- 1The Feinstein Institutes for Medical Research, Northwell Health, Manhasset, NY, United States
- 2Division of Endocrinology, Diabetes & Bone Disease, Icahn School of Medicine at Mt. Sinai, New York, NY, United States
- 3Azevan Pharmaceuticals, Bethlehem, PA, United States
Inflammation contributes to many chronic conditions. It is often associated with circulating pro-inflammatory cytokines and immune cells. GLP-1 levels correlate with disease severity. They are often elevated and can serve as markers of inflammation. Previous studies have shown that oxytocin, hCG, ghrelin, alpha-MSH and ACTH have receptor-mediated anti-inflammatory properties that can rescue cells from damage and death. These peptides have been studied well in the past century. In contrast, GLP-1 and its anti-inflammatory properties have been recognized only recently. GLP-1 has been proven to be a useful adjuvant therapy in type-2 diabetes mellitus, metabolic syndrome, and hyperglycemia. It also lowers HbA1C and protects cells of the cardiovascular and nervous systems by reducing inflammation and apoptosis. In this review we have explored the link between GLP-1, inflammation, and sepsis.
1 Introduction to GLP-1
Glucagon-like peptide-1 (GLP-1) is a peptide hormone that is produced in the intestine and in multiple other sites that are known for their role in regulating glucose metabolism. GLP-1 is also involved in multiple other physiological processes including appetite, cardiovascular function, and inflammation (1).
Acute Inflammation is central to in-vivo responses to a wide range of challenges including viral and bacteriological infections, and to host repair processes. Chronic inflammation, on the other hand, is associated with conditions like type 2 diabetes, metabolic syndrome, obesity, cancer, arthritis, and bowel diseases like Crohn’s disease and ulcerative colitis (2). Our recent studies have revealed the anti-inflammatory properties of several peptide hormones such as hCG, oxytocin, ghrelin, and vasopressin.(3-6) In this review article, we focus on the anti-inflammatory properties of the incretin hormone ‘Glucagon-like Peptide-1 (GLP-1). Known for promoting glucose homeostasis and weight loss, the anti-inflammatory properties of GLP-1 suggest that it may also blunt inflammation and protect against organ damage (3–6).
2 Functions of GLP-1 and its receptors
Glucagon-like peptide-1 (GLP-1) is a 30-31 amino acid long incretin that is produced when proglucagon undergoes post-translational processing. This glucose-lowering agent is secreted by intestinal enteroendocrine L-cells in response to nutritional and inflammatory stimuli and by neurons in the nucleus of the solitary tract in the brainstem. GLP-1 activates a seven transmembrane G protein coupled receptor, GLP-1R. GLP-1 receptors are expressed in pancreatic islet β-cells, pulmonary epithelial cells, atrial cardiac myocytes, vagal afferent neurons, neurons in a number of brain regions, as well as cells lining gastric pits and small intestinal mucosa. The GLP-1R can couple to the Gs or Gq proteins, leading to increases in intracellular cAMP and/or Ca2+ levels and activation of PKA, Epac-2, phospholipase C and ERK1/2 signal transduction pathways. Activation of GLP-1R by GLP-1 or other exogenous agonists, including exendin-4 and liraglutide, decreases inflammatory responses in several animal models like rat heart and whole animal model. The hypoglycemic activity of GLP-1 is associated with the stimulation of glucose-dependent insulin secretion, inhibition of glucagon production and regulation of islet cell proliferation, differentiation, and survival. Under physiological conditions, GLP- 1 is rapidly degraded by dipeptidyl peptidase-4 (DPP- 4) after it is released (7).
3 Discovery of GLP-1
In 1923, Charles Kimball and John Murlin, in an attempt to purify commercial insulin, precipitated a pancreatic fraction that had a hyperglycemic effect (8). Identifying it as a secreted factor, they named it ‘Glucagon’ or ‘Glucose Agonist’. In 1959, Roger Unger et al., developed the first antibody that could be used in a radioimmunoassay to detect glucagon in tissue samples and blood (9, 10). In 1966, Ellis Samols, Vincent Marks and others confirmed the presence of glucagon-like immunoreactivity in extra-pancreatic tissue, especially the intestine. Subsequently in 1967, Samols and Marks reported glucagon-like material in pancreatectomized humans, indicating its extra-pancreatic origin (11). In 1968, Roger Unger demonstrated that intraduodenal administration of glucose increased the levels of a circulating glucagon-like substances (9, 10). In contrast to glucagon, the intestinal glucagon-like material stimulated the release of insulin. It was clear that glucagon and the glucagon-like material were distinct entities, and immunohistochemical studies revealed that intestinal cells that were positive for the glucagon-like material had a different morphology from glucagon secreting α-cells. The cells that made glucagon-like material were called L-cells. In 1970 the glucagon precursor, proglucagon, was identified. In the pancreas, proglucagon undergoes post-translational cleavage yielding two fragments. One was a mature glucagon and the other was called the proglucagon fragment. In 1980, the intestinal glucagon-like material, glicentin, was identified along with a smaller species named oxyntomodulin in 1982. Collectively, these studies suggested that proglucagon undergoes tissue-specific processing resulting in formation of glicentin and oxyntomodulin in the intestine, and glucagon plus the N-terminal fragment of glicentin in the pancreas. In the 1980s Joel Habener described a new glucagon-related peptide encoded in the anglerfish preproglucagon cDNA. Subsequently two glucagon-related peptides were identified in rat, bovine, hamster, and human proglucagon. These two peptides are now called glucagon-like peptides 1 and 2 (GLP-1 and GLP-2) as shown in Figure 1 “The proglucagon precursor (12–16).
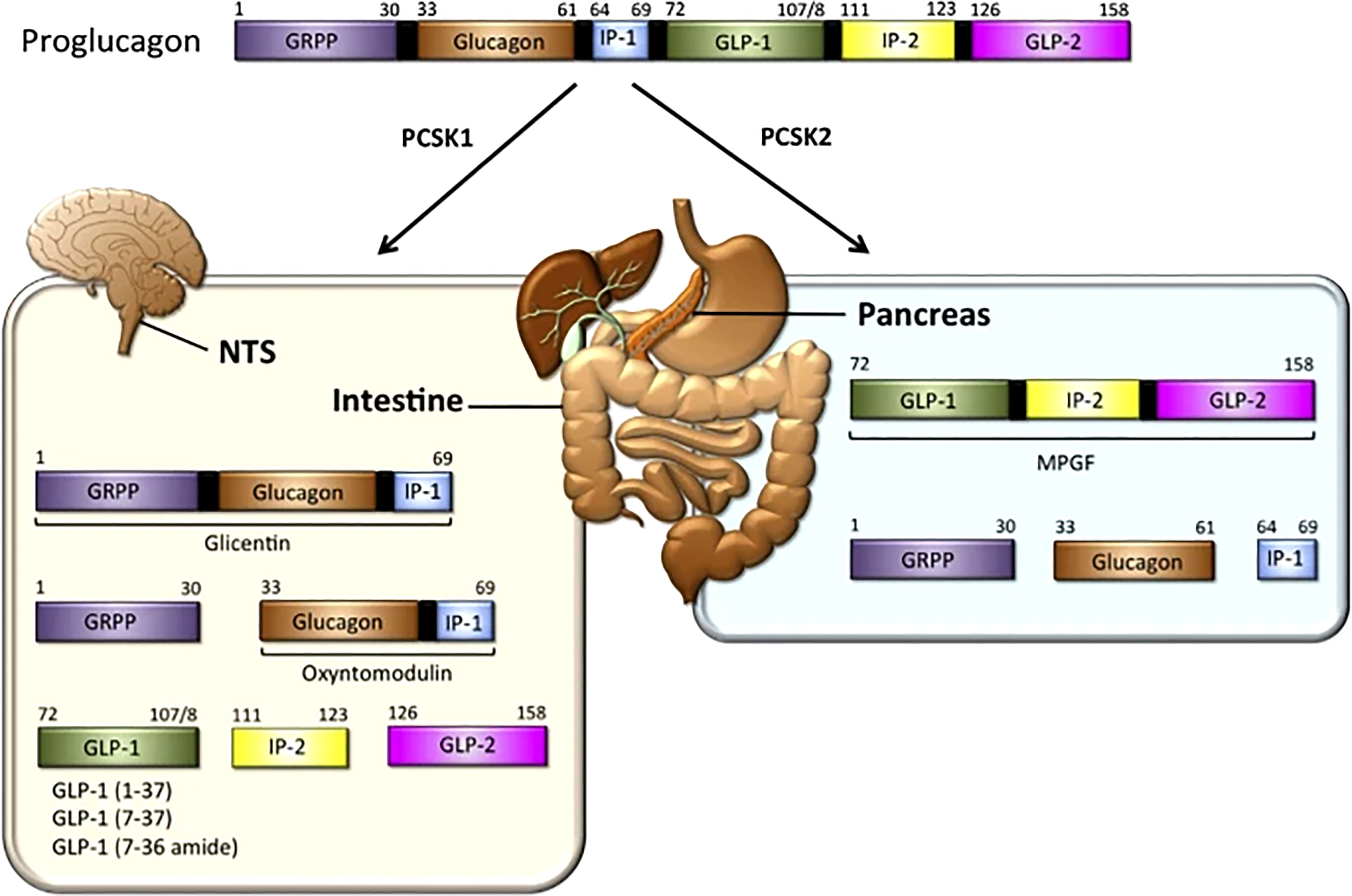
Figure 1 GLP-1 synthesis: In the intestine — The proglucagon precursor gives rise to oxyntomodulin, GLP1 (and its two equipotent, truncated derivatives) and GLP-2. Like the GLPs, the intervening peptides (IP-1 and IP-2) may also have physiological functions (12, 13). In the pancreas, the proglucagon precursor yields glucagon and the glicentin-related pancreatic peptide (GRPP) (12–16) Figure modified from article 12. Figure from Open access (Molecular Metabolism) permissible to re-use under a CC-BY 4.0 license.
4 GLP-1 receptor
The GLP-1 receptor is a member of the secretin subfamily (B1) of G-protein coupled receptors (GPCRs). It consists of 463 amino acids (17). These amino acids are arranged in seven transmembrane (7TM) alpha-helices with an N-terminal domain that is located extracellularly and a C-terminal domain that is intracellular. The transmembrane helices are connected by three extracellular and three intracellular loops (17, 18). Ligand binding to its receptor occurs in two stages. The first step involves the binding of the extracellular domain to the C-terminus of the ligand. This causes a conformational shift that leads to attachment of the N-terminus of the ligand to the 7TM domain (18–20). (Figure 2) for a more detailed figure, refer to reference 18.
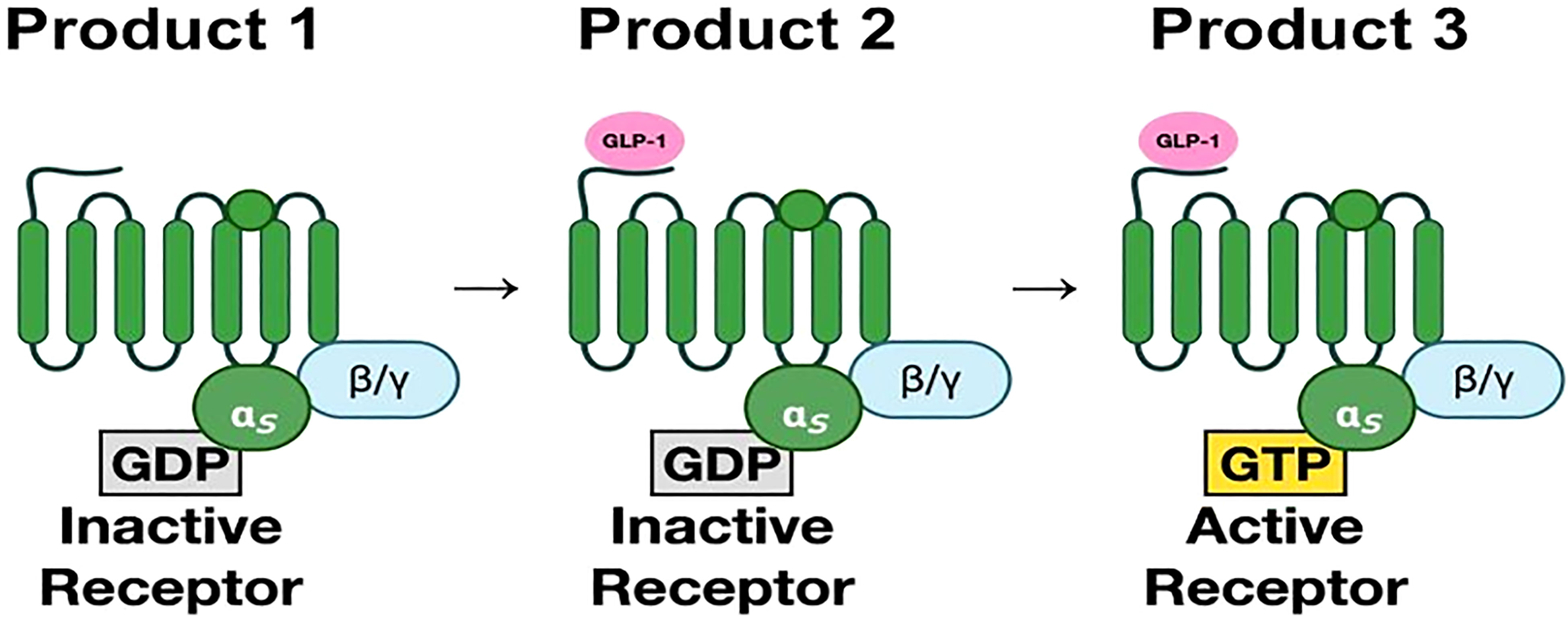
Figure 2 (Original by authors of the manuscript): The seven transmembrane alpha-helices are bound to G-protein subunits (19). These consist of alpha subunit and beta-gamma subunit complexes bound to GDP. In the inactive state, the α-subunit is bound to GDP. Upon binding of GLP-1, GDP is replaced by GTP, which then activates the α-subunit. The α-subunit and GTP complex activate signaling cascades through adenylyl cyclase and phospholipase C (20).The third intracellular loop is most important in receptor signaling.
GLP-1 receptor signaling occurs primarily through the Gαs stimulatory G protein (21). Coupling of Gαs and Gαq in beta cells of pancreas lead to an increase in cAMP by activation of adenylyl cyclase and phosphoinositol 3 kinase (PI3K) pathway. cAMP activates PKA and Epac-2 signal transduction pathways (22). PKA and Epac-2 inhibit the K-channel, altering Kv currents leading to calcium influx as well as calcium release from the endoplasmic reticulum. This results in calcium-induced release of insulin granules (23). PKA and Epac-2 also activate cyclin D and CREB, leading to beta-cell proliferation, differentiation, and a decrease in endoplasmic reticulum stress response (24). Exenatide decreases ER stress in response to synthetic stressors (25). In mouse models, exendin-4 increases beta cell proliferation by activation of epidermal growth factor receptors (26). Human beta cells exposed to GLP-1 show increased beta cell proliferation (24, 25) (Figure 3).
