formulation concepts based on sustainable feed ingredients such as plant protein, krill meal, insect meal and microbial biomass without (NoPAP) and with the inclusion of processed animal protein (PAP) and (2) how much fishmeal could be replaced within these two formulation concepts (replacement levels 30% and 60%).
2 Material and methods
2.1 Experimental diets
Based on the nutrient requirements of marked sized turbot, five experimental diets were formulated to be isonitrogenous (crude protein. ~520 g/kg as fed), isolipidic (crude lipid: ~160 g/kg as fed) and isoenergetic (gross energy: 20 MJ/kg as fed) and manufactured as 6 mm floating pellets by extrusion at SPAROS LDA (Olhão, Portugal). The control diet (CTRL) was formulated to mimic a current standard commercial diet used for turbot with 40% fishmeal combined with 42% plant ingredients. One aspect of the feeding trial was to replace 30% and 60% of the fishmeal content in the novel formulation concepts. Additionally, the remaining fish-derived protein sources were fully replaced by fishmeal and fish protein hydrolysate (4% FHP) from fisheries by-products. The difference in fishmeal content was replaced by a combination of krill meal, insect meal and fermentation biomass (21% and 31% respectively). One formulation concept contained 30% plant protein concentrates (PPC) (NoPAP) and the second formulation concept was based on 12% PPCs and on 21% processed animal proteins (PAP). Furthermore, 50% of traditional fish oil in the control diet was replaced by salmon oil from by-products and algae oil. Moreover, in the novel formulation concepts the selenium and iodine from the vitamin and mineral premix was replaced by enriched macro and microalgae to increase the bioavailability of selenium and iodine for turbot. The formulation and chemical composition of the experimental diets is shown in Tables 1 and 2. Before and during the trials the feed was stored at 4°C to ensure continuous quality of the diets throughout the feeding experiment.
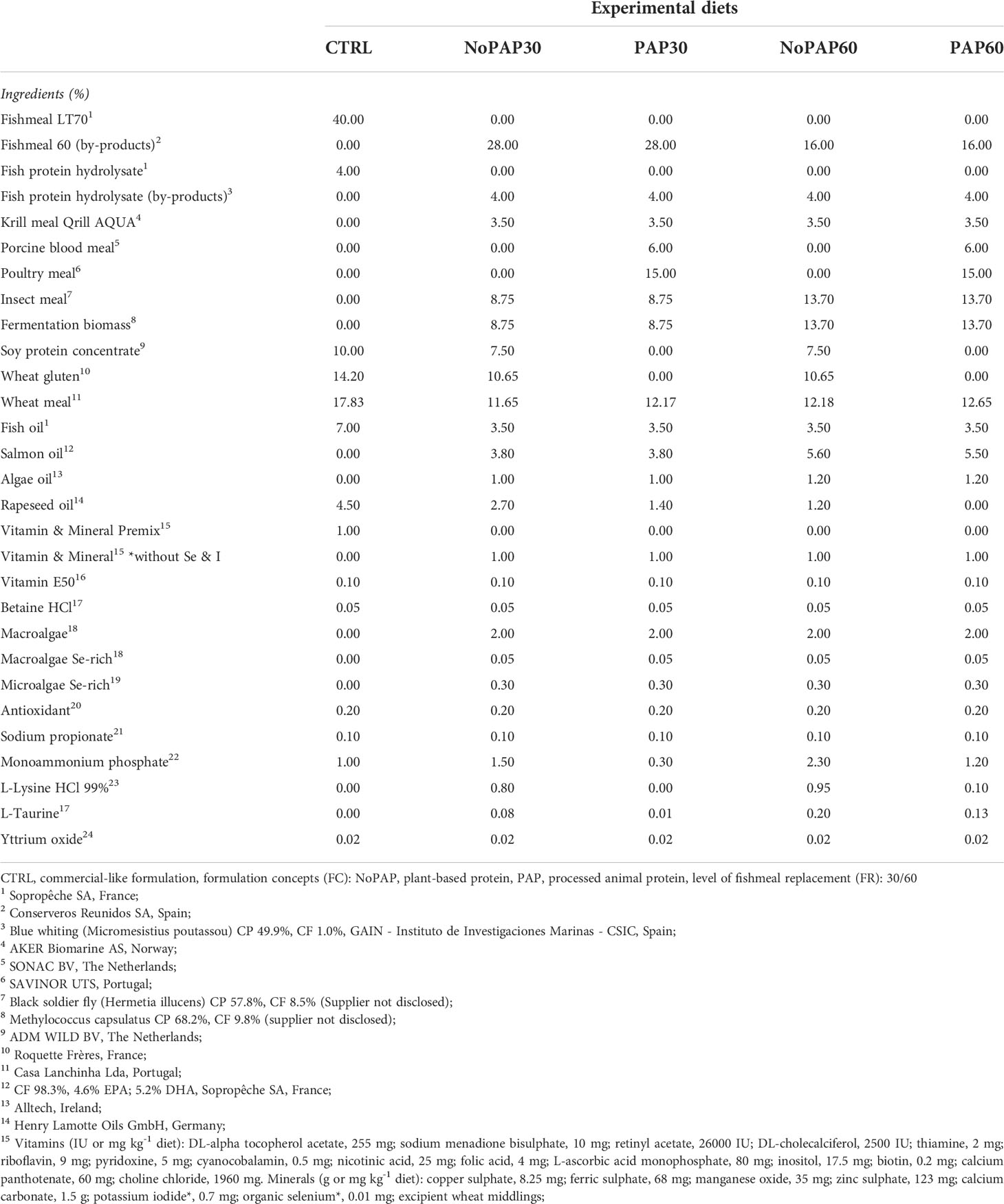
Table 1 Formulation of the five experimental diets (Manufacturer of feed ingredients) for market-sized turbot.
2.2 Experimental setup
Turbot (Scophthalmus maximus) were purchased from France Turbot (L’Épine, France), transferred in specified transport containers overland to the Centre for Aquaculture Research (ZAF) at the Alfred Wegener Institute Helmholtz Centre for Polar and Marine Research (AWI, Bremerhaven, Germany). The fish were acclimated to the recirculating aquaculture system (RAS) for two weeks prior to starting the 16 week (112 days) experimental trial. A total of 440 turbots with a mean weight (± SD) of 300.0 ± 8.5 g and total length of 25.3 ± 0.1 cm were randomly distributed into 20 tanks. The five experimental treatments were randomly distributed between the 20 tanks with four replicate tanks per treatment to counteract any possible tank effects. For more precise growth parameters 330 fish (300.0 ± 47.0 g and 25.3 ± 1.0 cm) were individually tagged with pit tags (7x1.35 mm, ISO 11784, Loligo Systems AS, Denmark). The RAS consisted of 36 tanks each containing 700 L with a bottom area of 1 m² each. The temperature (17.5 ± 0.1°C), pH (7.6 ± 0.1), conductivity (53.4 ± 1.8 mS/cm), and oxygen saturation (97.3 ± 5.3%) of the process water was monitored constantly (N = 112 days, SC 1000 Multiparameter Universal Controller, Hach Lange GmbH, Germany). The ammonium (0.12 ± 0.05 mg/L), nitrite (0.4 ± 0.3 mg/L), and nitrate (193.6 ± 34.5 mg/L) concentration was measured with an automated analyzer (QuAAtro39 AutoAnalyzer, SEAL Analytical, Germany) twice a week (N = 28).
The fish were fed in the morning (9 am) a weight adapted portion (1% of estimated tank biomass) and in the afternoon (2 pm) ad libitum. In the afternoon, 30 min after the feeding the remaining pellets were netted (mesh size 1 mm) from the tanks and counted.
2.3 Measurements and sampling
Fish were weighed to 0.2 g precision and measured in length to 0.5 cm precision and tags were read to track individual growth every four weeks. At the end of the 16-week trial, five fish from each of the 20 tanks were sampled for tissue sampling to determine the energy reserves and three fish per tank were sampled as whole fish for proximate and mineral analysis of the carcass. Fish were anesthetized with 500 mg/L tricaine methane sulphonate (MS-222, Sigma Aldrich, Germany) prior to exsanguination. After taking the weight (precision 0.01 g) and length (precision 0.5 cm) fish were killed and tissues (liver and filet without skin) were rapidly sampled on ice. The liver of 20 fish per treatment was weighed with 0.0001 g precision to determine the hepato-somatic index (HSI). Both tissues were shock frozen in liquid nitrogen and stored at -80°C until further analysis. For digestibility analysis, the feces were sampled by stripping anesthetized fish and pooled from all fish in one tank, centrifuged at 4°C and 3,000g for 5 min, and the pellets were frozen at -80°C until further analysis. To gain sufficient tissue weight the fish carcasses were pooled (from the three fish taken per tank), and cut into small pieces and stored at -20°C until further analysis.
For analysis of the marketable products of turbot three fish per tank (n = 12 per diet and N = 60 in total) were killed with a blow to the head and separation of the gill artery. The fish were weighed to 0.1 g precision and measured in length to 0.5 cm precision, visceral and filet removed and weighted to 0.1 g precision to determine the percentage of marketable gutted whole fish and filet yield.
2.4 Chemical analysis of diets, whole body and feces
The chemical analysis of the diets was conducted in duplicates (n=10), while carcass and fecal samples were pooled on tank level with four replicate tanks per experimental diet. The carcass samples were minced frozen using a commercial meat grinder homogeneously and refrozen The samples of the experimental diets homogenized in a knife grinder (5000 rpm, 30 s, Grindomix GM 200, Retsch, Germany).
The dry matter, ash, crude protein and crude lipid of the experimental diets and fish carcass were determined after AOAC (1980). Dry matter content of the diets and carcass was determined by drying the samples at 105°C for 24 h. Total ash content was determined by combustion of the samples in a muffle oven at 550°C for 6 h. The total nitrogen in the feed and carcass was determined following the automated Kjeldahl Method. Due to small sample volume in the feces samples, the total nitrogen in the freeze-dried feces was determined after the Dumas Method. For all samples, the measured total nitrogen was converted to equivalent crude protein (%) by the numerical factor of 6.25. Crude lipid was determined by acid hydrolysis. Gross energy was measured in an adiabatic bomb calorimeter (Model 6100; Parr Instrument, Germany).
For the analysis of the mineral content, 0.2 g of freeze-dried and homogenized samples of the experimental diets, carcass and feces were digested in 3 ml nitric acid HNO3 (65%, trace grade) in a microwave oven (CEM MARS5, Kamp-Lintfort, Germany) according to DIN EN 13805 (2014). After digestion, the samples were diluted with milli-q water to 50 mL. Calcium, potassium, magnesium, phosphorus, arsenic, copper, iron, manganese, selenium, yttrium and zinc concentrations were analyzed in an ICP-OES (iCAP7400, Fisher Scientific, Schwerte, Germany). As reference fish muscle (ERM – BB422, EU) was used.
2.5 Glycogen of liver and muscle tissue
Following the procedure described by Keppler and Decker (1988) glycogen content was determined photometrically after enzymatic hydrolysis of glycogen to glucose. Filet and liver samples of 5 individual fish per tank (20 fish per treatment) were grinded under liquid nitrogen and approx. 200 mg tissue was homogenized in 5x volume of ice-cold 0.6 M perchloric acid (PCA) (w:v). After one cycle of 20 s at 6000 rpm and 3°C using Precellys 24 (Bertin Technologies, France) samples were sonicated for 2 min at 0°C and 360 W (Branson Sonifier 450) and homogenates were immediately divided for the analysis of total and free glucose concentrations.
2.6 Data analysis
The growth parameters were based on initial body weight (BW, g), final BW and final body length (BL, cm) and calculated as follows.
The feed performance parameters, Voluntary feed intake (VFI) and feed conversion ratio (FCR) were based on the total feed intake (FI) in g of the offered amount of feed and the uneaten feed.
The apparent digestibility (ADC) of the dietary nutrients and minerals were based on the amount of the inert yttrium marker in the diet and feces and the respective nutrient or element in feces and diets.
The glycogen content was calculated based on the concentration of total glucose subtracted by the concentration of free glucose. The difference between Absorptions ΔA (A2 – A1), the total measurement volume of the assay Vassay total (mL), the coefficient of extinction at 339 nm (ϵ = 6.3 mL/µmol cm), the thickness of layer d = 1 cm for the cuvette, the sample volume Vsample (mL), the dilution factor (DF) and the concentration of tissue wet weight in crude extract ctissue (mg/mL).
The glucose concentration was converted to glycogen content using the molecular weight of the glucosyl moiety in glycogen with Mr = 162 g/mol.
Statistical analysis was conducted with Sigma Plot (12.5, Systat Software, Germany). One-way Analysis of Variance (ANOVA) was used to determine significant differences between the five treatments. Two-way ANOVA was used to determine significant differences and interaction between the novel formulation concepts (NoPAP vs. PAP; FC) and level of fishmeal replacement (30% vs. 60%; FR). Whenever there were statistically significant differences an All Pairwise Multiple Comparison Procedure was performed using the Holm-Sidak method (overall significance level P = 0.05) to find the difference within the treatments. Values are given as means ± standard deviations (SD).
3 Results
We present results relevant to the commercial culture on a tank basis (Table 3; n = 4 tanks per treatment) and growth performance on an individual basis (Table 4; average n = 66 fish per treatment, total N = 330 fish). Survival was 98 ± 4% (N = 20 tanks), with no significant differences between experimental groups.
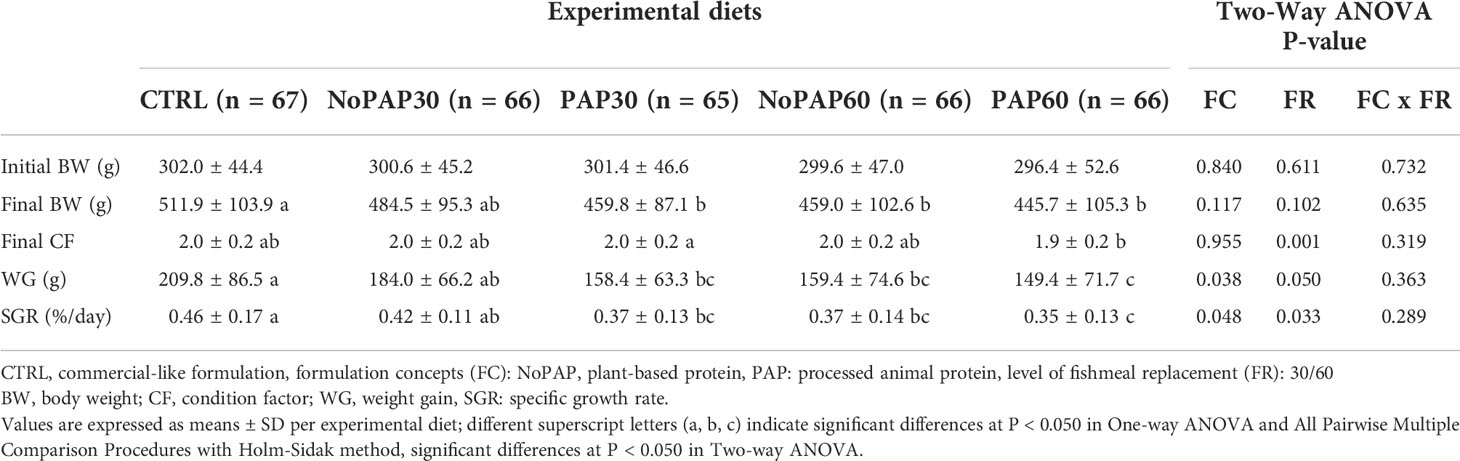
Table 4 Growth performance parameters of individually tagged turbot (N = 330) fed five experimental diets.
3.1 Growth and feed performance per tank
The fish from the control (CTRL) group had a significantly higher (One-way ANOVA; P = 0.043) final body weight (BW) than the fish from in the NoPAP60 and PAP60 groups, with no significant differences to the fish from the NoPAP30 and PAP30 groups (Table 3). The fish from the CTRL group had a significantly higher biomass gain (One-way ANOVA; P = 0.041) and specific growth rate (SGR) (One-way ANOVA; P = 0.010) than in the fish from the NoPAP60, PAP30 and PAP60 groups, with no significant difference to the fish from NoPAP30 group. The feed conversion ratio (FCR) was significantly (One-way ANOVA; P = 0.008) increased in the fish from the NoPAP60, PAP 30 and PAP60 groups compared to the fish from the CTRL groups), with no difference to the fish from the NoPAP30 group. The protein efficiency ratio (PER) was highest in the fish from the CTRL group and significantly decreased (One-way ANOVA, P = 0.001) in the fish from the NoPAP60, PAP30 and PAP60 groups, with no significant difference to the fish from the NoPAP30 group. The voluntary feed intake (VFI) with an average value of showed no significant differences between all experimental groups, with a slightly higher value in the fish from the PAP60 group. Fish fed with the PAP formulation concept (FC) had a significantly increased VFI (Two-way ANOVA ‘FC’; P = 0.028) and decreased PER compared (Two-way ANOVA ‘FC’; P = 0.017) to fish fed the NoPAP formulations, with no effect by replacement level (FR) and no interaction between formulation concept and replacement level (Two-way ANOVA ‘FC x FR’).
3.2 Individual growth performance
Due to the higher number of total replicates (N = 330), the analysis of the growth of the individually tagged fish revealed more comprehensive effects of the dietary formulations (Table 4). The fish from the CTRL group had a significantly (One-way ANOVA; P = 0.001) higher final BW than the fish from the PAP30, NoPAP60 and PAP60 groups, with no significant differences to the NoPAP30 group. The condition factor (CF) significantly increased (N = 330 fish; t-test; p< 0.001) from initially 1.8 ± 0.2 to finally 2.0 ± 0.2 in all groups. However, only the CF from the fish in the PAP30 group was significantly higher than in the fish from the PAP60 group (One-way ANOVA; P = 0.012). The weight gain (WG) and specific growth rate (SGR) in the fish from the CTRL group was significantly (One-way ANOVA; P< 0.001) higher than in the fish from the NoPAP60 PAP30 and PAP60 groups, with no significant differences to the NoPAP30 group. Furthermore, the fish from the NoPAP30 group had a significantly higher WG and RGR than the fish from the PAP60 group, with no significant differences to the PAP30 and NoPAP60 group.
Considering the replacement levels, the CF, WG and SGR of the fish from 30% groups were significantly higher than in the 60% groups (Two-way ANOVA ‘FR’, P = 0.001, P = 0.050, P = 0.033, respectively). Furthermore, the WG and SGR of the fish fed with the NoPAP formulation were both significantly higher compared to the fish from the PAP groups (Two-way ANOVA ‘FC’, P = 0.038, P = 0.048). However, no interaction was found between the formulation concept and replacement level (Two-way ANOVA ‘FC x FR’).