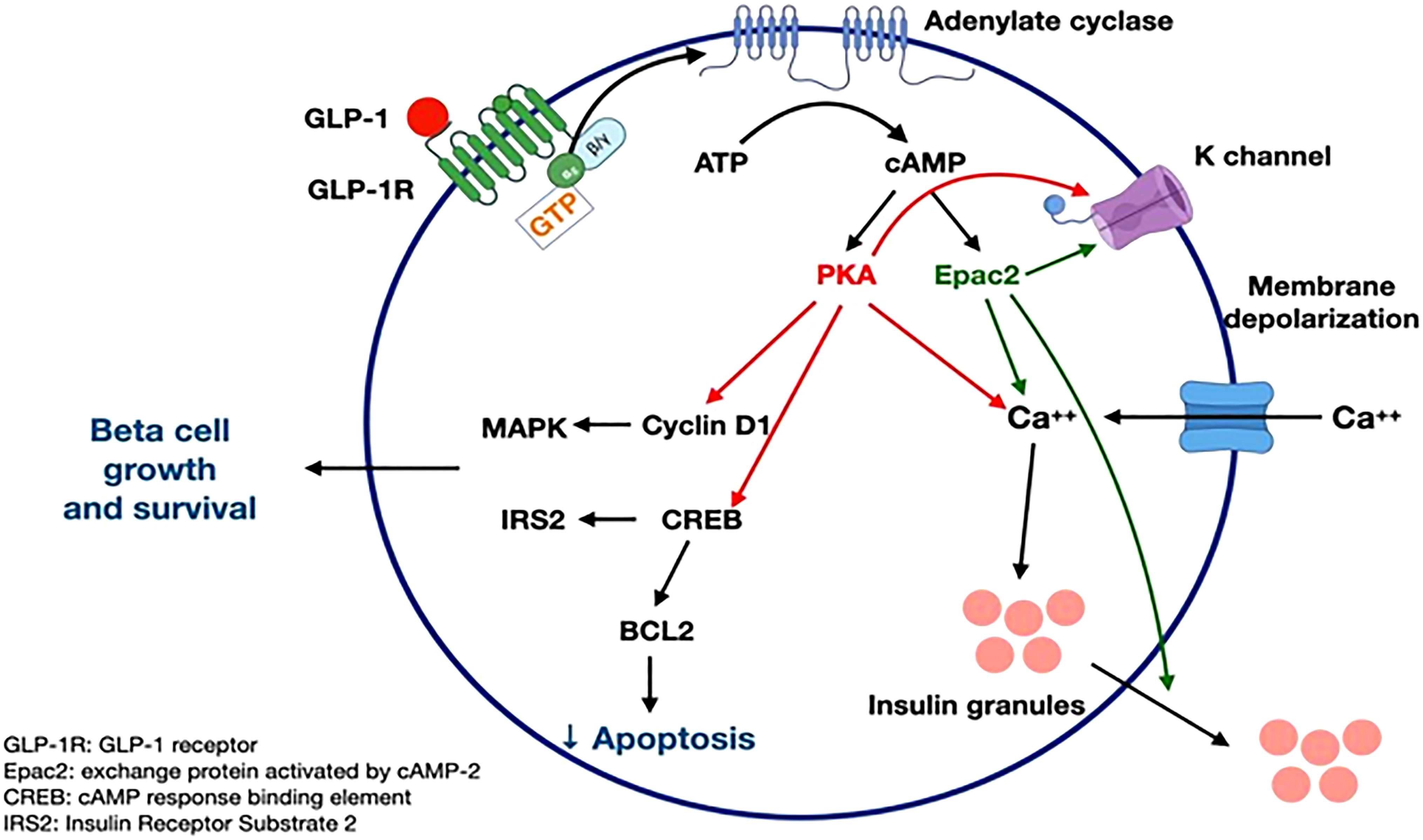
Figure 3 GLP-1 receptor signaling (21-26). Figure modified from article 23. Figure from Open access (Gastroenterology) permissible to re-use under a CC-BY 4.0 license.
Activation of the GLP-1 pathways decreases the inflammatory response in multiple models. GLP-1 Analog liraglutide improves vascular function in polymicrobial sepsis by reduction of oxidative stress and inflammation (24, 27–31). Exendin in diabetic mice diminishes inflammatory responses by increasing the expression of regulatory T cells (32). Liraglutide has anti-inflammatory effects on endothelial cells by decreasing activation of NF-kB, inhibiting TNF-alpha, and increasing nitric oxide production (28). Like GLP-1 agonists, dipeptidyl peptidase-4 (DPP-4) inhibitors, which block the degradation of GLP-1, also cause attenuation of the inflammatory responses. Sitagliptin decreases the LPS-inflammatory response by inhibiting the NF-kB pathway. This leads to decreased production of proinflammatory cytokines including TNF-α, IL-6, IL-1β and decreased expression of COX-2 in cardiomyocytes (33).
5 GLP-1 and various organs
GLP-1 has been shown to carry out numerous protective and regulatory functions in different organ systems. The functions are illustrated in the Figure (Figure 4).
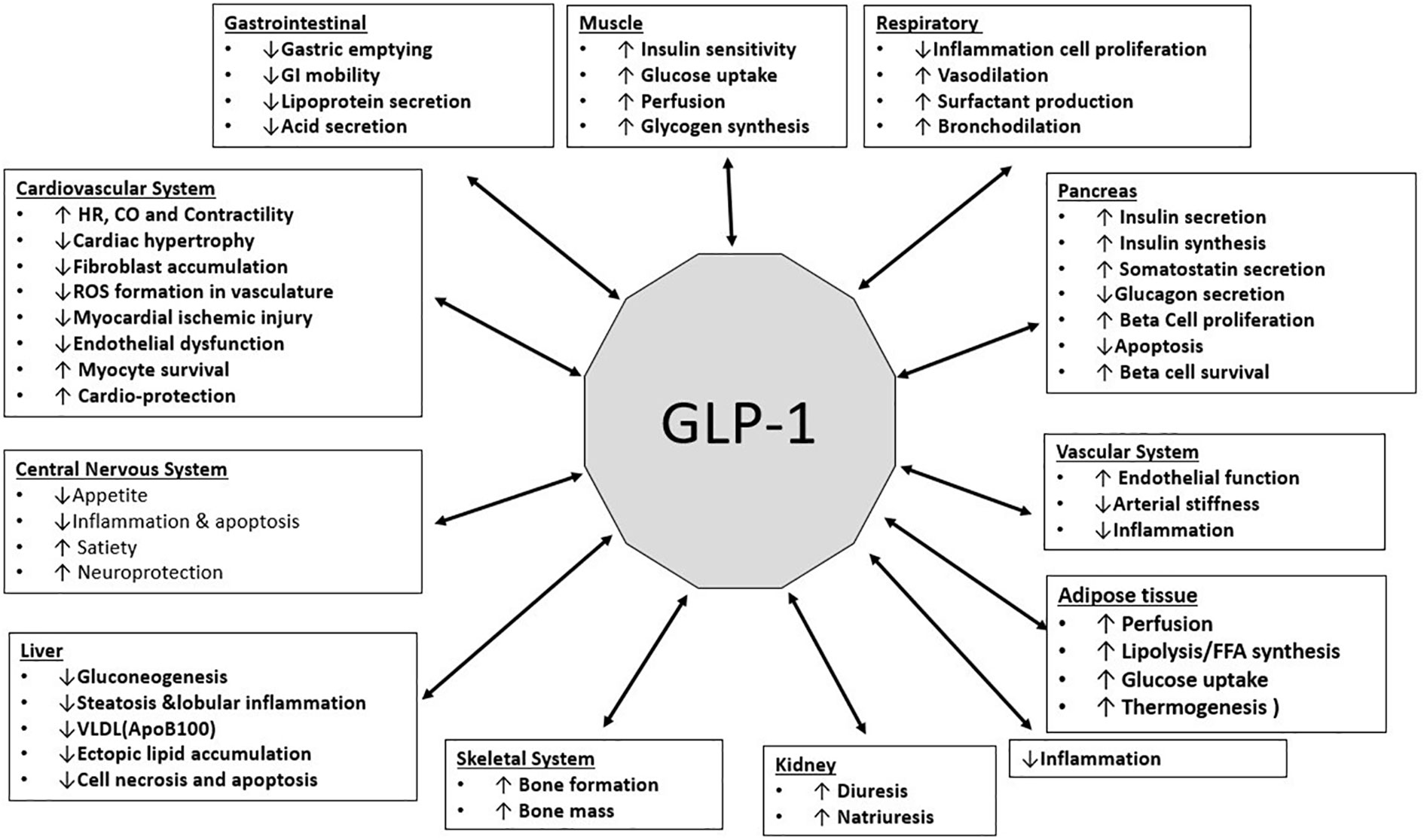
Figure 4 (Original by authors of the manuscript): Effects of GLP-1 on various organ systems (34–39).
6 GLP-1: anti-inflammatory effects
6.1 Cardiovascular system
The antioxidant and anti-inflammatory effects of GLP-1 protect the cardiovascular system. GLP-1 levels are elevated in patients post myocardial infarction. Administration of GLP-1 analogs (or DPP-4 inhibitors, which inhibit the degradation of GLP-1), decreased cardiovascular and thrombotic complications in animal models of LPS-induced sepsis. They also suppressed inflammation and formation of reactive oxygen species (ROS) in vasculature, resulting in vasorelaxation and amelioration of hypotension. Moreover, reduced organ damage by thrombotic occlusion in the lung has been reported in LPS-induced sepsis due to improvement in microvascular circulation by GLP-1 analogs. In a polymicrobial model of sepsis induced by cecal ligation and puncture, a GLP-1 analog ameliorated vascular inflammation and oxidative stress by improving endothelial function (28)
In a cardiac fibrosis model, the GLP-1 analogue liraglutide reduced vascular reactivity, cardiac hypertrophy, fibroblast accumulation, collagen deposition and MCP-1 production (40). Another GLP-1 analogue, exendin-4, also prevented cardiac remodeling and diastolic dysfunction in an experimental diabetes model. This was associated with a reduction in macrophage infiltration, lower expression of IL-1β and IL-6, and an increase in IL-10 in the heart (41).
GLP-1 improved left ventricular function in patients with chronic heart failure and in dogs with dilated cardiomyopathy. Survival rates after myocardial infarction also improved after GLP-1 administration. Sitagliptin, a DPP-4 inhibitor, improves myocardial response in coronary artery disease patients. LPS-induced cardiac dysfunction recovered in DPP-4 deficient rats after treatment with sitagliptin. Exendin-4 and DPP-4 deficiency prevented vasoconstriction and multiple organ injury after LPS treatment, and improved survival in endotoxemic rats (31).
In animal studies, GLP-1 and its analogs reduced macrophage infiltration in blood vessels, and production of pro-inflammatory cytokines such as IL-6, IL-1β, TNF-α, and CRP. It has been speculated that liraglutide, a GLP-1 analog, suppresses cytokine release in bacterial septic shock and in SARS-CoV-2 viral sepsis. GLP-1 analogs and DPP-4 inhibitors have shown promise in animal models of cardiovascular disease. Studies in humans should be done (42, 43) (Table 1).
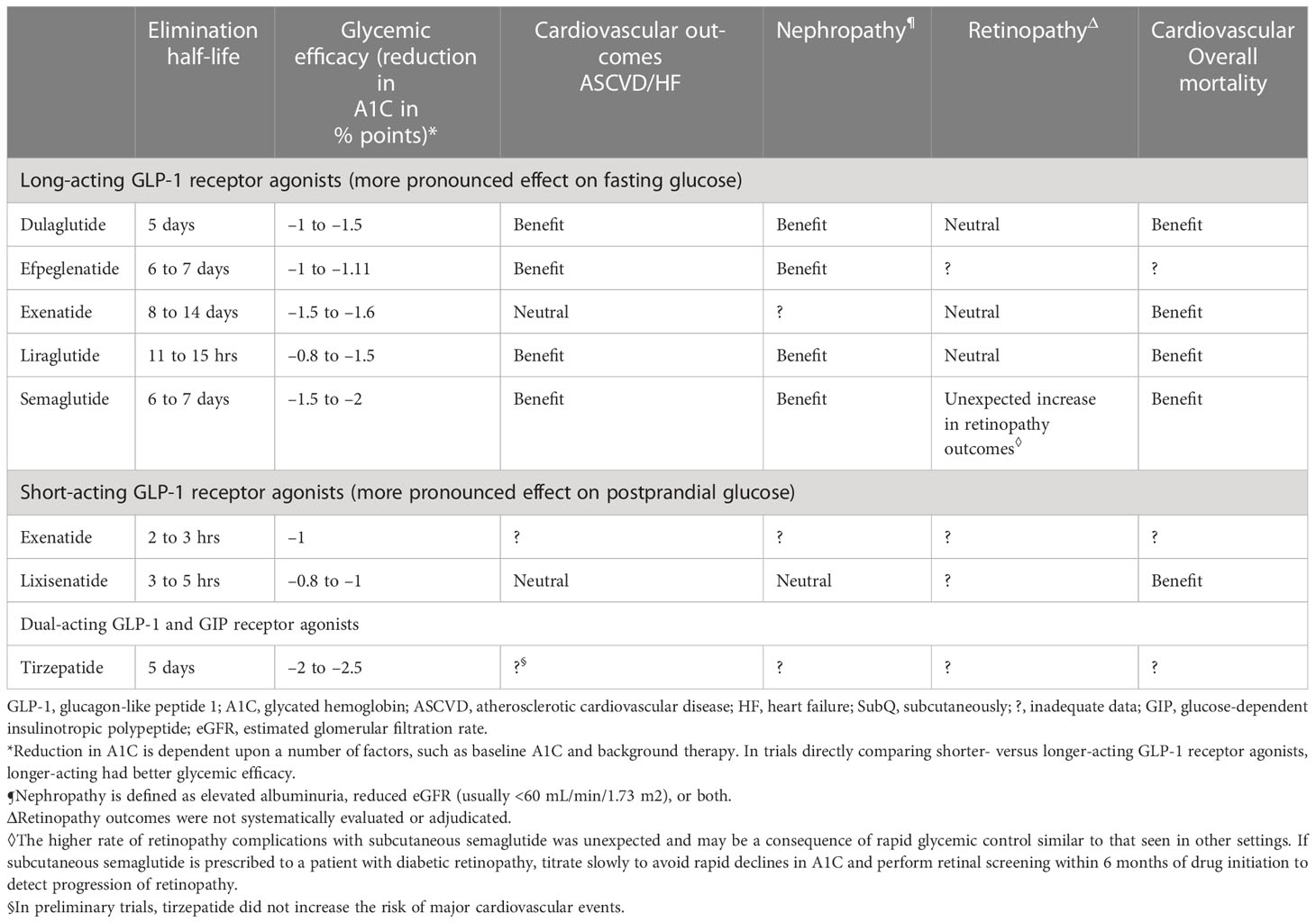
Table 1 Efficacy of GLP-1 agonists efficacy (adapted from UpToDate) (44).
6.2 Gastrointestinal system
GLP-1 is secreted into the distal intestine by enteroendocrine L cells in response to nutrient ingestion (42). GLP-1 receptors are widely distributed in the gastrointestinal tract, pancreas, heart, lungs, kidneys, and nervous system. These receptors contribute to the wide range of physiological functions (45). Besides metabolic effects, GLP-1 improves mucosal integrity and diminishes inflammation (42, 46). Exendin-4, a GLP-1 mimetic peptide, decreases the production of pro-inflammatory cytokines, and diminishes the enteric immune response. GLP-1 decreases production of pro-inflammatory cytokines, mainly by downregulating NF-κB phosphorylation and nuclear translocation (45).
Several recent studies have suggested that GLP-1 should be considered as a treatment for a wide range of intestinal diseases, including Inflammatory bowel diseases, intestinal mucositis, coeliac disease and short bowel syndrome (45). GLPs, (including GLP-1, GLP-2 and DPP-4) have recently gained increased attention from researchers studying Inflammatory bowel diseases (IBDs).
IBDs including Crohn’s disease and ulcerative colitis are chronic relapsing-remitting diseases with multifactorial etiologies and complex pathogenesis. The Incidence and prevalence of IBDs are rising globally. GLPs including GLP-1 regulate weight and glycemia. GLP-1 also inhibits gastric emptying, decreases food ingestion, and increases crypt cell proliferation. It also improves intestinal growth and nutrient absorption. GLPs have been proposed to improve tissue healing of injured epithelium, regulate T-cell growth and function, control innate immune cells such as macrophages and dendritic cells, and lower pro-inflammatory cytokines in IBD (47) (Table 2).