3.3 Proximate and mineral composition on wet weight basis of the carcass and dress-out loss and filet yield
The proximate composition on wet weight basis of the fish carcass did not significantly differ between the five treatments (One way ANOVA, see Table 5) with a mean of all tanks (N = 20) of 74.9 ± 1.7% moisture, 17.2 ± 0.7% crude protein, 3.7 ± 1.0% crude lipid, 4.7 ± 0.8% ash and 4.6 ± 0.6 MJ/kg gross energy. However, there were significant differences considering the interaction of formulation concepts with level of replacement (Two-way ANOVA ‘FC x FR’; P = 0.007) resulting in a significantly higher crude protein content in the fish fed with the NoPAP30 diet (P = 0.006) and in fish from the PAP60 group (P = 0.010) compared to the fish fed the NoPAP60 diet. Furthermore, the energy content of 4.9 ± 0.3 MJ/kg (n = 8) in the fish from the 30% replacement groups was significantly higher than 4.1 ± 0.6 MJ/kg (n = 8) in of the 60% groups (Two-way ANOVA ‘FR’; P = 0.009). The mineral composition of the fish carcasses did not significantly differ between all treatments (Table 5). The mean carcass contents of all tanks (N = 20) of 13.7 ± 3.4 g/kg calcium, 0.7 ± 0.1 mg/kg copper, 9.6 ± 8.1 mg/kg iron, 2.8 ± 0.3 g/kg potassium, 18.1 ± 4.0 mg/kg manganese, 1.9 ± 0.2 g/kg sodium, 7.9 ± 1.6 g/kg phosphorus, 0.5 ± 0.1 mg/kg selenium, 16.4 ± 2.2 mg/kg zinc.
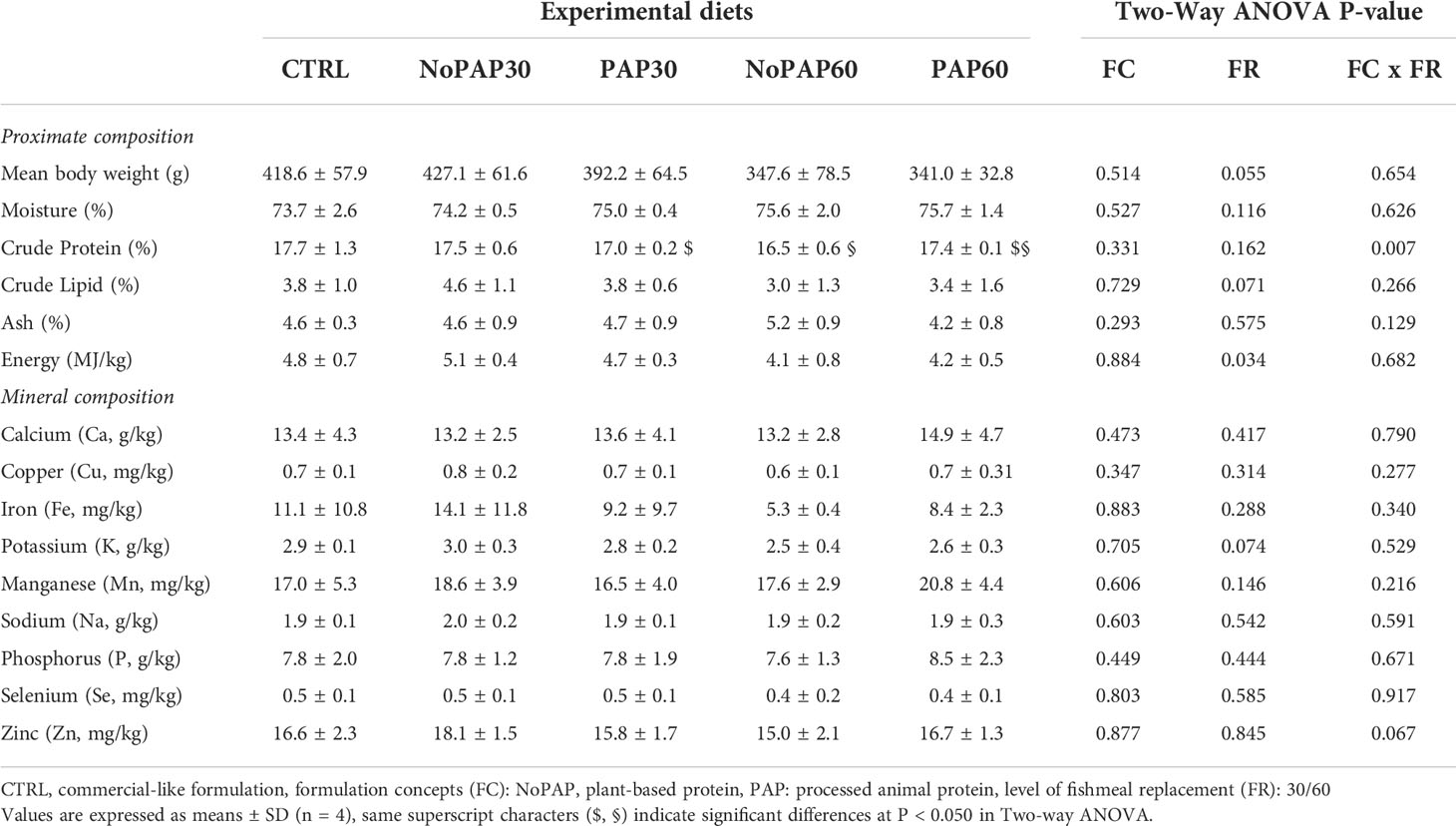
Table 5 Chemical composition of the fish carcass on wet weight basis of market-sized turbot fed with fed five experimental diets.
The fish from all experimental groups (N = 60) with a mean total body weight of 534.1 ± 80.8 g resulted in 94.1 ± 0.8% marketable whole fish gutted and a filet yield of 41.5 ± 4.2% with no significant differences between all diets.
3.4 Apparent digestibility of dry matter, crude protein and minerals
Due to a small sample volume of the feces collected from two tanks of the PAP30 group, the mineral analysis including the yttrium levels failed. Hence, the apparent digestibility coefficients (ADCs) from the fish from the PAP30 group (n = 2) are not statistically sound.
The ADC of dry matter (Table 6) in the fish from the CTRL group was significantly (One-way ANOVA, P< 0.001) higher than in fish from the PAP30, NoPAP60 and PAP60 groups, with no significant difference to the fish from the NoPAP30 group. Furthermore the fish from the 30% replacement groups had a significantly higher ADC of dry matter than the fish in the 60% replacement groups (Two-way ANOVA ‘FR’, P = 0.006). The ADC of crude protein in the fish from the CTRL group was significantly decreased in the fish from the NoPAP60 and PAP60 groups, with no significant differences to the fish from NoPAP30 and PAP30 groups.
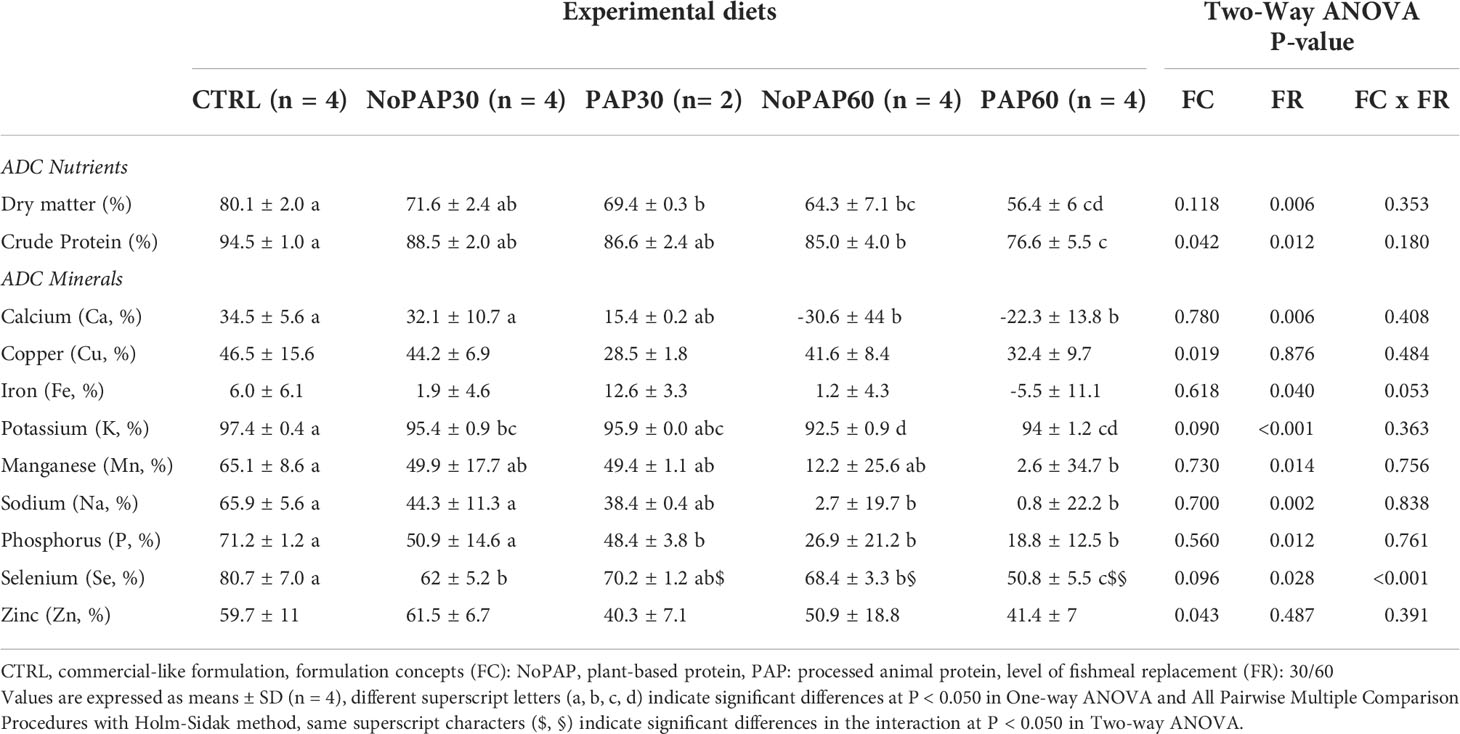
Table 6 Apparent digestibility coefficients (ADC) of nutrients and minerals of market-sized turbot fed with fed five experimental diets.
The ADCs of all evaluated minerals were significantly affected by the experimental diets except for copper, iron and zinc (Table 6). The ADC of calcium and sodium in fish from the control was significantly (One-way ANOVA, P = 0.003 and P< 0.001, respectively) higher than in the fish from the NoPAP60 and PAP60 groups with no significant differences to fish from the NoPAP30 and PAP30 groups. The fish from CTRL group had a significant (One-way ANOVA, both P< 0.001) higher potassium and selenium ADC than the fish from NoPAP30, NoPAP60 and PAP60 groups, with no significant difference to the fish from the PAP30 group. The fish from the CTRL group showed a significantly higher ADC of phosphorus (One-way ANOVA, P = 0.003) compared to the fish from the PAP30, NoPAP60 and PAP60 groups with no significant differences to fish from the NoPAP30 group. Furthermore the fish from the CTRL group showed a significantly (One-way ANOVA, P = 0.017) higher ADC of manganese than the PAP60 group with no significant differences to the fish from the other groups.
The formulation concept affected the ADC for potassium, being significantly higher in the fish from the NoPAP groups compared to the PAP groups (Two-way ANOVA ‘FC’, P = 0.026). The level of fishmeal replacement significantly affected (Two-way ANOVA ‘FR’) the ADCs of calcium (P = 0.006), potassium (P< 0.001), sodium (P< 0.001) and selenium (P = 0.006) being higher in the turbot from the 30% replacement groups compared to fish from the 60% groups. The ADC for selenium was significantly (Two-way ANOVA ‘FC x FR’) higher in the PAP30 compared to PAP60 (P< 0.001) and compared to the NoPAP30 group (P = 0.005). Fish from the NoPAP60 group also had a significantly higher selenium ADC than the fish from the PAP60 group (P< 0.001).
3.5 Energy reserve parameters
The energy reserve parameters such as hepato-somatic index and glycogen storage in liver and muscle were not significantly affected by the diets. The hepato-somatic index of the sampled fish was on average 1.5 ± 0.4 (N = 100 fish). The glycogen content in the liver ranged from 48.8 ± 26.5 mg/g (n = 20) in fish from the PAP30 group to 38.4 ± 23.2 mg/g (n = 20) in fish from the PAP60 group with a total mean of 43.0 ± 23.9 mg/g (n = 100). The glycogen in the muscle ranged from 3.3 mg/g in the fish from the NoPAP30 and PAP30 groups to 2.8 mg/g (n = 20) in fish from the NoPAP60 group with a total mean of 3.1 ± 1.0 mg/g (n = 100).
4 Discussion
In this study, we observed adverse effects on growth and feed performance as well as the nutrient utilization of marked-sized turbot fed with diets of different levels of processed animal proteins (PAP) and a high level of sustainable feed ingredients without the inclusion of PAPs (NoPAP) compared to the turbot from the control group (CTRL) fed with a commercial formulation. On an individual fish basis, due to a higher sample number (N = 330) these effects were more comprehensive and could be traced either to the formulation concept or to the fishmeal (FM) replacement level.
There are only a few studies, which investigate the effects of alternative feed ingredients on the growth and feed performance of larger sized turbot with an initial body weight (BW) of more than 100 g. In order to classify the observed growth performance, we first compare the growth performance of the fish from the CTRL group (initial BW: 303 g; weight gain (WG): 210 g; specific growth rate (SGR): 0.47%/day; mean water temperature: 17.5°C) with growth data and models for turbot in similar aquaculture settings. Compared to the modeled growth performance of strain B (WG: 215 g and SGR: 0.48%/day at 16.5°C water temperature) in Lugert et al. (2019) and to the data from Baer et al. (2011) the fish from the CTRL group had a normal growth performance. However, the growth was lower compared to the models from Arnason et al. (2009) (WG: 385 g and SGR: 0.74%/day at 17.5°C) and Lugert et al. (2019) for strain A (WG: 270 g and SGR: 0.57%/day at 16.5°C) and to the data from Weiß and Buck (2017) (initial BW: 201 g; SGR: 0.82%/day at 16.4°C). This comparably lower growth performance of the fish from the CTRL group might be explained by the origin of the turbot strain used in the present study. Turbot is known to exhibit counter gradient variation (Imsland et al., 2000) and strains from lower latitudes, such as France, show generally lower growth compared to populations from higher latitudes, such as Norway and Iceland (Imsland et al., 2001).
The feed performance of the fish from the CTRL group measured as voluntary feed intake (VFI: 0.61% ABW/day) and feed conversion ratio (FCR: 1.3) was slightly better than modeled for strain B at 16.5°C (0.56% ABW/day and 1.47, respectively) in Lugert et al. (2019). Arnason et al. (2009) calculated the optimum temperature at 16.1°C for the FCR for large turbot (499g) at a value of 0.77, which is similar to the water temperature in this study.
The growth and feed performance the turbot from the CTRL group exhibited, it can be considered as normal for a strain from France and the diet-dependent effects should be comparable to other studies using smaller turbot.
There were no diet-dependent effects on the proximate composition (N = 20) of the carcass with values within the range of turbot (Dietz et al., 2012; Bonaldo et al., 2015; Fuchs et al., 2015; Bian et al., 2017; Hoerterer et al., 2022b). However, this is expected since the proximate composition of growing fish is mainly determined by fish size, life cycle stage and energy intake (Shearer, 1994), which did not differ in this study. Similarly for the mineral composition of the carcass, where data for turbot is rare but similar to previous studies (van Bussel et al., 2014; Hoerterer et al., 2022b), and is similar to those of other species (Antony Jesu Prabhu et al., 2016). Differences in the mineral composition are only expected when there are strong deficiencies in the diet (Shearer, 1994) and are further influenced by the mineral concentrations in the rearing water (van Bussel et al., 2014; Antony Jesu Prabhu et al., 2016). Both factors are not present in this study, since the diets were formulated and manufactured to meet the species-specific demands for macro- and micronutrients and all fish were kept in the same rearing water.
The nutritional status (N = 100) indicated by the hepato somatic index (HSI) and glycogen content in liver and muscle did not show any diet-dependent effects. The HSI of the turbot in this study were on the lower range of compared to other studies (Dietz et al., 2012; Bonaldo et al., 2015; Nagel et al., 2017) it can be considered as normal. The in general lower HSI might be attributed to the French strain used in this study, as similar to growth turbot strains from France exhibit lower BW and lower HSI compared to strains from other countries such as Norway (Schlicht et al., 2019), Great Britain (Arfsten et al., 2010) and Iceland (Imsland et al., 2001). Positively correlated to the HSI is the hepatic glycogen as it is stored in the liver as an energy reserve and therefore influences the liver weight (Hemre et al., 2002). The glycogen levels in liver and muscle are also within the expected range (Liu et al., 2014; Guerreiro et al., 2015; Zeng et al., 2015; Miao et al., 2016).The nutritional status indicators show that at the end of the study, the fish could be considered as healthy and the energy allocation not negatively affected.
4.1 Effects of the formulation concepts and fish meal replacement level
Similar to salmonids (Hua and Bureau, 2012), this study suggest that the tolerance to FM replacement depends on the group of alternative ingredients. At lower levels of FM replacement, these effects cannot be detected, as shown in the preceding study with smaller turbot (initial BW: 20 g), there were no significant differences between the PAP and PLANT (analogue to NoPAP) formulation concepts (Hoerterer et al., 2022b), which might be contributed to the comparably low FM replacement level of 20%. In order to further explain the differences in protein digestibility and growth further analysis of the amino-acid profile of the diets and a more detailed evaluation of the variability of the nutritional value for the specific fish species could be helpful to optimize the formulation concepts (Glencross et al., 2020). The results from this study suggest that the inflection point of FM replacement level for turbot lies between 30% and 60% and might be higher for plant protein ingredients that for animal protein ingredients. This means that more fishmeal could be replaced with plant protein ingredients in the diets and maintain similar performance as the currently used practical diets. This could lead to an economic advantage since the calculated costs of the NoPAP formulation is lower than of the commercial diets.