6.3 Hepatobiliary system
GLP-1 based therapies have shown promise in liver diseases e.g. non-alcoholic fatty liver disease (NAFLD) and non-alcoholic steatohepatitis (NASH). In recent years, the prevalence of non-alcoholic fatty liver disease (NAFLD) has continued to rise, and 10%-25% of NAFLD cases progress to non-alcoholic steatohepatitis (NASH). 10%-15% of NASH cases will develop into hepatocellular carcinoma, approximately 700,000 people die from the disease each year (53).
Nonalcoholic steatohepatitis is associated with inflammation of the liver, driven by an aberrant accumulation of fat. In rats fed with a high-fat diet, treatment with liraglutide, a GLP-1R analog, reduced steatosis and lobular inflammation compared to the saline-injected group. Exendin-4, a GLP-1R agonist, was shown in another study to lower hepatic production of the inflammatory markers TNF-, IL-1, and IL-6, as well as macrophage markers cluster of differentiation 68 (CD68), and F4/80 in mice fed a western-type (high fat) diet (54).
C-reactive-protein (CRP) is produced by the liver and is a marker of inflammation. Liraglutide produced a significant decrease in the mean concentration of CRP in a retrospective investigation of 110 obese patients with type 2 diabetes mellitus, indicating its potential as an anti-inflammatory drug. Exenatide plus metformin caused a significant reduction in baseline CRP and TNF-α. These findings show that GLP-1-based treatments improve fatty liver disease in rats and humans via reducing inflammation (42).
NAFLD is associated with cell death and fibrosis that ultimately progress to cirrhosis. In obese patients with NAFLD, Fibroblast growth factor-21 protein (FGF21) and RNA levels are higher in the liver. Treatment with GLP-1R agonists reduced the level of FGF21. This supports its use in cirrhosis. Note that 80% of patients who develop hepatocellular carcinoma had cirrhosis beforehand (55, 56).
GLP-1RA significantly reduced cell necrosis and apoptosis, the two major forms of liver cell death. Hepatic cell death mainly includes two forms: apoptosis and cell necrosis. Gupta et al. showed that a GLP-1RA significantly reduced cell necrosis and apoptosis.The reduction of abdominal visceral adiposity by GLP-1RAs results in a reduction in liver fat content that can alleviate NAFLD. The ability of GLP-1 to reduce fat is due to its binding to a specific GLP-1R present in adipose tissue (57). Vendrell et al. confirmed the expression of GLP-1R in mature adipose cells by the detection of the mRNA and protein (58). A 6-month-long treatment with GLP-1RAs in obese patients with T2DM resulted in significant reductions in intrahepatic lipids (IHL). In addition, the median relative reduction in IHL was 42% (53) (Figure 5).
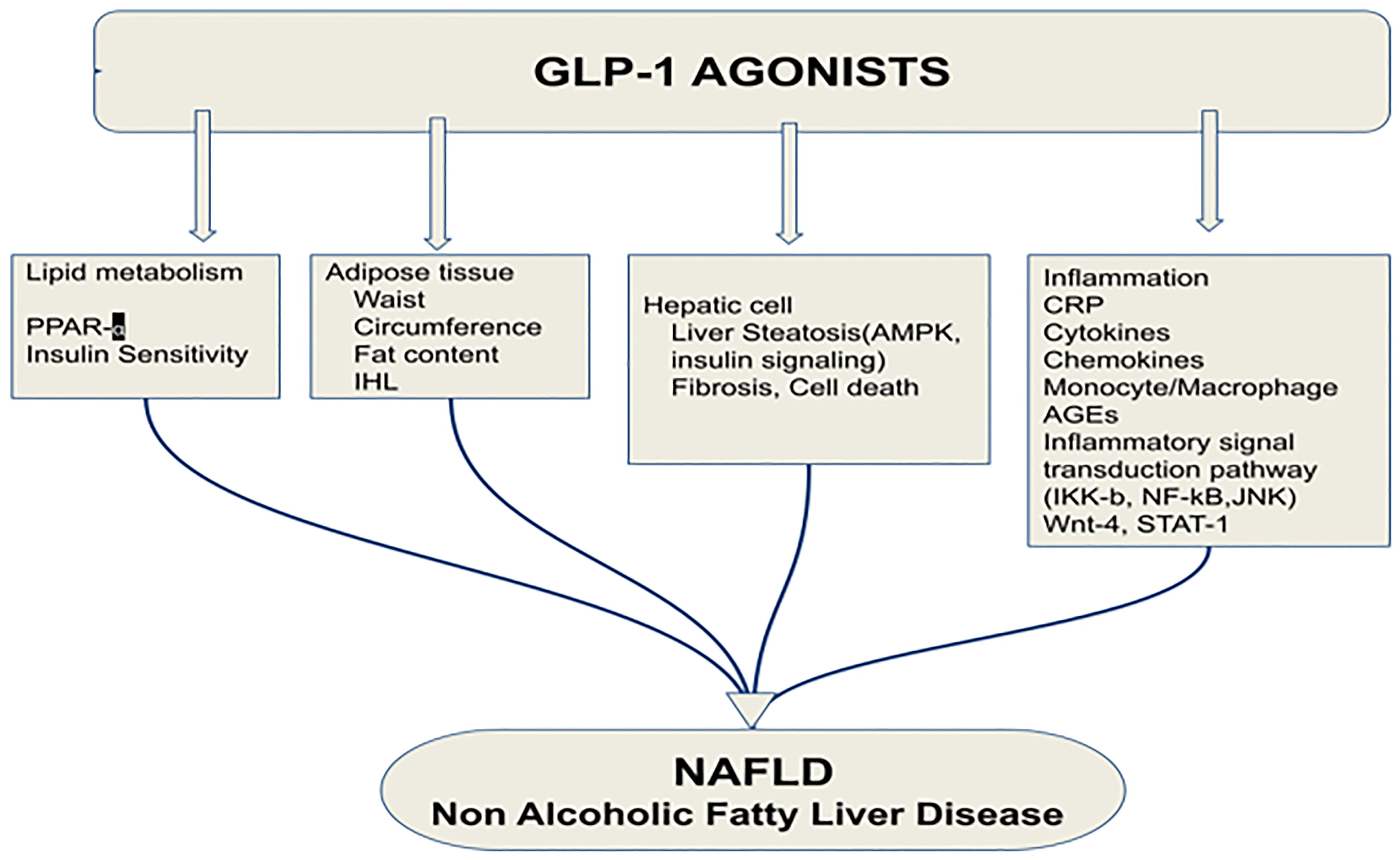
Figure 5 Effects of glucagon-like peptide-1 receptor agonist on non-alcoholic fatty liver disease. PPAR-α, Peroxisome proliferator-activated receptor; IHL, intrahepatic lipids; AMPK, AMP-activated protein kinase; CRP, C reactive protein; AGEs, Advanced glycation and end products; JNK, c-Jun NH2-terminal kinase; GLP-1RA, Glucagon-like peptide-1 receptor agonist; NAFLD, Non-alcoholic fatty liver disease (53). Modified figure from reference 53. Figure from Open access (World Journal of Gastroenterology) permissible to re-use under a CC-BY 4.0 license).
6.4 Central nervous system
Glucagon-like peptide-1 is produced in the brainstem and has numerous functions, including neuroprotection (59–61) GLP-1 and GLP-1 analogs can cross the blood-brain barrier (62–68) GLP-1 receptors have been observed in the neurons of the nucleus tractus solitarius that project to GLP-1R–expressing regions in the hindbrain, hypothalamus, including the paraventricular nucleus (PVN), dorsal medial nucleus of the hypothalamus, and arcuate nucleus (ARC) (42, 64, 66, 67, 69, 70). GLP-1-based therapies have anti-inflammatory effects on multiple tissues (42, 53, 71–73).
Chronic inflammation is a significant risk factor for many neurodegenerative disorders, e.g., Alzheimer’s disease and Parkinson’s disease (42, 74–78).
6.4.1 Parkinson’s disease
The prevalence of Parkinson’s disease has been rising in recent years (79, 80). It is the second most common chronic neurodegenerative disease and affects between 1% - 2% of people above age 60 and 4% of those above age 80 (81–87). Parkinson’s disease occurs when dopaminergic neurons in the substantia nigra pars compacta form Lewy bodies and gradually die (88–90). The Lewy body is an abnormal aggregate containing alpha-synuclein. Most Parkinson’s disease treatments focus on managing symptoms by replacing dopamine and improving dopaminergic signaling, but these treatments fail to address the underlying cellular degeneration (64, 91). Since dopamine breaks down to form reactive oxygen species, it may contribute to disease progression (92, 93). Activation of microglia plays a crucial role in spontaneous Parkinson’s Disease in humans (64, 94–96). MPTP (1-methyl-4-phenyl-1,2,3,6-tetrahydropyridine) induces Parkinson’s disease in rodents. MPTP is a pro-drug for the neurotoxin MPP+ (1-methyl-4-phenylpyridinium). This agent destroys dopaminergic neurons in the substantia nigra (81, 97–104).Exendin- 4, a GLP-1 R agonist, has inhibitory effects on microglial activation and greatly reduces the expression of TNF-α and IL-1β caused by MPTP (67, 105–107). Exendin-4 inhibits 6-hydroxydopamine (6-OHDA)-induced dopaminergic cell death in neuronal culture. The intraventricular administration of GLP-1 protects mice from MPTP-induced dopaminergic cell loss (64, 86, 108, 109).
6.4.2 Alzheimer’s disease
Alzheimer’s disease is the most common form of dementia; it is responsible for 60–70% of cases (110, 111). About 1 person in 9 (10.8%) in the US population age 65 and older has AD (112). People 65+ years of age in Europe had a pooled incidence rate of AD of 19.4 per 1000 person-years (113–115). The Alzheimer’s disease population increased by 5% from age 65 to 73, 13.1% from age 75 to 84, and 32% from age 85 and older (112, 116). Alzheimer’s disease was the seventh-leading cause of death in 2020 and 2021 (112).
In AD, IL-1 beta is significantly increased in the frontal cortex and hippocampus and may contribute to cognitive dysfunction by promoting the synthesis of amyloid precursor protein (117, 118). GLP-1 therapies may have preventive and restorative effects on Alzheimer’s disease (42, 119). Exogenous GLP-1 (7–36) amide administration inhibited IL-1 beta transcription and prevented beta-induced amnesia and cell death (36, 59, 113, 114). Also, it restores learning and memory by stimulating LTP (long-term potentiation) (60, 120–122). In a rodent model, neuroinflammation was reduced due to suppression of TNF-alpha when GLP-1 exenatide (20 ug/kg/day) was given intraperitoneally. The peptide improved memory and prevented the loss of hippocampal neurons (111, 123). Treatment with liraglutide in a mouse model of Alzheimer’s disease reduced the inflammatory response in the cortex by decreasing the number of activated microglia (60, 65, 107, 124, 125). Mice that express two human mutant genes linked to early-onset Alzheimer’s disease develop a chronic inflammatory response (126). In these animals, D-Ala2-GIP reduces the activation of microglia and astrocytes in the brain, decreasing the release of pro-inflammatory cytokines and oxidative stress (127).Microglia and astroglia express GIP receptors (128, 129). Activating them reduces central inflammatory responses. GIP receptor activation increases microglia expression of key growth factors such as brain-derived neurotrophic factor(BDNF), glial cell-line derived neurotrophic factor (GDNF), and nerve growth factor (NGF) in a phosphoinositide 3-kinase (PI3K) and protein kinase A (PKA) dependent manner (130) (Figure 6).
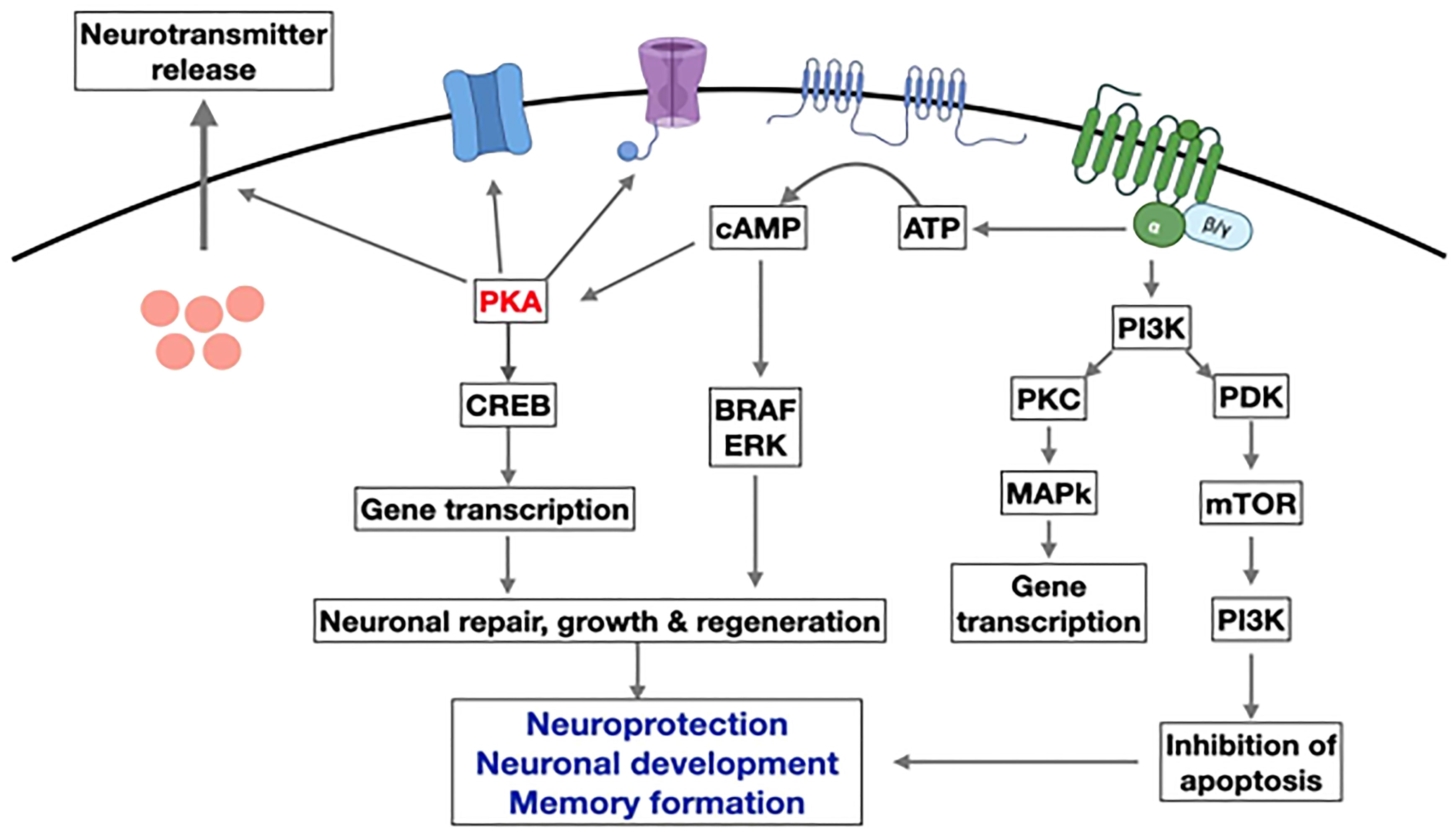
Figure 6 Overview of the main pathways induced by GLP-1 in neurons. Activation of the GLP-1R activates adenylyl cyclase and increases cAMP levels. This activates PKA and other downstream kinases related to growth factor signaling. GLP-1 supports neurogenesis, reduces inflammation, and inhibits apoptosis while improving learning and memory in the hippocampus.(modify from reference 130) (AATP, adenosine triphosphate; cAMP, Cyclic adenosine monophosphate; CREB, cAMP response element binding protein; PKA, protein kinase A; PI3K, phosphatidylinositol-3 kinase; PKC, protein kinase c; mTOR, Mammalian target of rapamycin; ERK, extracellular signal-regulated kinase; BRAF, v-raf murine sarcoma viral oncogene homolog B1.) Figure modified from reference 130, Open access (Peptides journal) permissible to re-use under a CC-BY 4.0 license).