4.1.1 Growth and feed performance
The growth performance of the market-sized turbot was better in the fish from the NoPAP groups as well as the 30% FM replacement groups, without any interactions between those factors. A similar pattern was observed for the protein efficiency ratio (PER). These performance indicators obtained in the present study showed that the market-sized turbot could better utilize the NoPAP than the PAP formulations, independent from the FM replacement level. Even though the fish from the PAP groups consumed more of the diets on a daily basis, they were not able to compensate the growth and feed utilization. Similar to this study, plant proteins allowed a higher level of FM replacement of 35% and more without negatively affecting growth rate (Burel et al., 2000a; Fournier et al., 2004; Bonaldo et al., 2015; Hermann et al., 2016; von Danwitz et al., 2016; Bian et al., 2017). Whereas, turbot growth reacts more sensitive to FM replacement by terrestrial animal derived proteins such as insect meal (20%, Kroeckel et al., 2012) and feather meal (30%, Cao et al., 2020). Looking at the possible interactions between the formulation concepts and the level of FM replacement, only the crude protein content of the carcass was affected. The fish from the PAP60 group had a significantly higher crude protein content in the carcass than in fish from the NoPAP60 group, reflecting the low crude protein content in the NoPAP60 diet (50.5%). This is in contrast to Shearer (1994) who suggested that fish size is the main factor influencing the crude protein of the carcass.
4.1.2 Apparent digestibility of nutrients
The effects on the growth and feed performance by the formulation concepts and by the level of fishmeal replacement might be contributed to the fact that the digestibility of the single ingredients used to replace the fish protein fraction differs greatly and these effects accumulate at higher replacement levels. The ADC of protein was higher in the turbot from the NoPAP groups than in the PAP groups. This reflects the results from previous studies reporting a higher digestibility of plant protein (70 - 96%; Burel et al., 2000b, 84 - 86%; Bonaldo et al., 2011) than the digestibility of insect meal (63%; Kroeckel et al., 2012) or of processed animal proteins (75 - 78%; Davies et al., 2009, 70-74%; Cao et al., 2020). Similar to growth and in line with previous studies the ADC of crude protein in turbot decreases with FM inclusion level (Regost et al., 1999; Bonaldo et al., 2011; Liu et al., 2014; Bai et al., 2019; Li et al., 2019). Furthermore, the fishmeal from by products and the fish protein hydrolysate might have a lower protein digestibility (47%; Davies et al., 2009) as well, but the effects only accumulate in the fish fed the diets with the higher replacement. The level of FM replacement also had an effect on the energy content of the carcass and ADCs of dry matter and of some minerals. The ADC of dry matter manly depends on composition of macronutrients present in the diets, and can depend on the carbohydrate levels in plant-based diets (REF). In order to get a more detailed picture into the causes of decreased digestibility of protein, amino acid composition and digestibility and possible anti-nutritional factors (Hua et al., 2019)present further analysis of the single ingredients and diets need to be done to evaluate the variability of the nutritional value for the specific fish species (Glencross et al., 2020).
4.1.3 Apparent digestibility of minerals
The digestibility of minerals strongly depends on the bioavailability in the ingredients (Lall and Kaushik, 2021). In this study, the mineral composition of the fish carcass was not affected by the formulations concepts nor by the level of FM replacement indicating no deficiencies. However, the ADCs of most minerals measured, except for copper and zinc, were correlated to the level of FM in the diets, being highest in the CTRL group and lowest in the 60% replacement groups. This indicates a lower bioavailability of the minerals in the alternative feed ingredients, due to different mineral composition leading to interaction between minerals in by-product fish meals, the presence of mineral binding compounds such as phytate in plant ingredients (Lall, 2022). Especially in the case of calcium and sodium, the low levels might indicate an increased uptake of calcium and sodium from seawater, due to low dietary levels (Lall, 2022). Copper and zinc availability was lower in the PAP groups than in the NoPAP groups indicating lower availability in animal derived feed ingredients. Besides the correlation to the FM replacement level, the low selenium digestibility in the PAP60 indicates a higher availability in the low fishmeal replacement levels within the PAP formulation and the 60% replacement levels compared to the NoPAP formulation. This might be contributed to the fact, that the dietary selenium level was lowest in the PAP60 group.
4.2 Performance of the sustainable formulations concepts in comparison to a commercial formulation
On a tank basis, fish from the CTRL and NoPAP30 groups (n = 4) had similar final BW, biomass gain, SGR, FCR and PER, whereas feeding the PAP and NoPAP60 formulations negatively affected these performance indicators in the fish. A similar pattern was observed on an individual basis, in final BW, WG and SGR, with fish from the PAP60 group having a significantly lower performance than the fish from the NoPAP30 group. The ADCs of dry matter and crude protein as well as the macro minerals calcium, potassium, sodium and phosphorus and of the trace minerals manganese and selenium follow the same pattern. Even though no diet-dependent effects on growth performance and VFI could be detected in the preceding study on smaller turbot fed with the same formulation concepts with 20% fishmeal replacement, the PAP formulation concept negatively affected feed utilization (FCR, PER, ADC) (Hoerterer et al., 2022b).
At final sampling, the condition factor (CF) of the individually tagged fish from the PAP60 (1.9) group was significantly lower compared to the fish from the CTRL group (2.0). The difference is very small and the CFs presented in the fish from all groups is considered as normal (Fuchs et al., 2015; von Danwitz et al., 2016; Nagel et al., 2017; Weiß and Buck, 2017; Wanka et al., 2019), whereas turbot strains from France appear to have a lower CF (Imsland et al., 2000; Arfsten et al., 2010). In smaller turbot the CF was reduced at FM replacement levels of 45% by fermented soybean and blood meal in turbot (Dan et al., 2021; Zheng et al., 2022) more than 55% with plant-based diets (Bonaldo et al., 2015) and insect meal-based diets (Kroeckel et al., 2012). Similar to previous studies with smaller turbot, the CF of larger turbot has a higher tolerance at high FM replacement levels with plant protein ingredients than with animal protein ingredients.
The present results suggest that the NoPAP30 formulation could be an adequate alternative for the practical diets currently used in commercial turbot aquaculture. The PAP60 formulation seems to be the least suitable alternative to the practical diet, having the lowest overall performance, also compared to the NoPAP30 formulation.
4.3 Marketable product and prediction of feed and production costs
For a fish farmer’s revenue and overall production costs the growth and feed performance, price and amount of marketable product and feed costs are variable and therefore offer the opportunity to lower costs or/and increase profit. Turbot is considered a high value species in Europe and, depending on the market and country, sold as live/fresh or frozen fish (unit price between 7.30 €/kg to 17.18 €/kg) starting from 0.5 kg to larger fish of up to 3 kg (EUMOFA, 2022). In this study, the different diets did not affect the amount of the marketable products gutted fish (94%) and filet (42%) and lying within the commercial range (Arfsten et al., 2010; Schlicht et al., 2019).
The present results on growth and feed performance indicate that the NoPAP30 formulation has the highest potential to be used in the grow-out phase of commercial turbot aquaculture. Compared to the commercial diet, the NoPAP30 formulation is cheaper, therefore offering the opportunity to increase profits (Tirano et al., 2021). The lower formulation costs of the PAP formulation and being the more sustainable choice considering environmental impacts in life cycle assessment (Maiolo et al., 2020) will not outweigh the lower growth performance in this study. However, the price and competitiveness of the today’s standard feed ingredients might change due to shortages, thus further increasing the economic benefits of the alternative ingredients. Furthermore, to make sustainable fish products more attractive, communication efforts should include and target the preferences for sustainable lifestyle and products of the different consumer groups, which are known to be influenced by age, education and location of stakeholders/consumers (Maesano et al., 2020; Hoerterer et al., 2022a).
5 Conclusion
Comparing the growth and feed performance among all experimental diets as well as the potential costs, we can conclude that the NoPAP30 formulation is an environmentally sustainable and economically viable alternative to the current commercial/standard formulation. Furthermore, the performance of the fish fed the PAP30 and NoPAP60 formulations was similar showing that turbot has the potential for further fishmeal reduction with the alternative feed ingredients used in the NoPAP formulation concept. The fish fed with the PAP60 formulation had overall the lowest performance leading to the conclusion that processed animal protein is not a suitable feed ingredient for market-sized turbot at high inclusion and when compared to other sustainable alternatives such as plant protein. Further experimental studies in combination with meta-analyses and/or nutritional model simulations could find the inflection point. We conclude that for plant-based ingredients investigated here this breaking point is at a higher FM replacement level than for processed terrestrial animal protein ingredients used in the PAP.
In addition to the performance of the fish, the sustainability of the feed ingredients and the economic benefits for the fish farmer, other factors influence the sustainable development of European aquaculture. Consumers are becoming increasingly interested in the production processes of their food (health and welfare issues concerning animal husbandry, feeding and slaughter), making the communication of the life cycle analysis of a product necessary, thus warranting more transdisciplinary aquaculture research.
Data availability statement
The raw data supporting the conclusions of this article will be made available by the authors, without undue reservation.
Ethics statement
The experiments were performed under the guidelines of the local authority ‘Food surveillance, animal welfare and veterinary service (LMTVet)’ of the state of Bremen with the permission to carry out animal experiments (500–427-103–1/2019–1-19).
Author contributions
CH is lead author, conceptualized and designed the study, conducted the experiment, lab and data analysis, wrote and reviewed the manuscript, and prepared it for submission. JP conceptualized and designed the study, conducted the experiment, reviewed the manuscript. GL provided input to the methodology and analysis, provided laboratory resources and supervision. JJ conceptualized the study, provided input to data analysis, results and discussion, reviewed the manuscript, responsible for funding acquisition. LC: conceptualization and design of the study, provided resources, and responsible for project administration and funding acquisition. BB conceptualized and designed the study, provided input to results and discussion, and reviewed the manuscript, responsible for project administration and funding acquisition. All authors contributed to the article and approved the submitted version.
Funding
The study was part of the GAIN2020 project funded by the European Union’s Horizon 2020 research and innovation programme under grant agreement No. 773330.
Acknowledgments
The authors would like to thank the technical staff from the marine aquaculture group, the integrated ecophysiology group, the apprentices and the marine geochemistry group at the AWI for their efforts and valuable assistance. Special thanks go to Jorge Dias and the SPAROS aquafeed production team for feed formulation and prototyping. Special thanks to the GAIN2020 partners from IIM-CSIC, SHP and WUR for contributing their efforts of producing the feed ingredients based on the eco-intensification and circular economy concept.
Conflict of interest
Author LC was employed by the company SPAROS Lda.
The remaining authors declare that the research was conducted in the absence of any commercial or financial relationships that could be construed as a potential conflict of interest.
Publisher’s note
All claims expressed in this article are solely those of the authors and do not necessarily represent those of their affiliated organizations, or those of the publisher, the editors and the reviewers. Any product that may be evaluated in this article, or claim that may be made by its manufacturer, is not guaranteed or endorsed by the publisher.
References
Aas T. S., Grisdale-Helland B., Terjesen B. F., Helland S. J. (2006). Improved growth and nutrient utilisation in Atlantic salmon (Salmo salar) fed diets containing A bacterial protein meal. Aquaculture 259, 365–376. doi: 10.1016/j.aquaculture.2006.05.032
Antony Jesu Prabhu P., Schrama J. W., Kaushik S. J. (2016). Mineral requirements of fish: a systematic review. Rev. Aquac. 8, 172–219. doi: 10.1111/raq.12090
AOAC (1980). “Animal feed,” in Official methods of analysis, 13 ed. Ed. Horwitz ,. W. (Washington: Association of Official Analytical Chemists).
Aragao C., Cabano M., Colen R., Fuentes J., Dias J. (2020). Alternative formulations for gilthead seabream diets: Towards a more sustainable production. Aquac. Nutr. 26, 444–455. doi: 10.1111/anu.13007
Arfsten M., Tetens J., Thaller G. (2010). The application of easily recorded body traits to the prediction of performance parameters in turbot. Zuchtungskunde 82, 371–386.
Arnason T., Bjornsson B., Steinarsson A., Oddgeirsson M. (2009). Effects of temperature and body weight on growth rate and feed conversion ratio in turbot (Scophthalmus maximus). Aquaculture 295, 218–225. doi: 10.1016/j.aquaculture.2009.07.004
Baer A., Schulz C., Traulsen I., Krieter J. (2011). Analysing the growth of turbot (Psetta maxima) in a commercial recirculation system with the use of three different growth models. Aquac. Int. 19, 497–511. doi: 10.1007/s10499-010-9365-0
Bai N., Gu M., Liu M., Jia Q., Pan S., Zhang Z. (2019). Corn gluten meal induces enteritis and decreases intestinal immunity and antioxidant capacity in turbot (Scophthalmus maximus) at high supplementation levels. PloS One 14, e0213867. doi: 10.1371/journal.pone.0213867
Bendiksen E. A., Johnsen C. A., Olsen H. J., Jobling M. (2011). Sustainable aquafeeds: Progress towards reduced reliance upon marine ingredients in diets for farmed Atlantic salmon (Salmo salar l.). Aquaculture 314, 132–139. doi: 10.1016/j.aquaculture.2011.01.040
Bian F., Zhou H., He G., Wang C., Peng H., Pu X., et al. (2017). Effects of replacing fishmeal with different cottonseed meals on growth, feed utilization, haematological indexes, intestinal and liver morphology of juvenile turbot (Scophthalmus maximus l.). Aquac. Nutr. 23, 1429–1439. doi: 10.1111/anu.12518
Bonaldo A., Di Marco P., Petochi T., Marino G., Parma L., Fontanillas R., et al. (2015). Feeding turbot juveniles Psetta maxima l. with increasing dietary plant protein levels affects growth performance and fish welfare. Aquac. Nutr. 21, 401–413. doi: 10.1111/anu.12170
Bonaldo A., Parma L., Mandrioli L., Sirri R., Fontanillas R., Badiani A., et al. (2011). Increasing dietary plant proteins affects growth performance and ammonia excretion but not digestibility and gut histology in turbot (Psetta maxima) juveniles. Aquaculture 318, 101–108. doi: 10.1016/j.aquaculture.2011.05.003
Burel C., Boujard T., Kaushik S. J., Boeuf G., van der Geyten S., Mol K. A., et al. (2000a). Potential of plant-protein sources as fish meal substitutes in diets for turbot (Psetta maxima): growth, nutrient utilisation and thyroid status. Aquaculture 188, 363–382. doi: 10.1016/S0044-8486(00)00342-2
Burel C., Boujard T., Tulli F., Kaushik S. J. (2000b). Digestibility of extruded peas, extruded lupin, and rapeseed meal in rainbow trout (Oncorhynchus mykiss) and turbot (Psetta maxima). Aquaculture 188, 285–298. doi: 10.1016/S0044-8486(00)00337-9
Campos I., Matos E., Marques A., Valente L. M. P. (2017). Hydrolyzed feather meal as a partial fishmeal replacement in diets for European seabass (Dicentrarchus labrax) juveniles. Aquaculture 476, 152–159. doi: 10.1016/j.aquaculture.2017.04.024
Cao S. H., Li P. Y., Huang B. S., Song Z. D., Hao T. T., Wang C. Q., et al. (2020). Assessing feasibility of replacement of fishmeal with enzyme-treated feather meal in the diet of juvenile turbot (Scophthalmus maximus l.). Aquac. Nutr. 26, 1340–1352. doi: 10.1111/anu.13090
Charles Bai S., Hardy R. W., Hamidoghli A. (2022). “Chapter 10 - diet analysis and evaluation,” in Fish nutrition (Fourth edition). Eds. Hardy ,. R. W., Kaushik ,. S. J. (Academic Press). doi: 10.1016/B978-0-12-819587-1.00010-0
Dan Z. J., Jiang D. L., Zheng J. C., Tang Z. Y., Gong Y., Liu Y. T., et al. (2021). Effects of dietary inorganic salts supplementation on growth performance, bone mineral deposition, intestinal morphology and immune response of turbot juveniles (Scophthalmus maximus l.) in fermented soybean meal-based diets. Aquac. Nutr. 27, 2541–2554. doi: 10.1111/anu.13383
Davies S. J., Gouveia A., Laporte J., Woodgate S. L., Nates S. (2009). Nutrient digestibility profile of premium (category III grade) animal protein by-products for temperate marine fish species (European sea bass, gilthead sea bream and turbot). Aquac. Res. 40, 1759–1769. doi: 10.1111/j.1365-2109.2009.02281.x
Davis D. A., Hardy R. W. (2022). “Chapter 14 - feeding and fish husbandry,” in Fish nutrition (Fourth edition). Eds. Hardy ,. R. W., Kaushik ,. S. J. (Academic Press). doi: 10.1016/B978-0-12-819587-1.00015-X
Dietz C., Kroeckel S., Schulz C., Susenbeth A. (2012). Energy requirement for maintenance and efficiency of energy utilization for growth in juvenile turbot (Psetta maxima, l.): The effect of strain and replacement of dietary fish meal by wheat gluten. Aquaculture 358, 98–107. doi: 10.1016/j.aquaculture.2012.06.028
DIN (2014). “Lebensmittel – bestimmung von elementspuren – druckaufschluss; deutsche fassung EN 13805:2014,” in Deutsches institut für normung e. V (Berlin: Deutsches Institut für Normung e. V) doi: 10.31030/2141105.
EUMOFA (2022). COVID-19 impacts on farmed fish: focus on turbot and caviar (Luxembourg: Publications Office of the European Union: EU). doi: 10.2771/672226
FAO (2020). “The state of world fisheries and aquaculture 2020,” in Sustainability in action(Rome, Italy: Food and Agriculture Organization of the United Nations). doi: 10.4060/ca9229en
FAO (2022a) Salmo salar [Online] (Rome: Fisheries and aquaculture division). Available at: https://www.fao.org/fishery/en/culturedspecies/salmo_salar/en (Accessed July 8th 2022).
FAO (2022b) Scophthalmus maximus [Online] (Rome: Fisheries and Aquaculture Division). Available at: https://www.fao.org/fishery/en/culturedspecies/psetta_maxima/en (Accessed July 8th 2022).