Brain irradiation has been demonstrated to increase the expression of IL-6, IL-1β, and IL-12p70 cytokines. Liraglutide reduces the proinflammatory cytokine gene expression caused by X-ray irradiation (42, 131).
In a study on rats, when cultured astrocytes were stimulated by LPS, IL-1b mRNA expression increased temporally. GLP-1 therapy decreased IL-1b mRNA production compared to the LPS alone-treated cultures (67, 72). The GLP-1 suppresses TNF-alpha and associated cytokines in microglia (Figure 7).
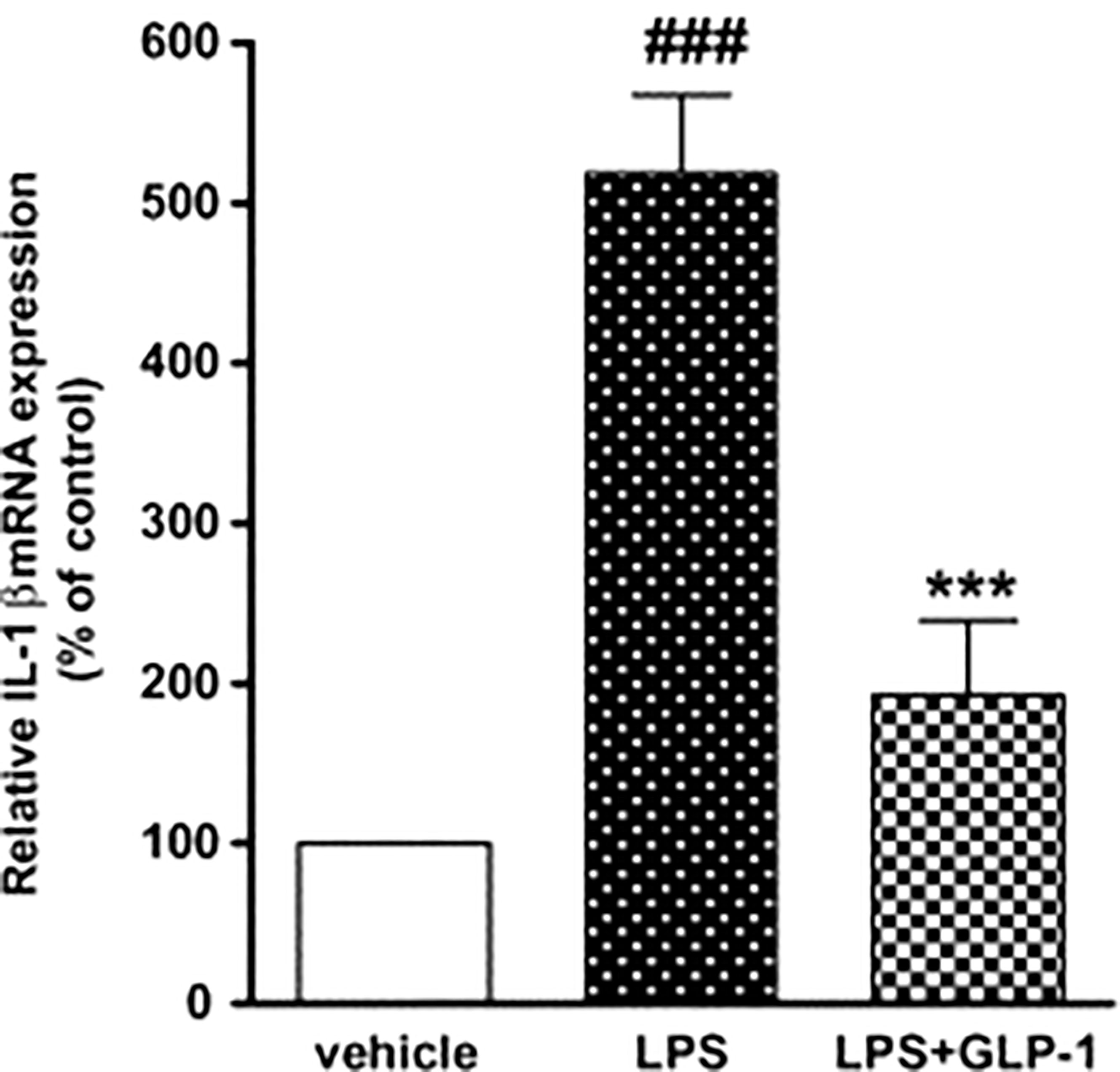
Figure 7 Impact of GLP-1 on LPS-induced IL-1b mRNA production in rat astrocytes. ELISA investigation was performed at 360 min after LPS (100 ng/mL) or vehicle treatment with or without GLP-1 (1 mM) (72). Data represent the mean ± SEM. ***p<0.001. Figure modified from Open access (Neuroscience Research Journal) permissible to re-use under a CC-BY 4.0 license). ###p<.001.
6.5 Stroke models
Strokes in the elderly can cause permanent neurological damage and are among the leading causes of death. Patients who have hyperglycemia and diabetes mellitus type 2 (T2DM) have a higher stroke frequency than those who do not have these conditions (132, 133). Stimulating GLP-1Rs with exendin-4 reduces brain damage and improves stroke outcomes (108, 132, 134, 135). Exendin-4 suppresses oxidative stress, inducible nitric oxide synthase (iNOS) expression, and cellular apoptosis after ischemia/reperfusion injury (135, 136).
It is well known that inflammation contributes to the progression of brain damage following ischemia/reperfusion injury, and that COX-2 is a significant mediator of oxidative damage (132, 137). Activation of GLP-1Rs has anti-inflammatory effects in cerebral ischemia. COX-2 expression in rats was reduced when they were treated with exendin 9-39 (antagonist) after ischemia was induced (132) (Figure 8).
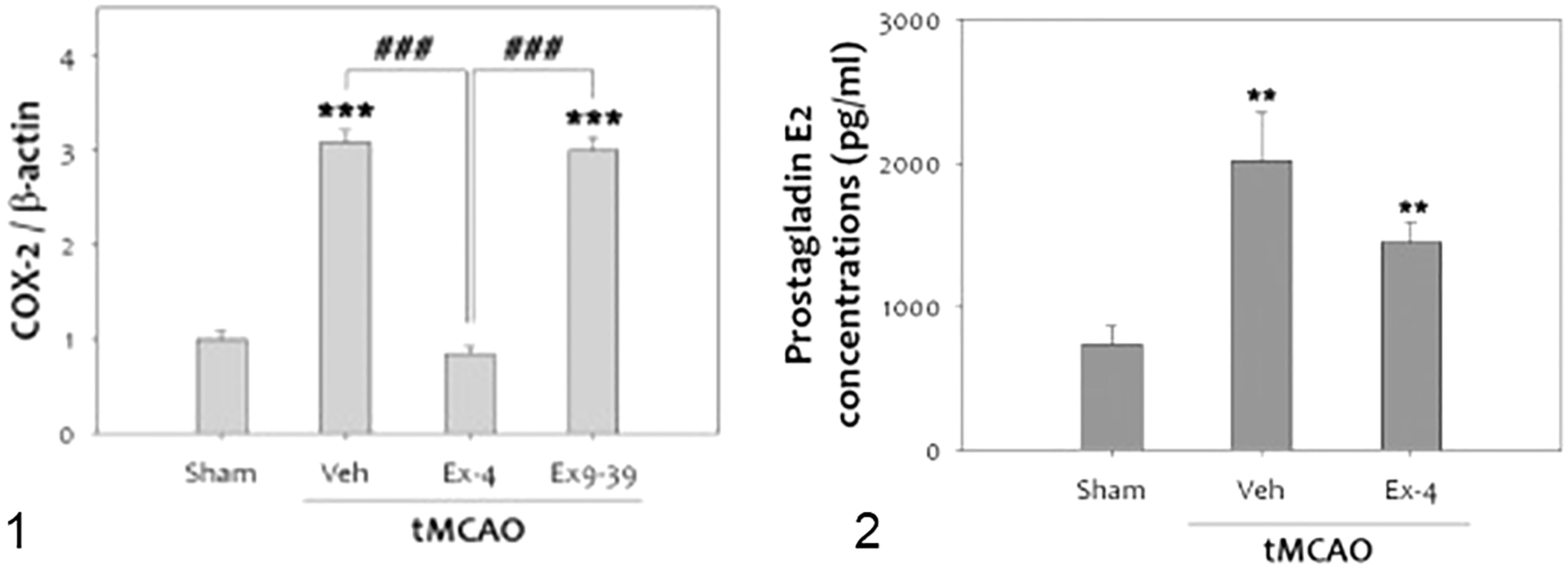
Figure 8 tMCAO, Transient middle cerebral artery occlusion (132): 1-The level of COX-2 was significantly increased at 48 h after tMCAO. Treatment with ex-4 restored COX-2 to the basal level after tMCAO in the rat brain. Ex9-39 treatment increased COX-2 levels as much as vehicle Group.(***p<0.001, compared to sham operated group, ###p<0.001, compared to chemical group) 2-The level of PGE2, which is product of COX-2 activity, was increased by 1 h tMCAO, but this level was attenuated by ex-4 (n =5, **p<0.01, compared to the sham-operated group. (1) Data represent the mean ± SEM. ***p<0.001, ###p<0.001, (2) Data represent the mean ± SEM. **p<0.01. Figure modified from Open access (Experimental Neurobiology) permissible to re-use under a CC-BY 4.0 license).
There was a reduction in GLP-1R expression in rat brains after cerebral ischemia. Furthermore, administration of the GLP-1R agonist exendin-4 in vivo and in vitro proved protective (132, 138, 139) (Figure 9).
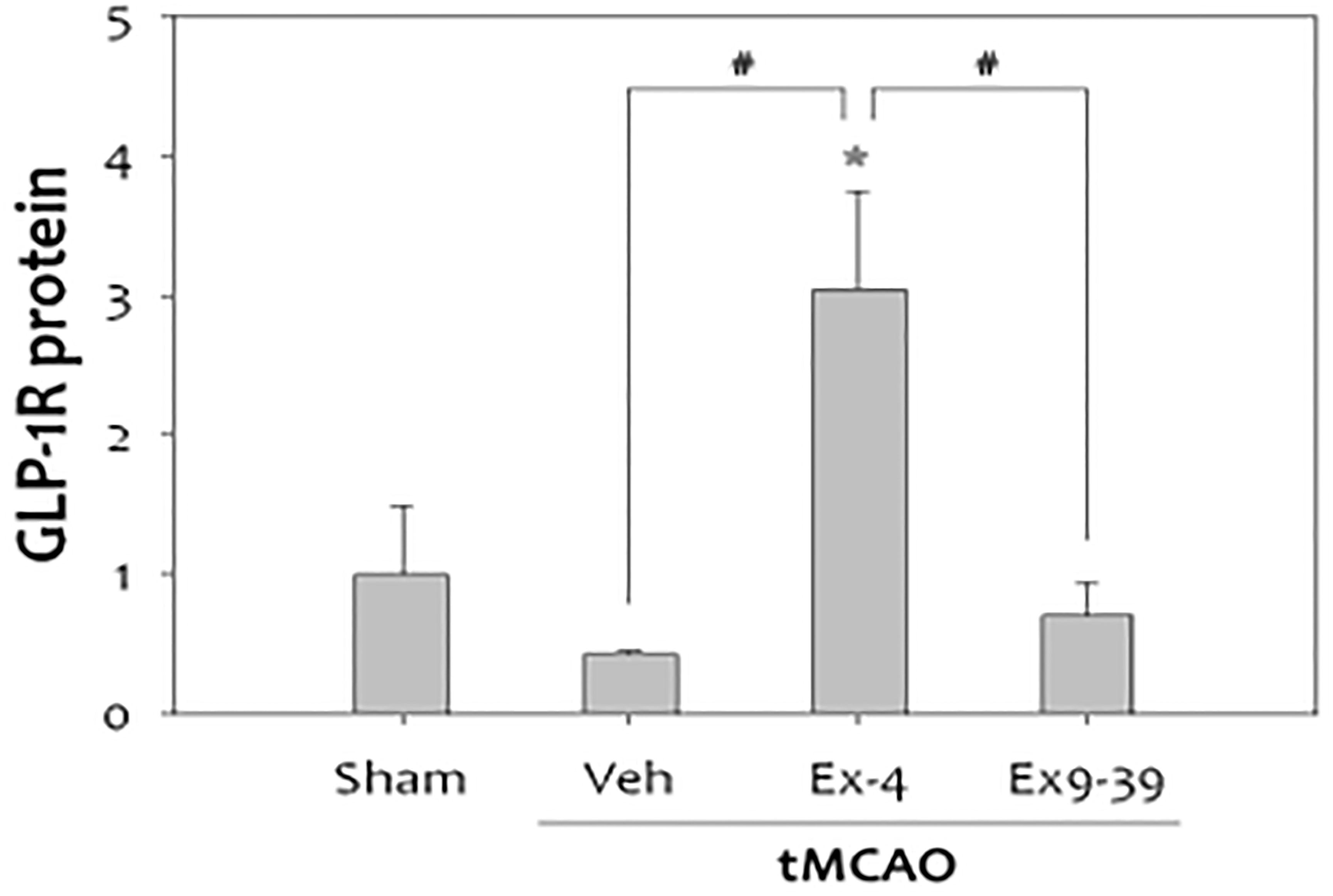
Figure 9 Treatment with exendin-4 was accompanied by increased expression of GLP-1R, while treatment with the GLP-1R antagonist, exendin-9-39 (antagonist), did not show this neuroprotective effect in stroke(n=4, *p<0.05, compared to sham-operated group, #p<0.01, compared to the chemical-treated group). Data represent the mean ± SEM. *P<.05; #P<0.01 (132). Figure modified from Open access (Experimental Neurobiology) permissible to re-use under a CC-BY 4.0 license).
GLP-1R elevates cAMP levels and activates protein kinase A (PKA) signaling. Adding GLP-1 to neurons increases cAMP, which indicates receptor activation (108, 132, 140). In mice with transient focal cerebral ischemia, exendin-4 treatment increased cAMP and activated the cAMP response element-binding protein (CREB) compared with vehicle-treated mice (135).
6.6 Respiratory system
GLP-1 plays an important role in respiratory system homeostasis (141). Glucagon-like peptide-1 receptors (GLP1-R) are found in airway structures as well as vascular and smooth muscle tissues (142). Covid victims who took GLP-1R agonists had fewer hospital admissions (143).
7 GLP-1 in obstructive lung disease and asthma
Asthma affects about 25 million people in the US and more than 330 million people world-wide (144). GLP-1 receptor agonists decreased allergic responses in asthma by preventing the activation of NF-kB leading to decreased release of proinflammatory cytokines (IL-5, IL-13, IL-33) and neutrophils, eosinophils, basophils and CD4+ T cell numbers (142, 145). Exendin-4 also relaxes bronchial smooth muscles by acting on the cAMP-PKA pathway (144).
A recent study demonstrated that GLP-1 agonists improve survival and lung function in mouse models of asthma and COPD. The results showed that GLP-1R agonists have therapeutic potential in the treatment of chronic obstructive pulmonary diseases by decreasing the severity of acute exacerbations. The anti-inflammatory effects of GLP-1 agonists in obstructive disease was evident in studies of female C57BL/6 mice. There was a decrease in CD31+ endothelial cells in lung tissues after agonist treatment (146). Trials in humans have also shown that liraglutide administration improves forced vital capacity (147).