Folke C., Polasky S., Rockström J., Galaz V., Westley F., Lamont M., et al. (2021). Our future in the anthropocene biosphere. Ambio 50, 834–869. doi: 10.1007/s13280-021-01544-8
Forster I., Babbitt J. K., Smiley S. (2005). Comparison of the nutritional quality of fish meals made from by-products of the Alaska fishing industry in diets for pacific threadfin (Polydactylus sexfilis). J. World Aquac. Soc. 36, 530–537. doi: 10.1111/j.1749-7345.2005.tb00401.x
Fournier V., Huelvan C., Desbruyeres E. (2004). Incorporation of a mixture of plant feedstuffs as substitute for fish meal in diets of juvenile turbot (Psetta maxima). Aquaculture 236, 451–465. doi: 10.1016/j.aquaculture.2004.01.035
Fuchs V. I., Schmidt J., Slater M. J., Zentek J., Buck B. H., Steinhagen D. (2015). The effect of supplementation with polysaccharides, nucleotides, acidifiers and Bacillus strains in fish meal and soy bean based diets on growth performance in juvenile turbot (Scophthalmus maximus). Aquaculture 437, 243–251. doi: 10.1016/j.aquaculture.2014.12.007
Glencross B. (2016). “Understanding the nutritional and biological constraints of ingredients to optimize their application in aquaculture feeds,” in Aquafeed formulation. Ed. Nates ,. S. F. (San Diego: Academic Press). doi: 10.1016/b978-0-12-800873-7.00003-8
Glencross B. D., Baily J., Berntssen M. H. G., Hardy R., MacKenzie S., Tocher D. R. (2020). Risk assessment of the use of alternative animal and plant raw material resources in aquaculture feeds. Rev. Aquac. 12, 703–758. doi: 10.1111/raq.12347
Guerreiro I., Enes P., Merrifield D., Davies S., Oliva-Teles A. (2015). Effects of short-chain fructooligosaccharides on growth performance and hepatic intermediary metabolism in turbot (Scophthalmus maximus) reared at winter and summer temperatures. Aquac. Nutr. 21, 433–443. doi: 10.1111/anu.12175
Hemre G. I., Mommsen T. P., Krogdahl A. (2002). Carbohydrates in fish nutrition: effects on growth, glucose metabolism and hepatic enzymes. Aquac. Nutr. 8, 175–194. doi: 10.1046/j.1365-2095.2002.00200.x
Hermann B. T., Reusch T. B. H., Hanel R. (2016). Effects of dietary purified rapeseed protein concentrate on hepatic gene expression in juvenile turbot (Psetta maxima). Aquac. Nutr. 22, 170–180. doi: 10.1111/anu.12251
Hoerterer C., Petereit J., Krause G. (2022a). Informed choice: The role of knowledge in the willingness to consume aquaculture products of different groups in Germany. Aquaculture 556, 738319. doi: 10.1016/j.aquaculture.2022.738319
Hoerterer C., Petereit J., Lannig G., Johansen J., Pereira G. V., Conceição L. E. C., et al. (2022b). Sustainable fish feeds: potential of emerging protein sources in diets for juvenile turbot (Scophthalmus maximus) in RAS. Aquac. Int. 30, 1481–1504. doi: 10.1007/s10499-022-00859-x
Hua K., Bureau D. P. (2012). Exploring the possibility of quantifying the effects of plant protein ingredients in fish feeds using meta-analysis and nutritional model simulation-based approaches. Aquaculture 356, 384–301. doi: 10.1016/j.aquaculture.2012.05.003
Hua K., Cobcroft J. M., Cole A., Condon K., Jerry D. R., Mangott A., et al. (2019). The future of aquatic protein: Implications for protein sources in aquaculture diets. One Earth 1, 316–329. doi: 10.1016/j.oneear.2019.10.018
Imsland A. K., Foss A., Naevdal G., Cross T., Bonga S. W., Ham E. V., et al. (2000). Countergradient variation in growth and food conversion efficiency of juvenile turbot. J. Fish Biol. 57, 1213–1226. doi: 10.1006/jfbi.2000.1384
Imsland A. K., Foss A., Stefansson S. O. (2001). Variation in food intake, food conversion efficiency and growth of juvenile turbot from different geographic strains. J. Fish Biol. 59, 449–454. doi: 10.1006/jfbi.2001.1637
Jozefiak A., Nogales-Merida S., Mikolajczak Z., Rawski M., Kieronczyk B., Mazurkiewicz J. (2019). The utilization of full-fat insect meal in rainbow trout (OncorhynchusmMykiss) nutrition: The effects on growth performance, intestinal microbiota and gastrointestinal tract histomorphology. Ann. Anim. Sci. 19, 747–765. doi: 10.2478/aoas-2019-0020
Karapanagiotidis I. T., Psofakis P., Mente E., Malandrakis E., Golomazou E. (2019). Effect of fishmeal replacement by poultry by-product meal on growth performance, proximate composition, digestive enzyme activity, haematological parameters and gene expression of gilthead seabream (Sparus aurata). Aquac. Nutr. 25, 3–14. doi: 10.1111/anu.12824
Keppler D., Decker K. (1988). “1.2 glycogen,” in Methods of enzymatic analysis: Metabolites 1: Carbohydrates, 3 ed. Ed. Bergmeyer ,. H. U. (Weinheim, Basel: Verlag Chemie).
Keys P. W., Galaz V., Dyer M., Matthews N., Folke C., Nyström M., et al. (2019). Anthropocene risk. Nat. Sustain. 2, 667–673. doi: 10.1038/s41893-019-0327-x
Kousoulaki K., Saether B. S., Albrektsen S., Noble C. (2015). Review on European sea bass (Dicentrarchus labrax,Linnaeus 1758) nutrition and feed management: a practical guide for optimizing feed formulation and farming protocols. Aquac. Nutr. 21, 129–151. doi: 10.1111/anu.12233
Kroeckel S., Harjes A. G. E., Roth I., Katz H., Wuertz S., Susenbeth A., et al. (2012). When a turbot catches a fly: Evaluation of a pre-pupae meal of the black soldier fly (Hermetia illucens) as fish meal substitute — growth performance and chitin degradation in juvenile turbot (Psetta maxima). Aquaculture 364-365, 345–352. doi: 10.1016/j.aquaculture.2012.08.041
Lall S. P. (2022). “Chapter 6 - the minerals,” in Fish nutrition (Fourth edition). Eds. Hardy ,. R. W., Kaushik ,. S. J. (Academic Press). doi: 10.1016/B978-0-12-819587-1.00005-7
Lall S. P., Kaushik S. J. (2021). Nutrition and metabolism of minerals in fish. Anim. (Basel) 11, 2711. doi: 10.3390/ani11092711
Lewis S. L., Maslin M. A. (2015). Defining the anthropocene. Nature 519, 171–180. doi: 10.1038/nature14258
Li Y., Kortner T. M., Chikwati E. M., Belghit I., Lock E.-J., Krogdahl Å. (2020). Total replacement of fish meal with black soldier fly (Hermetia illucens) larvae meal does not compromise the gut health of Atlantic salmon (Salmo salar). Aquaculture 520, 734967. doi: 10.1016/j.aquaculture.2020.734967
Liu X. W., Mai K. S., Liufu Z. G., Ai Q. H. (2014). Effects of dietary protein and lipid levels on growth, nutrient utilization, and the whole-body composition of turbot, Scophthalmus maximus, Linnaeus 1758, at different growth stages. J. World Aquac. Soc. 45, 355–366. doi: 10.1111/jwas.12135
Li C. Q., Zhang B. L., Wang X., Pi X. O. G., Wang X., Zhou H. H., et al. (2019). Improved utilization of soybean meal through fermentation with commensal shewanella sp. MR-7 in turbot (Scophthalmus maximus l.). Microb. Cell Factories 18, 214. doi: 10.1186/s12934-019-1265-z
Lugert V., Hopkins K., Schulz C., Schlicht K., Krieter J. (2019). The course of growth, feed intake and feed efficiency of different turbot (Scophthalmus maximus) strains in recirculating aquaculture systems. Turkish J. Fish. Aquat. Sci. 19, 305–312. doi: 10.4194/1303-2712-v19_4_05
Lu F., Haga Y., Satoh S. (2015). Effects of replacing fish meal with rendered animal protein and plant protein sources on growth response, biological indices, and amino acid availability for rainbow trout Oncorhynchus mykiss. Fish. Sci. 81, 95–105. doi: 10.1007/s12562-014-0818-7
Maesano G., Di Vita G., Chinnici G., Pappalardo G., Amico M. (2020). The role of credence attributes in consumer choices of sustainable fish products: A review. Sustainability 12, 10008. doi: 10.3390/su122310008
Maiolo S., Forchino A. A., Faccenda F., Pastres R. (2021). From feed to fork – life cycle assessment on an Italian rainbow trout (Oncorhynchus mykiss) supply chain. J. Cleaner Product. 289, 125155. doi: 10.1016/j.jclepro.2020.125155
Maiolo S., Parisi G., Biondi N., Lunelli F., Tibaldi E., Pastres R. (2020). Fishmeal partial substitution within aquafeed formulations: life cycle assessment of four alternative protein sources. Int. J. Life Cycle Assess. 25, 1455–1471. doi: 10.1007/s11367-020-01759-z
Malcorps W., Newton R. W., Sprague M., Glencross B. D., Little D. C. (2021). Nutritional characterisation of European aquaculture processing by-products to facilitate strategic utilisation. Front. Sustain. Food Syst. 5. doi: 10.3389/fsufs.2021.720595
Miao S. Y., Nie Q., Miao H. J., Zhang W. B., Mai K. S. (2016). Effects of dietary carbohydrate-to-lipid ratio on the growth performance and feed utilization of juvenile turbot (Scophthalmus maximus). J. Ocean Univ. China 15, 660–666. doi: 10.1007/s11802-016-2934-8
Nagel F., Appel T., Rohde C., Kroeckel S., Schulz C. (2017). Blue mussel protein concentrate versus prime fish meal protein as a dietary attractant for turbot (Psetta maxima l.) given rapeseed proteinbased diets. J. Aquac. Res. Dev. s2, 012. doi: 10.4172/2155-9546.S2-012
Naya-Català F., do Vale Pereira G., Piazzon M. C., Fernandes A. M., Calduch-Giner J. A., Sitjà-Bobadilla A., et al. (2021). Cross-talk between intestinal microbiota and host gene expression in gilthead Sea bream (Sparus aurata) juveniles: Insights in fish feeds for increased circularity and resource utilization. Front. Physiol. 12. doi: 10.3389/fphys.2021.748265
Naylor R. L., Hardy R. W., Buschmann A. H., Bush S. R., Cao L., Klinger D. H., et al. (2021). A 20-year retrospective review of global aquaculture. Nature 591, 551–563. doi: 10.1038/s41586-021-03308-6
Newton G. L., Sheppard D. C., Watson D. W., Burtle G. J., Dove C. R., Tomberlin J. K., et al. (2005). The black soldier fly, hermetia illucens, as a manure management/resource recovery tool. symposium on the state of the science of animal manure and waste management. Semantic Scholar 1, 5–7.