GLP-1 causes an increase in cAMP concentration and phosphorylation of endothelial nitric oxide synthase (NOS). Nitric oxide produced as a result may be responsible for the effects of GLP-1 on vasodilation, surfactant production and bronchodilation.
GLP-1 also activates protein kinase A (PKA), which inhibits pro-inflammatory mediators such as nuclear factor kappa light chain enhancer of activated B cells (NF-kB), receptor of advanced glycation end products (RAGE) and asymmetric dimethylarginine (ADMA), an endogenous NOS inhibitor. These mediators play a central role in obesity-related asthma by increasing inflammatory cell proliferation and infiltration, airway remodeling, airway hyperreactivity and bronchoconstriction (148). A recent study showed that bronchodilation caused by GLP-1 analog Exendin-4 was inhibited by GLP-1 receptor blockers. or cAMP-PKA antagonists. Dipeptidyl peptidase-4 (DPP-4), which degrades GLP-1, is expressed in the lungs. Allergens cause upregulation of DPP-4 expression. DPP-4 activates pro-inflammatory pathways (MAPK and NF-kB) and also increases reactive oxygen species, AGE and RAGE gene expression (148).
8 GLP-1 in acute lung injuries
Acute lung injury is one of the most serious complications of sepsis. LPS administration in mice leads to endotoxemia and sepsis. Inflammation in sepsis can wash out surfactant leading to the development of acute respiratory distress syndrome (ARDS). GLP-1 agonists have a protective effect in acute lung injury and ARDS. GLP-1 promotes the production of surfactant through PKA-dependent and PKC-dependent mechanisms (149, 150). Following LPS injections in mice, co-administration of GLP-1 diminishes the decline in surfactant levels (142).
9 Studies with liraglutide
Liraglutide has benefits in the treatment of acute lung injury. It increases surfactant protein A (SPA) expression in type 2 pneumocytes (151). Pre-administration of liraglutide in mice with LPS-induced acute lung injury decreases the concentration of neutrophils and pro-inflammatory cytokines (IL-1B & IL-18) in the bronchoalveolar lavage fluid by down-regulating the expression of NLRP3 inflammasome (152). Liraglutide also reduced the levels of TNF-α, IL-1β, IL-6 and the severity of lung injury in mouse models (153). Use of liraglutide along with mesenchymal stem cells (MSCs) for treatment of acute lung injury inhibits MSC apoptosis via PKA/β-catenin pathway and improves their efficacy (141).
10 Studies with exendin-4
Decreased FOXA2 expression leads to increased mucus secretion in the lungs of asthma, COPD, and bronchiectasis patients. Exendin-4, (GLP-1R agonist), increases FOXA2 expression and restores mucus homeostasis in Pseudomonas aeruginosa infected lungs. It decreases mucin expression by pyocyanin (154). It protects against hyperglycemia-induced lung injury by reducing oxidative injury and glucose levels and stimulating the proliferation of pneumocytes. On the other hand, Oztay et al. reported that exendin-4 administration led to increased lung injury by increasing collagen accumulation around pulmonary vessels (155).
11 GLP-1 in lung fibrosis
Along with its beneficial effects in obstructive diseases and acute lung injury, GLP-1 also protects against lung fibrosis. In mice exposed to bleomycin, significant reductions in inflammation and fibrosis were seen after GLP-1 therapy due to reduction in NF-kB signaling and TGF-β1 levels (156).
12 GLP-1 in pulmonary hypertension and lung development
GLP-1 also has a beneficial effect on pulmonary hypertension. Activation of GLP-1 receptors in pulmonary arteries leads to vasodilation (157, 158). GLP-1 also plays an important part in lung development, and Liraglutide improved lung function and development in pups suffering from intrauterine growth restriction caused by ACE2-Ang(1–7)-MasR (159).
13 Summary of pulmonary effects of GLP-1
GLP-1 and its analogs are potentially beneficial in the respiratory system at most stages in life. (Figures 10, 11) (148).
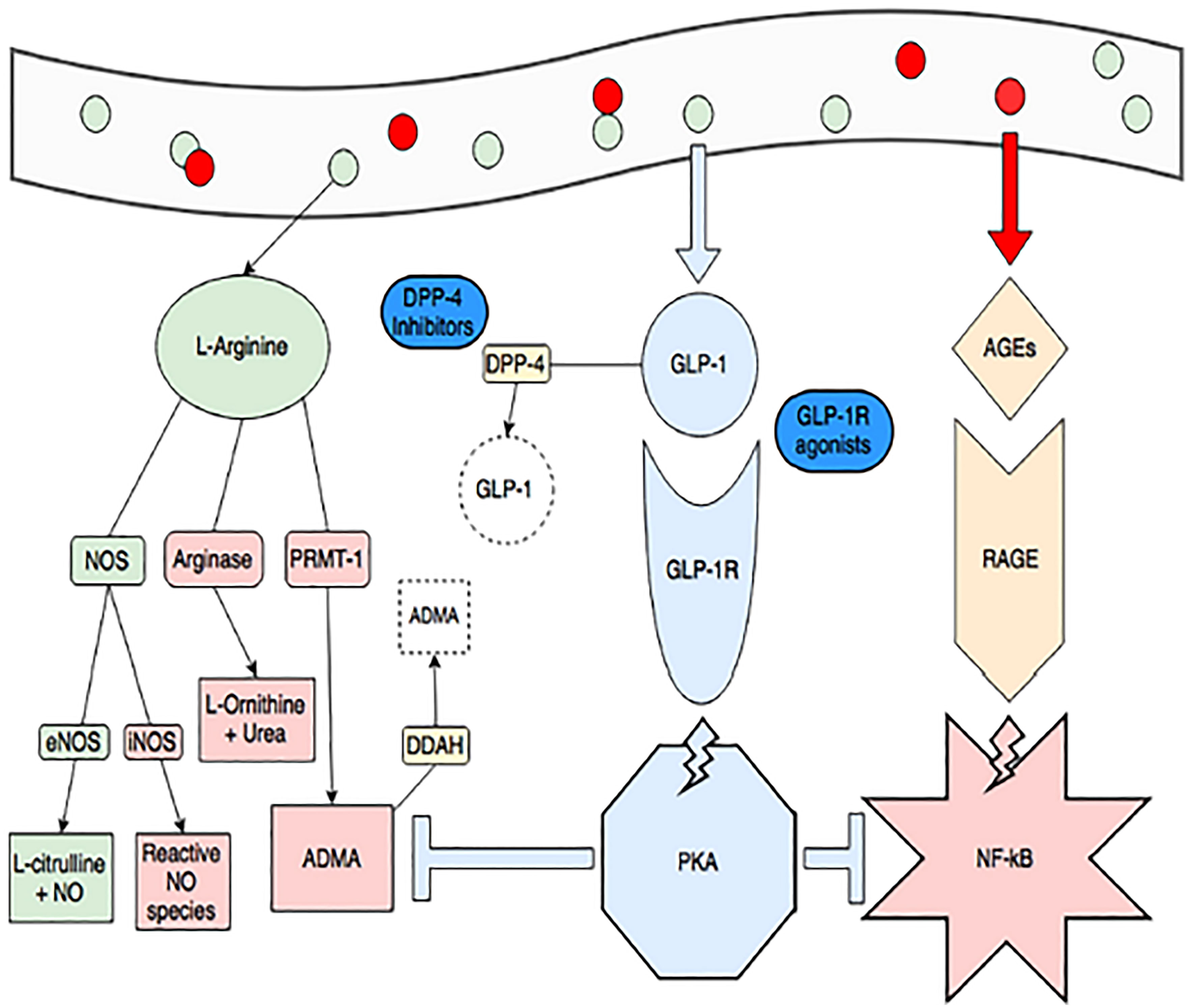
Figure 10 Obesity and consumption of foods high in advanced glycation end-products (red circles, AGEs) create a pro-inflammatory state through dysregulated arginine metabolism (increasing arginase activity and production of ADMA, in red) and activating RAGE-mediated, NF-kB inflammation (pink star). ADMA also inhibits endothelial NOS (eNOS) and increases NF-kB activity. GLP-1 production is spurred by consumption of L-arginine (green circles) and when it binds its receptor, it activates protein kinase A (blue octagon). This activity blunts RAGE-mediated inflammation and production of ADMA (blue T-lines). The GLP-1 pathway is also a target of treatments for diabetes and obesity. GLP-1 is rapidly degraded by DPP-4, and DPP-4 inhibitors (gliptins) are used to increase GLP-1. GLP-1 receptor agonists (exenatide and liraglutide) are also available (148). Figure modified from article 147 Open access (Journal of Immunology Research) permissible to re-use under a CC-BY 4.0 license).
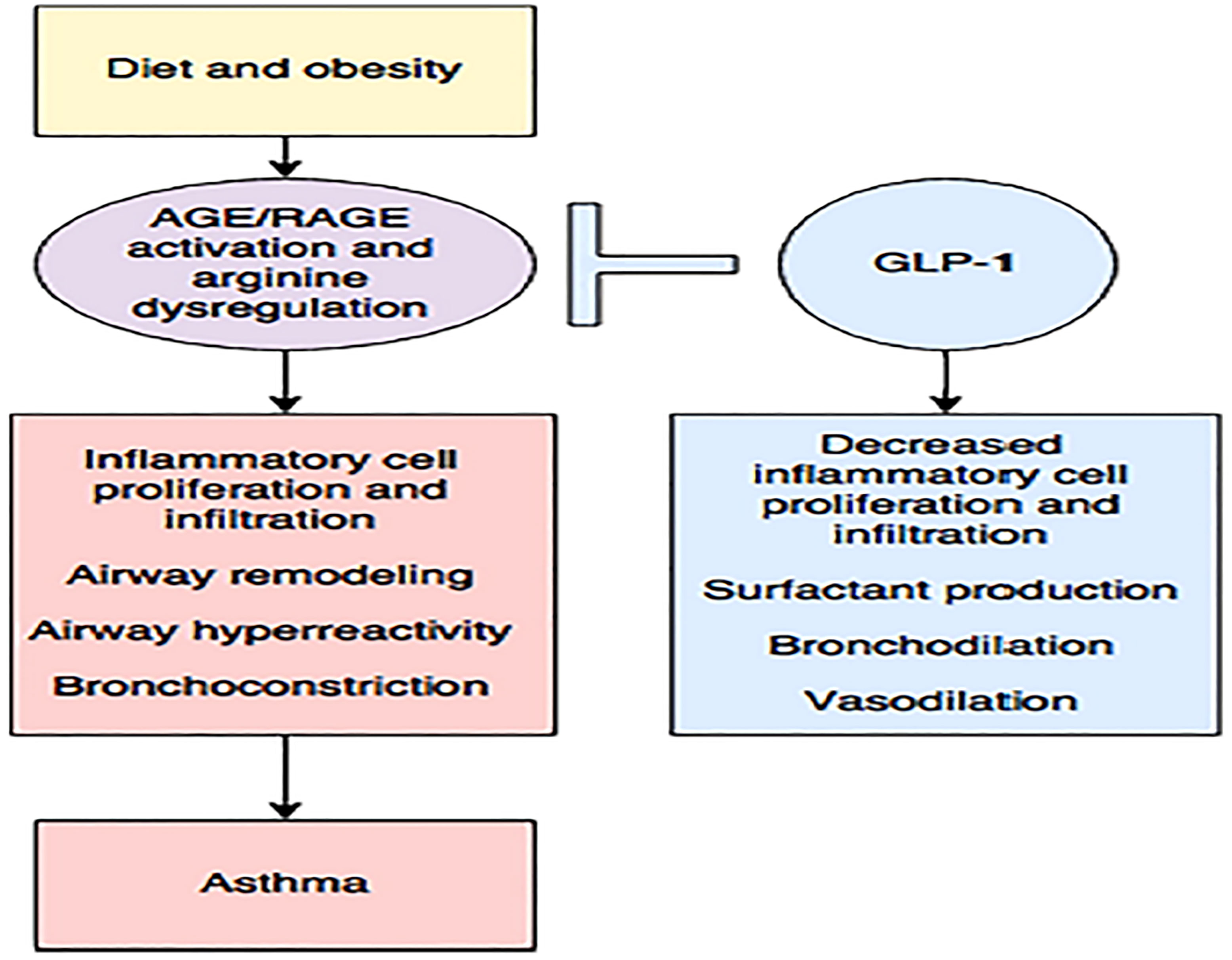
Figure 11 Diet and obesity may lead to dysregulated arginine metabolism and increase the production of advanced glycation end products (AGE) with subsequent activation of their receptor (RAGE), contributing to inflammation and asthma. The enhancing GLP-1 pathway may be the key to reducing this inflammation (148). Figure modified from articles 147 (Figure from Open access (Journal of Immunology Research) permissible to re-use under a CC-BY 4.0 license).
13.1 Renal system
Renal inflammation is a primary cause of kidney failure. Repeated kidney injuries ultimately result in end-stage renal disease. Diabetes is one cause of kidney damage. How diabetes causes inflammation is controversial, but it is known to promote the problem in both the organ and the whole body (160). Inflammatory cells, cytokines, and profibrotic growth factors cause vascular inflammation and fibrosis in diabetic nephropathy (DN). GLP-1, through its anti-inflammatory effects, reduces inflammation and fibrosis in diabetes (42).
The presence of oxidative stress in diabetic kidneys is a significant element in the inflammatory process. Oxidant/antioxidant imbalances activate NF-kB (161). GLP-1 receptor knockout mice have increased glomerular superoxide, upregulated renal NAD(P)H oxidase, and reduced renal cAMP and PKA activity. These changes lead to renal pathology. Activation of the cyclic adenosine monophosphate–protein kinase A (cAMP–PKA) pathway halts the synthesis of reactive oxygen species. GLP-1 receptor agonists activate cAMP-PKA pathway and protect against oxidative stress. Liraglutide reduced NADPH oxidase activity and increased cAMP-PKA activity in mice. It also enhanced glomerular hyperfiltration by improving glomerular nitric oxide and decreasing mesangial expansion (162, 163).
Advanced glycation end products are a common pathogenic stimulant in diabetes. They increase production of reactive oxygen species. GLP-1 agonists interfere with the signaling of receptors for advanced glycation end products. This leads to less oxidative stress and promotes protection against diabetic nephropathy (164).
Reactive oxygen species (ROS) increase the synthesis of monocyte chemotactic protein-1 (MCP-1) in diabetes (165). Increased NF-kB expression leads to higher levels of MCP-1, IL-1, and TNF-α. Macrophage activation generates a proinflammatory condition that causes structural damage to the kidneys. In the kidneys, prostaglandins serve a protective function. PGE2 synthesis is inhibited when macrophages secrete IL-1 and TNF-α. Reduced PGE2 levels hasten the inflammatory process in the kidneys (166). In rats with STZ-induced diabetes, exendin-4 decreases proteinuria and serum creatinine levels, and inhibits mesangial matrix expansion. It also protects against glomerular hypertrophy, monocyte infiltration and by reducing TGF-β, ICAM1, and CD14 in the renal cortex. Diabetes caused several histological changes in the renal tissue in another STZ-induced diabetes mouse model, including decreased height and continuity of the tubular brush border, vacuolization of proximal and distal tubular cells, necrosis of tubular and glomerular cells, hemorrhage, and mononuclear cell infiltration. Exendin-4 therapy resulted in a substantial reduction in all these lesions (167). In another similar mouse model, liraglutide resulted in restoration of catalase and glutathione peroxidase-3 levels, enzymes crucial in tissue protection against oxidative damage in kidneys (168).