Oliva-Teles A., Goncalves P. (2001). Partial replacement of fishmeal by brewers yeast (Saccaromyces cerevisae) in diets for sea bass (Dicentrarchus labrax) juveniles. Aquaculture 202, 269–278. doi: 10.1016/S0044-8486(01)00777-3
Olsen R. L., Hasan M. R. (2012). A limited supply of fishmeal: Impact on future increases in global aquaculture production. Trends Food Sci. Technol. 27, 120–128. doi: 10.1016/j.tifs.2012.06.003
Palma M., Trenkner L. H., Rito J., Tavares L. C., Silva E., Glencross B. D., et al. (2020). Limitations to starch utilization in barramundi (Lates calcarifer) as revealed by NMR-based metabolomics. Front. Physiol. 11. doi: 10.3389/fphys.2020.00205
Petereit J., Hoerterer C., Bischoff-Lang A. A., Conceição L. E. C., Pereira G., Johansen J., et al. (2022a). Adult European seabass (Dicentrarchus labrax) perform well on alternative circular-Economy-Driven feed formulations. Sustainability 14, 7279. doi: 10.3390/su14127279
Petereit J., Hoerterer C., Krause G. (2022b). Country-specific food culture and scientific knowledge transfer events – do they influence the purchasing behaviour of seafood products? Aquaculture 560, 738590. doi: 10.1016/j.aquaculture.2022.738590
Regost C., Arzel J., Kaushik S. J. (1999). Partial or total replacement of fish meal by corn gluten meal in diet for turbot (Psetta maxima). Aquaculture 180, 99–117. doi: 10.1016/S0044-8486(99)00026-5
Rema P., Saravanan S., Armenjon B., Motte C., Dias J. (2019). Graded incorporation of defatted yellow mealworm (Tenebrio molitor) in rainbow trout (Oncorhynchus mykiss) diet improves growth performance and nutrient retention. Animals 9, 187. doi: 10.3390/ani9040187
San Martin D., Orive M., Iñarra B., Castelo J., Estévez A., Nazzaro J., et al. (2020). Brewers’ spent yeast and grain protein hydrolysates as second-generation feedstuff for aquaculture feed. Waste Biomass Valorization 11, 5307–5320. doi: 10.1007/s12649-020-01145-8
Sarà G., Mangano M. C., Berlino M., Corbari L., Lucchese M., Milisenda G., et al. (2022). The synergistic impacts of anthropogenic stressors and COVID-19 on aquaculture: A current global perspective. Rev. Fish. Sci. Aquac. 30, 123–135. doi: 10.1080/23308249.2021.1876633
Schlicht K., Krattenmacher N., Lugert V., Schulz C., Thaller G., Tetens J. (2019). Estimation of genetic parameters for growth and carcass traits in turbot (Scophthalmus maximus). Arch. Anim. Breed. 62, 265–273. doi: 10.5194/aab-62-265-2019
Shearer K. D. (1994). Factors affecting the proximate composition of cultured fishes with emphasis on salmonids. Aquaculture 119, 63–88. doi: 10.1016/0044-8486(94)90444-8
Siddik M. A. B., Howieson J., Fotedar R., Partridge G. J. (2020). Enzymatic fish protein hydrolysates in finfish aquaculture: a review. Rev. Aquac. 13, 406–430. doi: 10.1111/raq.12481
Stadtlander T., Stamer A., Buser A., Wohlfahrt J., Leiber F., Sandrock C. (2017). Hermetia illucens meal as fish meal replacement for rainbow trout on farm. J. Insects as Food Feed 3, 165–175. doi: 10.3920/Jiff2016.0056
Tacon A. G. J., Hasan M. R., Metian M. (2011). “Demand and supply of feed ingredients for farmed fish and crustaceans: trends and prospects,” in FAO fisheries and aquaculture technical paper, I (Rome: Food and Agriculture Organization of the United Nations).
Tirano M., Kreiss C., Regueir Abelleirao L., Agostini M., Baarset H., Bruckner C., et al. (2021). “Report of the valorisation of secondary products in the aquaculture and biobased industries,” in Deliverable 6.9. GAIN – green aquaculture intensification in Europe (EU Horizon 2020 project grant n°), 773330.
Troell M., Naylor R. L., Metian M., Beveridge M., Tyedmers P. H., Folke C., et al. (2014). Does aquaculture add resilience to the global food system? Proc. Natl. Acad. Sci. 111, 13257–13263. doi: 10.1073/pnas.1404067111
van Bussel C. G. J., Schroeder J. P., Mahlmann L., Schulz C. (2014). Aquatic accumulation of dietary metals (Fe, zn, Cu, Co, Mn) in recirculating aquaculture systems (RAS) changes body composition but not performance and health of juvenile turbot (Psetta maxima). Aquac. Eng. 61, 35–42. doi: 10.1016/j.aquaeng.2014.05.003
Van Huis A. (2013). Potential of insects as food and feed in assuring food security. Annu. Rev. Entomol. 58, 563–583. doi: 10.1146/annurev-ento-120811-153704
Vazquez J. A., Duran A. I., Menduina A., Nogueira M. (2020). Biotechnological valorization of food marine wastes: Microbial productions on peptones obtained from aquaculture by-products. Biomolecules 10, 18. doi: 10.3390/biom10081184
von Danwitz A., van Bussel C. G. J., Klatt S. F., Schulz C. (2016). Dietary phytase supplementation in rapeseed protein based diets influences growth performance, digestibility and nutrient utilisation in turbot (Psetta maxima l.). Aquaculture 450, 405–411. doi: 10.1016/j.aquaculture.2015.07.026
Wang Y., Wang F., Ji W. X., Han H., Li P. (2015). Optimizing dietary protein sources for Japanese sea bass (Lateolabrax japonicus) with an emphasis on using poultry by-product meal to substitute fish meal. Aquac. Res. 46, 874–883. doi: 10.1111/are.12242
Wanka K. M., Schulz C., Kloas W., Wuertz S. (2019). Administration of host-derived probiotics does not affect utilization of soybean meal enriched diets in juvenile turbot (Scophthalmus maximus). J. Appl. Ichthyol. 35, 1004–1015. doi: 10.1111/jai.13929
Weiß M., Buck B. H. (2017). Partial replacement of fishmeal in diets for turbot (Scophthalmus maximus, linnaeus 1758) culture using blue mussel (Mytilus edulis, linneus 1758) meat. J. Appl. Ichthyol. 33, 354–360. doi: 10.1111/jai.13323
Whiteman K. W., Gatlin D. M. (2005). Evaluation of fisheries by-catch and by-product meals in diets for red drum Sciaenops ocellatus l. Aquac. Res. 36, 1572–1580. doi: 10.1111/j.1365-2109.2005.01380.x
Wu Y. B., Ren X., Chai X. J., Li P., Wang Y. (2018). Replacing fish meal with a blend of poultry by-product meal and feather meal in diets for giant croaker (Nibea japonica). Aquac. Nutr. 24, 1085–1091. doi: 10.1111/anu.12647
Zeng L., Lei J. L., Ai C. X., Hong W. S., Liu B. (2015). Protein-sparing effect of carbohydrate in diets for juvenile turbot Scophthalmus maximus reared at different salinities. Chin. J. Oceanol. Limnol. 33, 57–69. doi: 10.1007/s00343-015-4070-5
Keywords: insect meal, grow-out phase, by-products, digestibility, minerals, consumer, sustainable, circular economy (CE)
Citation: Hoerterer C, Petereit J, Lannig G, Johansen J, Conceição LEC and Buck BH (2022) Effects of dietary plant and animal protein sources and replacement levels on growth and feed performance and nutritional status of market-sized turbot (Scophthalmus maximus) in RAS. Front. Mar. Sci. 9:1023001. doi: 10.3389/fmars.2022.1023001
Received: 19 August 2022; Accepted: 26 October 2022;
Published: 14 November 2022.
Edited by:
Nathalie Rose Le François, Espace pour la vie, CanadaReviewed by:
Francisco Javier Moyano, University of Almeria, SpainMingzhu Pan, Ocean University of China, China
Jens Tetens, University of Göttingen, Germany
Houguo Xu, Yellow Sea Fisheries Research Institute (CAFS), China
Copyright © 2022 Hoerterer, Petereit, Lannig, Johansen, Conceição and Buck. This is an open-access article distributed under the terms of the Creative Commons Attribution License (CC BY). The use, distribution or reproduction in other forums is permitted, provided the original author(s) and the copyright owner(s) are credited and that the original publication in this journal is cited, in accordance with accepted academic practice. No use, distribution or reproduction is permitted which does not comply with these terms.
*Correspondence: Christina Hoerterer, Christina.Hoerterer@awi.de