GLP-1 protects diabetic kidneys. It lowers glucose levels and reduces inflammatory responses. GLP-1 receptor levels increase early in sepsis suggesting that it may have a protective role in this disorder as well (169). The use of recombinant human GLP-1 decreases the albumin content of the urine. In tubular tissue and human proximal tubular cells, it also reduces the production of multiple profibrotic factors including collagen I, alpha smooth muscle actin (SMA), fibronectin, and inflammatory proteins MCP-1 and TNF (HK-2 cells). Furthermore, in both diabetic tubular tissue and HK-2 cells, rhGLP-1 strongly decreased the phosphorylation of NF-kB and MAPK (170).
Sitagliptin inhibits inflammation and apoptosis. Use of sitagliptin in mice has been shown to decrease urine microalbumin, serum creatinine, blood glucose and blood urea nitrogen. It also decreased TNF-α receptor microRNA levels (171).
13.2 Skin
13.2.1 GLP-1 effects on wound healing
Along with its anti-inflammatory role in other organs, GLP-1 agonists play a vital role in wound healing. During normal wound healing, fibroblasts secrete collagen and multiple cytokines to regulate the process. They also produce matrix metalloproteinases [MMP] and tissue inhibitors of matrix metalloproteinases [TIMP] (172)]. Matrix metalloproteinases promote degradation of extracellular matrix proteins (173). At high levels in wounds, MMPs delay wound healing (174, 175). This activity of MMPs is modulated by the tissue inhibitors of matrix metalloproteinases (TIMPs) (176). Increased activity of TIMPs is associated with better wound healing (177).
Chronic skin wounds in patients with diabetes have high levels of MMP-9 and low levels of TIMPs with resultant high MMP-9/TIMP ratios (178). Higher MMP-9/TIMP ratios can also be seen in the serum of these patients (178, 179). C-reactive protein, another indicator of inflammation, is elevated in patients with foot ulcers (180). Use of exendin-4 in patients with chronic diabetic wounds normalizes CRP levels in serum and medium (181) (Figure 12). Low MMP-9/TIMP ratios are associated with earlier wound healing and better overall outcomes (183). Use of GLP-1, both in vitro and in-vivo, results in low levels of MMP-9 and low MMP-9/TIMP ratios in serum and medium, leading to quicker wound healing (182) (Figure 12).
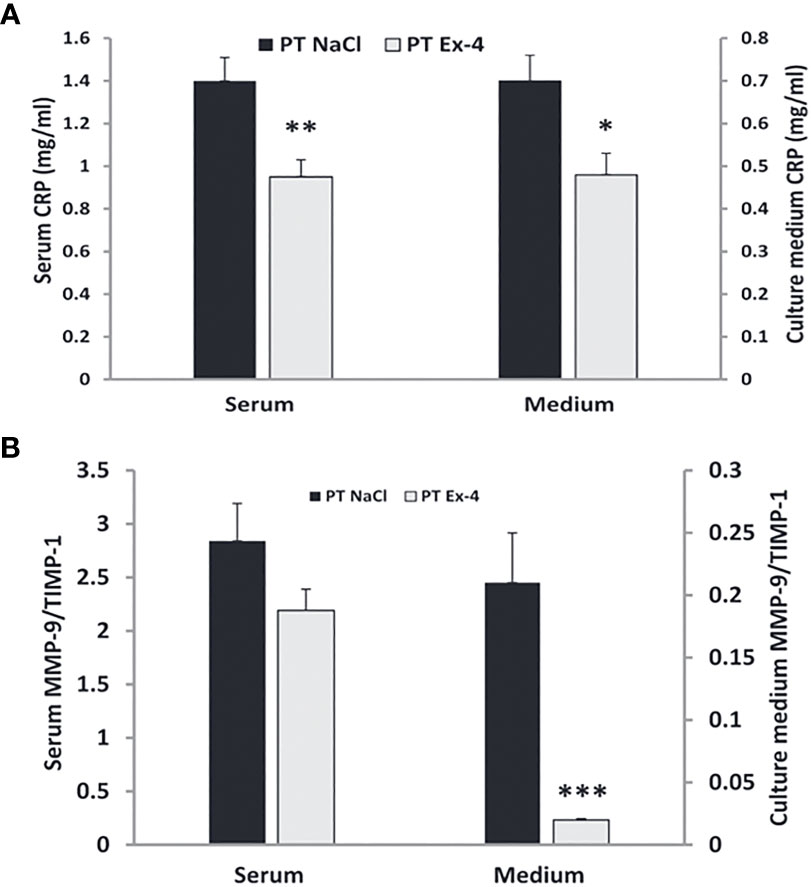
Figure 12 Use of GLP-1 Exendin-4 results in a) normalizes CRP levels in serum and medium b) low levels of MMP-9 and low MMP-9/TIMP ratios in serum and medium. (A) Data represent the mean ± SEM. *P<.05; **P<0.001, (B) Data represent the mean ± SEM. *P<.05; **P<0.01; ***P< 0.001 (182). Figure modified from article 183. Figure from Open access (European journal of pharmacology) permissible to re-use under a CC-BY 4.0 license).
13.2.2 Psoriasis
Along with effects in wound healing, GLP-1 agonists result in an improvement in psoriasis symptoms (184). Psoriasis is an inflammatory condition that is associated with excessive secretion of proinflammatory cytokines (IL-2, IL-6, IL-8, IL-12, IL-19, IL-22, IL-23, IFN-γ and TNF-α) into blood as well as dermal tissue (185). Skin biopsies show up-regulation of GLP-1 receptors in psoriasis lesions (186).
Obesity is an inflammatory disorder with dysregulated innate immune responses that cause the number of natural killer cells in the circulation to decrease (187). Obesity, along with psoriasis, is associated with chronic systemic inflammation (188). There is also a well-documented positive correlation between obesity and psoriasis (189). Treating obesity with GLP-1 agonists results in improvement of glucose tolerance and an improvement in psoriasis. The studies completed to date have shown decreases in both psoriasis area and severity index (PASI) (179, 180, 190–192). Histopathological examination of psoriasis skin lesions after 12 weeks of liraglutide showed reduced epidermal thickness as well as evidence for lesion resolution (190). A plausible mechanism through which GLP-1 improves psoriasis is by blocking expression of IL-17, IL-22, IL-23 and TNF-α through the IL-23/Th-17 pathway (191).
14 Type 2 diabetes and other metabolic disorders
14.1 Diabetes and metabolic syndrome
Chronic hyperglycemia (high blood sugar) in diabetes can lead to the production of advanced glycation end products (AGEs) and reactive oxygen species (ROS), which can damage cells all throughout the body. Cardiovascular disease, renal failure, and neuropathy are a few possible effects of this ongoing low-grade inflammation. The metabolic syndrome, on the other hand, is a combination of diseases that also cause chronic inflammation, including obesity, hypertension, diabetes, excessive blood sugar, and abnormal cholesterol levels. Inflammatory chemicals like cytokines can be produced by adipose tissue in obese people, which can increase metabolic dysfunction and lead to insulin resistance (193).
14.1.1 Liraglutide
Liraglutide decreased TNF-α, IkB, TLR2, and TLR4 mRNAs in peripheral blood mononuclear cells. Liraglutide appears to improve the metabolic profiles of obese type 2 diabetic patients and increase Sirtuin 1(SIRT1) expression, which in turn appears to suppress the pro-inflammatory NF-kB pathway. It also has an anti-inflammatory effect on vascular endothelial cells by increasing the generation of nitric oxide (193).
A sub-study of a randomized trial involving 54 with type 2 diabetes mellitus patients treated for 26 weeks with liraglutide or placebo examined whether liraglutide exerted anti-inflammatory effects through modulation of inflammatory gene expression in peripheral blood mononuclear cells (PBMCs) and Human monocytic cell line (THP-1) cells. When compared to baseline, the results showed that liraglutide dramatically lowered the production of TNF-A IL1B and raised CCL5 in PBMCs. The placebo group did not show these effects. THP-1 cells were used in an in vitro test to investigate the potential direct effects of GLP-1 receptor activation on inflammatory genes. The production of inflammatory genes by THP-1 cells was induced by LPS in the presence or absence of 2.5 nM recombinant GLP-1. GLP-1 did not influence any of the tested genes, suggesting that it has no direct effects on monocytes. GLP-1R is not expressed at the mRNA level in type 2 diabetes mellitus patients’ PBMCs or THP-1 monocytes. These results suggest that the effect of GLP-1 agonists on PBMCs are likely to be a secondary to changes in other tissues and/or the result of phenotypic alterations like weight loss or better glycemic control (194).
14.1.2 Exenatide
HbA1c and blood sugar levels are considerably lowered by each GLP-1 receptor agonist. One of the GLP-1RAs is exenatide, a synthetic GLP-1RA made from exendin-4. Exenatide 2 mg administered once weekly (QW) has been demonstrated to significantly reduce fasting plasma glucose levels. Current formulations of exenatide, a GLP-1RA based on exendin, include twice daily (BID), a long-acting GLP-1RA, and once weekly (QW), a short-acting GLP-1RA. According to the DURATION research program, exenatide 2 mg QW has shown clinical efficacy and safety in persons with type 2 diabetes (T2DM). Exenatide QW has been demonstrated to reduce HbA1c more than exenatide BID because it requires fewer injections and has higher treatment compliance (195).
14.1.3 Semaglutide
The Food Drug Administration (FDA) has approved the injectable GLP-1 receptor agonist semaglutide for use in the treatment of type 2 diabetes. The mean glycated hemoglobin level reductions in trials involving semaglutide patients have been reported to be as high as 1.8 percentage points, while the mean weight reductions have been reported to be as high as 6.5 kg (196).
14.1.4 Tirzepatide
The once-weekly dual glucose-dependent insulinotropic polypeptide-GLP-1 receptor agonist tirzepatide outperformed the selective GLP-1 receptor agonist semaglutide in patients with type 2 diabetes who were taking metformin monotherapy, according to the SURPASS trial (196).
14.2 GLP-1 and polycystic ovary syndrome
PCOS is associated with hyperinsulinemia and a decrease in circulating levels of GLP-1 and GIP, two incretin hormones. Administration of either of the two improves insulin sensitivity and glucose metabolism in patients with PCOS (197).
14.2.1 Liraglutide
In postmenopausal PCOS rat models, the effects of liraglutide on the cardiometabolic profile, the intrarenal renin-angiotensin system (RAS), and the blood pressure (BP) were investigated. Four-week-old female mice were treated with dihydrotestosterone (DHT) for 17 months and a placebo. Liraglutide was administered to postmenopausal PCOS rats over the last three weeks; and this resulted in a greater decrease in body weight, fat mass, food consumption, and insulin resistance than in control rats. Liraglutide improved both dyslipidemia and leptin levels in postmenopausal PCOS rats. In the control group, Liraglutide, only decreased intrarenal RAS transiently while increasing heart rate and decreasing blood pressure. In PCOS rats, liraglutide increased heart rate but did not affect blood pressure. Enalapril, an inhibitor of the angiotensin-converting enzyme, eliminated the BP differences between PCOS and control rats. Liraglutide and enalapril co-administration further lowered blood pressure only in control rats. In summary, Liraglutide lowered a number of cardiometabolic risk factors in postmenopausal PCOS. Hyperandrogenemia, on the other hand, prevented Liraglutide from regulating blood pressure in postmenopausal PCOS. The stimulation of intrarenal RAS by androgens may contribute to BP increases in postmenopausal PCOS (198).
In a prospective observational study of the impact of liraglutide on weight loss in obese and overweight people with PCOS, 84 obese women with PCOS were given daily subcutaneous injections of liraglutide beginning with a dose of 0.6 mg. The dose was increased to 1.2 mg and then 1.8 mg if the compound was well tolerated. The treatment lasted 4 weeks and subjects were monitored for a total of 27 weeks. They had a significant decrease in weight and BMI. Weight and atherothrombotic markers, such as endothelial function and clotting time, significantly decreased in obese women with PCOS who were given liraglutide (1.8 mg per day) vs those given placebo. In another study, liraglutide (1.8 mg) had a beneficial effect on body weight, quality of life (QOL) and depression (197, 198).
In a randomized control trial, liraglutide and a placebo were given to 72 PCOS, BMI>25, insulin-resistant women for 26 weeks. Liver fat concentration, the prevalence of nonalcoholic fatty liver disease (NAFLD), and visceral adipose tissue (VAT) were investigated. DXA dual-x-ray absorptiometry was used to measure body composition, Proton magnetic resonance spectroscopy (1H-MRS) to measure liver fat content, MRI to measure VAT (Visceral adipose tissue) and an oral glucose tolerance test to measure glucose metabolism. In comparison to placebo, liraglutide treatment reduced the prevalence of NAFLD by two-thirds, the amount of fat in the liver by 44%, visceral adipose tissue by 18%, and body weight by 5.2 kg (5.6%) (199).
14.2.2 Exenatide
A randomized single-blinded trial was conducted in 119 PCOS women without diabetes and a BMI of 30 to 45 mm/kg. Exenatide (EXE) (2 mg weekly), Dapagliflozin (DAPA) (10 mg daily), Exenatide + Dapagliflozin (2 mg weekly/10 mg daily), Dapagliflozin (10 mg) + Metformin (MET) (2000 mg extended release daily), or Phentermine (7.5 mg)/Topiramate were given to the patients for 24 weeks (46 mg extended release daily). All medications caused decreases in fasting blood sugar, testosterone, and blood pressure. Both combinations of Exenatide, Dapagliflozin plus Phentermine, Topiramate resulted in significant weight loss and waist circumference decrease. Exenatide plus dapagliflozin was the only treatment that significantly decreased (fasting) blood sugar and improved insulin sensitivity. This combination, therefore, outperformed others in terms of clinical and metabolic effects (200).
For a period of 12 weeks, a combination of exenatide plus metformin and metformin monotherapy was assessed in fifty obese/overweight women of reproductive age. Forty patients completed the study. In terms of lowering weight, body mass index (BMI), and waist circumference, combination treatment outperformed metformin monotherapy. Additionally, with combination therapy as opposed to metformin, showed lower levels of fasting glucose, oral glucose tolerance test (OGTT) 2-h glucose, and OGTT 2-h insulin. Thus, in overweight/obese women with PCOS, combination treatment is more effective than metformin alone by improving insulin sensitivity, with tolerable short-term side effects (201).
A meta-analysis of the effects of insulin sensitizers in PCOS patients showed that GLP-1 receptor agonists are superior to metformin in improving insulin sensitivity, and metformin is superior to thiazolidinediones in decreasing BMI. A combination of GLP-1 receptor agonists and metformin had little effect on menstrual frequency or serum testosterone. Metformin combined with thiazolidinediones were particularly effective in promoting the recovery of menstruation in PCOS patients. A combination of GLP-1 receptor agonists and metformin or thiazolidinediones was superior to metformin monotherapy as a treatment for hyperandrogenism (202, 203).
14.3 Obesity
Between 1960 and 1980, obesity prevalence among adults aged 20-49 was between 13% and 15% (204). An estimated 33.0% of American people aged 20 and above are overweight, 35.7% are obese, and 6.3% are severely obese, according to data from the 2009–2010 National Health and Nutrition Examination Survey (NHANES), which used measured heights and weights (205). In the United States between 2017 and 2018 adults aged 20 and above had a prevalence of obesity of 42.4% and a prevalence of severe obesity of 9.2% (206). At present 33% of US adults are overweight and are in the 40-59 age group. Further weight gain is predicted by 2030 (205, 206). Men and women had equal obesity prevalence rates overall, but women were more likely to have severe obesity than men. Non-Hispanic black men and women had the highest prevalence of severe obesity, and non-Hispanic Asian adults had the lowest (206). In June 2013, the American medical association first declared obesity a disease. Obesity is now the most prevalent chronic disease in the United States; it results in $147 billion in health care spending annually (204). GLP-1 receptor agonists decrease appetite, increase satiety, reduce food intake and decrease weight gain (207).
14.3.1 Liraglutide
The GLP-1 receptor agonist Liraglutide may only decrease appetite for a short period of time. After 10 days of treatment with Liraglutide, patients had decreased responses in the insula and putamen to food pictures vs the control group treated with insulin glargine. After 12 weeks of treatment, there were no differences between the groups. GLP agonists may initiate weight loss, but not maintain it (206).
Treatment with a GLP-1R agonist resulted in a greater weight loss than control treatment did. The GLP-1R agonist had beneficial effects on systolic and diastolic blood pressure, plasma concentrations of cholesterol, and glycemic control, but did not have a significant impact on plasma concentrations of liver enzymes. Taking the GLP-1R agonist was associated with nausea, diarrhea, and vomiting, but not with hypoglycemia (208).
Liraglutide 3.0 mg (Saxenda®; Novo Nordisk), as an adjunct to a caloric restriction and increased physical activity, has been approved for weight management in the USA and Europe. The Satiety and Clinical Adiposity Liraglutide Evidence (SCALE) Phase III trial in non-diabetic and diabetic people investigated the safety and efficacy of liraglutide 3.0 mg (once daily subcutaneous injections). In this weight management program subjects treated with liraglutide 3.0 mg experienced a dose-dependent weight loss ranging from 6.0 kg to 8.8 kg, whereas subjects treated with placebo (on diet and exercise alone) had a mean weight loss of 0.2 kg to 3.0 k (209).
14.3.2 Semaglutide
Semaglutide is a GLP-1 receptor agonist that is dosed once a week subcutaneously based on its extended half-life. The molecule binds strongly to albumin because of a large fatty acid chain attached to the lysine in position 26. A phase 2 dose-finding trial in subjects with type 2 diabetes showed clear dose-dependent effects on HbA1c and weight over 12 weeks of treatment. 1.6 mg/week resulted in an absolute weight loss of 4.82 kg compared to the placebo group’s 1.18 kg and a drop in HbA1c of up to 1.7%. As a secondary end-point, a direct comparison with liraglutide (up to 1.8 mg) was made. Semaglutide appeared to be more efficacious for weight loss than liraglutide (2.6 kg) (210).
Current trials: The significant phase 3 clinical program assessing the efficacy and safety of semaglutide (SUSTAIN) in type 2 diabetes has been completed. To avoid the requirement for subcutaneous injections and new formulations of semaglutide have been developed. An orally available product is in phase 3. This formulation is combined with the absorption enhancer SNAC (sodium N-[8-(2-hydroxy benzoyl)amino] caprylate), causing a localized increase in pH and enabling a higher solubility and protection from enzymatic degradation. According to this study, both hyperglycemia and hypoglycemia in patients with type 1 diabetes gives rise to endothelial dysfunction, oxidative stress, and inflammation and GLP-1 can be useful to counterbalance these effects. Thus, it supports the usefulness of GLP-1 and its analogs in the management of type 1 diabetes (210).
14.4 GLP-1 and type 1 diabetes mellitus
The role of GLP-1 in patients with type 2 diabetes is well-studied and well-established. Surprisingly, GLP-1 has been slow to emerge in patients with T1DM. The use of GLP-1 agonists may be considered in T1DM patients who are overweight or obese and not at glycemic goals. GLP-1 decreases inflammation in pancreas which can help in preserving beta cells and ameliorate the progression to Type 1 diabetes. Further studies are required to fully understand the role of GLP-1 in T1DM management (210).
14.5 SEPSIS
Sepsis is characterized by widespread inflammation and organ dysfunction. It continues to be a major cause of illness, disability, and death at all ages (211). Hormones in the body, such as oxytocin ghrelin, alpha MSH, ACTH and hCG, have a significant role in reducing the inflammatory response that occurs during sepsis (3–6). GLP-1 plays a crucial role in regulating the cytokine storm by binding to receptors in a wide variety of tissues including the brain, kidneys, liver, and lungs. It reduces proinflammatory processes and boosts anti-inflammatory ones throughout the body. Even though GLP-1’s use of sepsis has been encouraging in animal models, there have been no human trials. Additional research on the use of GLP-1 in patients with sepsis may further elucidate its anti-inflammatory properties and spur human studies.
15 Conclusion
GLP-1 and its agonists have opened new avenues for treatment of inflammatory diseases to mitigate organ dysfunction, septicemia, and post-sepsis syndrome. Further clinical research is required. Besides peptide hormones like ghrelin, oxytocin and hCG, consideration should be given to incretin and other related peptides.
Author contributions
SM, SP, MA, DeL, PK, SA, FN and JR conceived of the article, compiled the literature, and interpreted its contents. DeL, HY, KT, MB and JR added critical intellectual content to the manuscript and can be considered experts on the topic. All authors provided critical feedback and helped shape the research and analysis. All authors contributed to the article and approved the submitted version.
Funding
The authors declare that this study received philanthropic funding from Alan and Tatyana Forman through Altronix Inc. The funder was not involved in the study design, collection, analysis, interpretation of data, the writing of this article or the decision to submit it for publication.
Acknowledgments
The authors would like to thank Dr. Kevin J. Tracey of the Feinstein Institutes for Medical Research for his review of the manuscript.
Conflict of interest
Author MB was employed by Azevan Pharmaceuticals Inc.
The remaining authors declare that the research was conducted in the absence of any commercial or financial relationships that could be construed as a potential conflict of interest.
Publisher’s note
All claims expressed in this article are solely those of the authors and do not necessarily represent those of their affiliated organizations, or those of the publisher, the editors and the reviewers. Any product that may be evaluated in this article, or claim that may be made by its manufacturer, is not guaranteed or endorsed by the publisher.
References
1. Holst JJ. The physiology of glucagon-like peptide 1. Physiol Rev (2007) 87:1409–39. doi: 10.1152/physrev.00034.2006
2. Inflammation. National institute of environmental health sciences. Available at: https://www.niehs.nih.gov/health/topics/conditions/inflammation/index.cfm (Accessed March 30, 2023).
3. Mostel Z, Perl A, Marck M, Mehdi SF, Lowell B, Bathija S, et al. Post-sepsis syndrome - an evolving entity that afflicts survivors of sepsis. Mol Med (2019) 26:6. doi: 10.1186/s10020-019-0132-z
4. Mehdi SF, Pusapati S, Khenhrani RR, Farooqi MS, Sarwar S, Alnasarat A, et al. Oxytocin and related peptide hormones: candidate anti-inflammatory therapy in early stages of sepsis. Front Immunol (2022) 13:864007. doi: 10.3389/fimmu.2022.864007
5. Mathur N, Mehdi SF, Anipindi M, Aziz M, Khan SA, Kondakindi H, et al. Ghrelin as an anti-sepsis peptide: review. Front Immunol (2020) 11:610363. doi: 10.3389/fimmu.2020.610363
6. Yoo SK, Mehdi SF, Pusapati S, Mathur N, Anipindi M, Lunenfeld B, et al. Human chorionic gonadotropin and related peptides: candidate anti-inflammatory therapy in early stages of sepsis. Front Immunol (2021) 12:714177. doi: 10.3389/fimmu.2021.714177
7. Kim W, Egan JM. The role of incretins in glucose homeostasis and diabetes treatment. Pharmacol Rev (2008) 60:470–512. doi: 10.1124/pr.108.000604
8. Kimball CP, Murlin JR. Aqueous extracts of pancreas: III. some precipitation reactions of insulin. J Biol Chem (1923) 58:337–46. doi: 10.1016/S0021-9258(18)85474-6
9. Unger RH, Eisentraut AM, McCall MS, Madison LL. Glucagon antibodies and an immunoassay for glucagon. J Clin Invest (1961) 40:1280–9. doi: 10.1172/JCI104357
10. Unger RH, Ketterer H, Eisentraut AM. Distribution of immunoassayable glucagon in gastrointestinal tissues. Metabolism (1966) 15:865–7. doi: 10.1016/0026-0495(66)90156-9
11. Samols E, Tyler J, Megyesi C, Marks V. Immunochemical glucagon in human pancreas, gut, and plasma. Lancet (1966) 2:727–9. doi: 10.1016/s0140-6736(66)92982-5
12. Drucker DJ. Biologic actions and therapeutic potential of the proglucagon-derived peptides. Nat Clin Pract Endocrinol Metab (2005) 1:22–31. doi: 10.1038/ncpendmet0017
13. Kieffer TJ, Habener JF. The glucagon-like peptides. Endocr Rev (1999) 20:876–913. doi: 10.1210/edrv.20.6.0385
14. Müller TD, Finan B, Bloom SR, D'Alessio D, Drucker DJ, Flatt PR, et al. Glucagon-like peptide 1 (GLP-1). Mol Metab (2019) 30:72–130. doi: 10.1016/j.molmet.2019.09.010
15. Drucker DJ, Habener JF, Holst JJ. Discovery, characterization, and clinical development of the glucagon-like peptides. J Clin Invest (2017) 127:4217–27. doi: 10.1172/JCI97233
16. Whiting L, Stewart KW, Hay DL, Harris PW, Choong YS, Phillips AR, et al. Glicentin-related pancreatic polypeptide inhibits glucose-stimulated insulin secretion from the isolated pancreas of adult male rats. Physiol Rep (2015) 3:e12638. doi: 10.14814/phy2.12638
17. Marzook A, Tomas A, Jones B. The interplay of glucagon-like peptide-1 receptor trafficking and signalling in pancreatic beta cells. Front Endocrinol (2021) 12. doi: 10.3389/fendo.2021.678055
18. de Graaf C, Donnelly D, Wootten D, Lau J, Sexton PM, Miller LJ, et al. Glucagon-like peptide-1 and its class b G protein–coupled receptors: a long march to therapeutic successes. Pharmacol Rev (2016) 68:954–1013. doi: 10.1124/pr.115.011395
19. Buteau J, Foisy S, Joly E, Prentki M. Glucagon-like peptide 1 induces pancreatic -cell proliferation Via transactivation of the epidermal growth factor receptor. Diabetes (2003) 52:9. doi: 10.2337/diabetes.52.1.124
20. Couvineau A, Laburthe M. The family B1 GPCR: structural aspects and interaction with accessory proteins. CDT (2012) 13:103–15. doi: 10.2174/138945012798868434
21. Zhang Y, Sun B, Feng D, Hu H, Chu M, Qu Q, et al. Cryo-EM structure of the activated GLP-1 receptor in complex with a G protein. Nature (2017) 546:248–53. doi: 10.1038/nature22394
22. Drucker DJ, Philippe J, Mojsov S, Chick WL, Habener JF. Glucagon-like peptide I stimulates insulin gene expression and increases cyclic AMP levels in a rat islet cell line. Proc Natl Acad Sci U S A (1987) 84:3434–8. doi: 10.1073/pnas.84.10.3434
23. Baggio LL, Drucker DJ. Biology of incretins: GLP-1 and GIP. Gastroenterology (2007) 132:2131–57. doi: 10.1053/j.gastro.2007.03.054
24. Yang F, Zeng F, Luo X, Lei Y, Li J, Lu S, et al. GLP-1 receptor: a new target for sepsis. Front Pharmacol (2021) 12:706908. doi: 10.3389/fphar.2021.706908
25. Yusta B, Baggio LL, Estall JL, Koehler JA, Holland DP, Li H, et al. GLP-1 receptor activation improves β cell function and survival following induction of endoplasmic reticulum stress. Cell Metab (2006) 4:391–406. doi: 10.1016/j.cmet.2006.10.001
26. Fusco J, Xiao X, Prasadan K, Sheng Q, Chen C, Ming Y-C, et al. GLP-1/Exendin-4 induces β-cell proliferation via the epidermal growth factor receptor. Sci Rep (2017) 7:9100. doi: 10.1038/s41598-017-09898-4
27. Lu C, Xie T, Guo X, Wu D, Li S, Li X, et al. Glucagon-like peptide-1 receptor agonist exendin-4 mitigates lipopolysaccharide-induced inflammatory responses in RAW264.7 macrophages. Int Immunopharmacol (2019) 77:105969. doi: 10.1016/j.intimp.2019.105969
28. Helmstädter J, Keppeler K, Aust F, Küster L, Frenis K, Filippou K, et al. GLP-1 analog liraglutide improves vascular function in polymicrobial sepsis by reduction of oxidative stress and inflammation. Antioxid (Basel) (2021) 10:1175. doi: 10.3390/antiox10081175
29. Shah FA, Mahmud H, Gallego-Martin T, Jurczak MJ, O’Donnell CP, McVerry BJ. Therapeutic effects of endogenous incretin hormones and exogenous incretin-based medications in sepsis. J Clin Endocrinol Metab (2019) 104:5274–84. doi: 10.1210/jc.2019-00296
30. Insuela DBR, Carvalho VF. Glucagon and glucagon-like peptide-1 as novel anti-inflammatory and immunomodulatory compounds. Eur J Pharmacol (2017) 812:64–72. doi: 10.1016/j.ejphar.2017.07.015
31. Steven S, Hausding M, Kröller-Schön S, Mader M, Mikhed Y, Stamm P, et al. Gliptin and GLP-1 analog treatment improves survival and vascular inflammation/dysfunction in animals with lipopolysaccharide-induced endotoxemia. Basic Res Cardiol (2015) 110:6. doi: 10.1007/s00395-015-0465-x
32. Xue S, Wasserfall CH, Parker M, Brusko TM, McGrail S, McGrail K, et al. Exendin-4 therapy in NOD mice with new-onset diabetes increases regulatory T cell frequency. Ann N Y Acad Sci (2008) 1150:152–6. doi: 10.1196/annals.1447.049
33. Lin C-H, Lin C-C. Sitagliptin attenuates inflammatory responses in lipopolysaccharide-stimulated cardiomyocytes via nuclear factor-κB pathway inhibition. Exp Ther Med (2016) 11:2609–15. doi: 10.3892/etm.2016.3255
34. Arakawa M, Ebato C, Mita T, Hirose T, Kawamori R, Fujitani Y, et al. Effects of exendin-4 on glucose tolerance, insulin secretion, and beta-cell proliferation depend on treatment dose, treatment duration and meal contents. Biochem Biophys Res Commun (2009) 390:809–14. doi: 10.1016/j.bbrc.2009.10.054
35. Kawamori D, Shirakawa J, Liew CW, Hu J, Morioka T, Duttaroy A, et al. GLP-1 signalling compensates for impaired insulin signalling in regulating beta cell proliferation in βIRKO mice. Diabetologia (2017) 60:1442–53. doi: 10.1007/s00125-017-4303-6
36. Li Y, Cao X, Li L-X, Brubaker PL, Edlund H, Drucker DJ. Beta-cell Pdx1 expression is essential for the glucoregulatory, proliferative, and cytoprotective actions of glucagon-like peptide-1. Diabetes (2005) 54:482–91. doi: 10.2337/diabetes.54.2.482
37. Li Y, Hansotia T, Yusta B, Ris F, Halban PA, Drucker DJ. Glucagon-like peptide-1 receptor signaling modulates beta cell apoptosis. J Biol Chem (2003) 278:471–8. doi: 10.1074/jbc.M209423200
38. Park S, Dong X, Fisher TL, Dunn S, Omer AK, Weir G, et al. Exendin-4 uses Irs2 signaling to mediate pancreatic beta cell growth and function. J Biol Chem (2006) 281:1159–68. doi: 10.1074/jbc.M508307200
39. Buteau J, El-Assaad W, Rhodes CJ, Rosenberg L, Joly E, Prentki M. Glucagon-like peptide-1 prevents beta cell glucolipotoxicity. Diabetologia (2004) 47:806–15. doi: 10.1007/s00125-004-1379-6
40. Gaspari T, Brdar M, Lee HW, Spizzo I, Hu Y, Widdop RE. Molecular and cellular mechanisms of glucagon-like peptide-1 receptor agonist-mediated attenuation of cardiac fibrosis. Diabetes Vasc Dis Res (2016) 13:56–68. doi: 10.1177/1479164115605000
41. Tate M, Robinson E, Green BD, McDermott BJ, Grieve DJ. Exendin-4 attenuates adverse cardiac remodelling in streptozocin-induced diabetes via specific actions on infiltrating macrophages. Basic Res Cardiol (2016) 111:1. doi: 10.1007/s00395-015-0518-1
42. Lee Y-S, Jun H-S. Anti-inflammatory effects of GLP-1-Based therapies beyond glucose control. Mediators Inflamm (2016) 2016:3094642. doi: 10.1155/2016/3094642
43. Rakipovski G, Rolin B, Nøhr J, Klewe I, Frederiksen KS, Augustin R, et al. The GLP-1 analogs liraglutide and semaglutide reduce atherosclerosis in ApoE-/- and LDLr-/- mice by a mechanism that includes inflammatory pathways. JACC Basic Transl Sci (2018) 3:844–57. doi: 10.1016/j.jacbts.2018.09.004
44. Dungan K, DeSantis A. Glucagon-like peptide 1-based therapies for the treatment of type 2 diabetes mellitus. in: Uptodate. Post DN, (Ed.), UpToDate. Waltham, MA: UpToDateInc. Available at: https://www.uptodate.com/contents/glucagon-like-peptide-1-based-therapies-for-the-treatment-of-type-2-diabetes-mellitus.
45. Hunt JE, Holst JJ, Jeppesen PB, Kissow H. GLP-1 and intestinal diseases. Biomedicines (2021) 9:383. doi: 10.3390/biomedicines9040383
46. Lebrun LJ, Lenaerts K, Kiers D, Pais de Barros JP, Le Guern N, Plesnik J, et al. Enteroendocrine l cells sense LPS after gut barrier injury to enhance GLP-1 secretion. Cell Rep (2017) 21:1160–8. doi: 10.1016/j.celrep.2017.10.008
47. Zatorski H, Sałaga M, Fichna J. Role of glucagon-like peptides in inflammatory bowel diseases–current knowledge and future perspectives. Naunyn-Schmiedeberg’s Arch Pharmacol (2019) 392:1321–30. doi: 10.1007/s00210-019-01698-z
48. Anbazhagan AN, Thaqi M, Priyamvada S, Jayawardena D, Kumar A, Gujral T, et al. GLP-1 nanomedicine alleviates gut inflammation. Nanomed: Nanotechnol Biol Med (2017) 13:659–65. doi: 10.1016/j.nano.2016.08.004
49. Yusta B, Baggio LL, Koehler J, Holland D, Cao X, Pinnell LJ, et al. GLP-1R agonists modulate enteric immune responses through the intestinal intraepithelial lymphocyte GLP-1R. Diabetes (2015) 64:2537–49. doi: 10.2337/db14-1577
50. Al-Dwairi A, Alqudah TE, Al-Shboul O, Alqudah M, Mustafa AG, Alfaqih MA. Glucagon-like peptide-1 exerts anti-inflammatory effects on mouse colon smooth muscle cells through the cyclic adenosine monophosphate/nuclear factor-κB pathway in vitro. JIR (2018) 11:95–109. doi: 10.2147/JIR.S152835
51. Işbil Büyükcoşkun N, Güleç G, Cam Etöz B, Ozlük K. Central effects of glucagon-like peptide-1 on cold-restraint stress-induced gastric mucosal lesions. Turk J Gastroenterol (2007) 18:150–6.
52. Su Y, Liu N, Zhang Z, Li H, Ma J, Yuan Y, et al. Cholecystokinin and glucagon-like peptide-1 analogues regulate intestinal tight junction, inflammation, dopaminergic neurons and α-synuclein accumulation in the colon of two parkinson’s disease mouse models. Eur J Pharmacol (2022) 926:175029. doi: 10.1016/j.ejphar.2022.175029
53. Wang X-C, Gusdon AM, Liu H, Qu S. Effects of glucagon-like peptide-1 receptor agonists on non-alcoholic fatty liver disease and inflammation. World J Gastroenterol (2014) 20:14821–30. doi: 10.3748/wjg.v20.i40.14821
54. Wang Y, Parlevliet ET, Geerling JJ, van der Tuin SJ, Zhang H, Bieghs V, et al. Exendin-4 decreases liver inflammation and atherosclerosis development simultaneously by reducing macrophage infiltration. Br J Pharmacol (2014) 171:723–34. doi: 10.1111/bph.12490
55. Samson SL, Sathyanarayana P, Jogi M, Gonzalez EV, Gutierrez A, Krishnamurthy R, et al. Exenatide decreases hepatic fibroblast growth factor 21 resistance in non-alcoholic fatty liver disease in a mouse model of obesity and in a randomised controlled trial. Diabetologia (2011) 54:3093–100. doi: 10.1007/s00125-011-2317-z
56. Simon TG, Patorno E, Schneeweiss S. Glucagon-like peptide-1 receptor agonists and hepatic decompensation events in patients with cirrhosis and diabetes. Clin Gastroenterol Hepatol (2022) 20:1382–1393.e19. doi: 10.1016/j.cgh.2021.07.010
57. Gupta NA, Kolachala VL, Jiang R, Abramowsky C, Romero R, Fifadara N, et al. The glucagon-like peptide-1 receptor agonist exendin 4 has a protective role in ischemic injury of lean and steatotic liver by inhibiting cell death and stimulating lipolysis. Am J Pathol (2012) 181:1693–701. doi: 10.1016/j.ajpath.2012.07.015
58. Vendrell J, El Bekay R, Peral B, García-Fuentes E, Megia A, Macias-Gonzalez M, et al. Study of the potential association of adipose tissue GLP-1 receptor with obesity and insulin resistance. Endocrinology (2011) 152:4072–9. doi: 10.1210/en.2011-1070
59. Harkavyi A, Whitton PS. Glucagon-like peptide 1 receptor stimulation as a means of neuroprotection. Br J Pharmacol (2010) 159:495–501. doi: 10.1111/j.1476-5381.2009.00486.x
60. Diz-Chaves Y, Herrera-Pérez S, González-Matías LC, Mallo F. Effects of glucagon-like peptide 1 (GLP-1) analogs in the hippocampus. Vitam Horm (2022) 118:457–78. doi: 10.1016/bs.vh.2021.12.005
61. Larsen PJ, Tang-Christensen M, Holst JJ, Orskov C. Distribution of glucagon-like peptide-1 and other preproglucagon-derived peptides in the rat hypothalamus and brainstem. Neuroscience (1997) 77:257–70. doi: 10.1016/s0306-4522(96)00434-4
62. Manavi MA. Neuroprotective effects of glucagon-like peptide-1 (GLP-1) analogues in epilepsy and associated comorbidities. Neuropeptides (2022) 94:102250. doi: 10.1016/j.npep.2022.102250
63. Hunter K, Hölscher C. Drugs developed to treat diabetes, liraglutide and lixisenatide, cross the blood brain barrier and enhance neurogenesis. BMC Neurosci (2012) 13:33. doi: 10.1186/1471-2202-13-33
64. Athauda D, Foltynie T. The glucagon-like peptide 1 (GLP) receptor as a therapeutic target in parkinson’s disease: mechanisms of action. Drug Discov Today (2016) 21:802–18. doi: 10.1016/j.drudis.2016.01.013
65. McClean PL, Parthsarathy V, Faivre E, Hölscher C. The diabetes drug liraglutide prevents degenerative processes in a mouse model of alzheimer’s disease. J Neurosci (2011) 31:6587–94. doi: 10.1523/JNEUROSCI.0529-11.2011
66. Hamilton A, Hölscher C. Receptors for the incretin glucagon-like peptide-1 are expressed on neurons in the central nervous system. Neuroreport (2009) 20:1161–6. doi: 10.1097/WNR.0b013e32832fbf14
67. Cheng D, Yang S, Zhao X, Wang G. The role of glucagon-like peptide-1 receptor agonists (GLP-1 RA) in diabetes-related neurodegenerative diseases. Drug Des Devel Ther (2022) 16:665–84. doi: 10.2147/DDDT.S348055
68. Hamilton A, Patterson S, Porter D, Gault VA, Holscher C. Novel GLP-1 mimetics developed to treat type 2 diabetes promote progenitor cell proliferation in the brain. J Neurosci Res (2011) 89:481–9. doi: 10.1002/jnr.22565
69. Alvarez E, Martínez MD, Roncero I, Chowen JA, García-Cuartero B, Gispert JD, et al. The expression of GLP-1 receptor mRNA and protein allows the effect of GLP-1 on glucose metabolism in the human hypothalamus and brainstem. J Neurochem (2005) 92:798–806. doi: 10.1111/j.1471-4159.2004.02914.x
70. Cork SC, Richards JE, Holt MK, Gribble FM, Reimann F, Trapp S. Distribution and characterisation of glucagon-like peptide-1 receptor expressing cells in the mouse brain. Mol Metab (2015) 4:718–31. doi: 10.1016/j.molmet.2015.07.008
71. Marques C, Mega C, Gonçalves A, Rodrigues-Santos P, Teixeira-Lemos E, Teixeira F, et al. Sitagliptin prevents inflammation and apoptotic cell death in the kidney of type 2 diabetic animals. Mediators Inflamm (2014) 2014:538737. doi: 10.1155/2014/538737
72. Iwai T, Ito S, Tanimitsu K, Udagawa S, Oka J-I. Glucagon-like peptide-1 inhibits LPS-induced IL-1beta production in cultured rat astrocytes. Neurosci Res (2006) 55:352–60. doi: 10.1016/j.neures.2006.04.008
73. Bendotti G, Montefusco L, Lunati ME, Usuelli V, Pastore I, Lazzaroni E, et al. The anti-inflammatory and immunological properties of GLP-1 receptor agonists. Pharmacol Res (2022) 182:106320. doi: 10.1016/j.phrs.2022.106320
74. Amor S, Puentes F, Baker D, van der Valk P. Inflammation in neurodegenerative diseases. Immunology (2010) 129:154–69. doi: 10.1111/j.1365-2567.2009.03225.x
75. Süβ P, Lana AJ. Schlachetzki JCM: chronic peripheral inflammation: a possible contributor to neurodegenerative diseases. Neural Regener Res (2021) 16:1711–4. doi: 10.4103/1673-5374.306060
76. Hölscher C. Central effects of GLP-1: new opportunities for treatments of neurodegenerative diseases. J Endocrinol (2014) 221:T31–41. doi: 10.1530/JOE-13-0221
77. Block ML, Hong J-S. Microglia and inflammation-mediated neurodegeneration: multiple triggers with a common mechanism. Prog Neurobiol (2005) 76:77–98. doi: 10.1016/j.pneurobio.2005.06.004
78. Mrak RE, Griffin WST. Glia and their cytokines in progression of neurodegeneration. Neurobiol Aging (2005) 26:349–54. doi: 10.1016/j.neurobiolaging.2004.05.010
79. Dorsey ER, Sherer T, Okun MS, Bloem BR. The emerging evidence of the Parkinson pandemic. J Parkinsons Dis (2018) 8:S3–8. doi: 10.3233/JPD-181474
80. Pringsheim T, Jette N, Frolkis A, Steeves TDL. The prevalence of parkinson’s disease: a systematic review and meta-analysis. Mov Disord (2014) 29:1583–90. doi: 10.1002/mds.25945
81. Kouli A, Torsney KM, Kuan W-L. Parkinson’s disease: etiology, neuropathology, and pathogenesis. In: Stoker TB, Greenland JC, editors. Parkinson’s Disease: Pathogenesis and Clinical Aspects. Brisbane (AU): Codon Publications (2018). Chapter 1. Available at: https://www.ncbi.nlm.nih.gov/books/NBK536722/
82. Keshavarzian A, Green SJ, Engen PA, Voigt RM, Naqib A, Forsyth CB, et al. Colonic bacterial composition in parkinson’s disease. Mov Disord (2015) 30:1351–60. doi: 10.1002/mds.26307
83. MacMahon Copas AN, McComish SF, Fletcher JM, Caldwell MA. The pathogenesis of parkinson’s disease: a complex interplay between astrocytes, microglia, and T lymphocytes? Front Neurol (2021) 12:666737. doi: 10.3389/fneur.2021.666737
84. Marras C, Beck JC, Bower JH, Roberts E, Ritz B, Ross GW, et al. Prevalence of parkinson’s disease across north America. NPJ Parkinsons Dis (2018) 4:21. doi: 10.1038/s41531-018-0058-0
85. Yang W, Hamilton JL, Kopil C, Beck JC, Tanner CM, Albin RL, et al. Current and projected future economic burden of parkinson’s disease in the U.S.. NPJ Parkinsons Dis (2020) 6:15. doi: 10.1038/s41531-020-0117-1
86. Manfready RA, Engen PA, Verhagen Metman L, Sanzo G, Goetz CG, Hall DA, et al. Attenuated postprandial GLP-1 response in parkinson’s disease. Front Neurosci (2021) 15:660942. doi: 10.3389/fnins.2021.660942
88. Gómez-Benito M, Granado N, García-Sanz P, Michel A, Dumoulin M, Moratalla R. Modeling parkinson’s disease with the alpha-synuclein protein. Front Pharmacol (2020) 11:356. doi: 10.3389/fphar.2020.00356
89. Maiti P, Manna J, Dunbar GL. Current understanding of the molecular mechanisms in parkinson’s disease: targets for potential treatments. Transl Neurodegener (2017) 6:28. doi: 10.1186/s40035-017-0099-z
90. Kalia LV, Lang AE. Parkinson’s disease. Lancet (2015) 386:896–912. doi: 10.1016/S0140-6736(14)61393-3
91. Kim WS, Kågedal K, Halliday GM. Alpha-synuclein biology in lewy body diseases. Alzheimers Res Ther (2014) 6:73. doi: 10.1186/s13195-014-0073-2
92. Dias V, Junn E, Mouradian MM. The role of oxidative stress in parkinson’s disease. J Parkinsons Dis (2013) 3:461–91. doi: 10.3233/JPD-130230
93. Meiser J, Weindl D, Hiller K. Complexity of dopamine metabolism. Cell Commun Signal (2013) 11:34. doi: 10.1186/1478-811X-11-34
94. Bartels AL, Willemsen ATM, Doorduin J, de Vries EFJ, Dierckx RA, Leenders KL. [11C]-PK11195 PET: quantification of neuroinflammation and a monitor of anti-inflammatory treatment in parkinson’s disease? Parkinsonism Relat Disord (2010) 16:57–9. doi: 10.1016/j.parkreldis.2009.05.005
95. Fan Z, Aman Y, Ahmed I, Chetelat G, Landeau B, Ray Chaudhuri K, et al. Influence of microglial activation on neuronal function in alzheimer’s and parkinson’s disease dementia. Alzheimers Dement (2015) 11:608–621.e7. doi: 10.1016/j.jalz.2014.06.016
96. Cao B, Zhang Y, Chen J, Wu P, Dong Y, Wang Y. Neuroprotective effects of liraglutide against inflammation through the AMPK/NF-κB pathway in a mouse model of parkinson’s disease. Metab Brain Dis (2022) 37:451–62. doi: 10.1007/s11011-021-00879-1
97. Zhu M, Gong D. A mouse model of 1-Methyl-4-Phenyl-1,2,3,6-Tetrahydropyridine (MPTP)-induced Parkinson disease shows that 2-aminoquinoline targets JNK phosphorylation. Med Sci Monit (2020) 26:e920989. doi: 10.12659/MSM.920989
98. De Iuliis A, Montinaro E, Fatati G, Plebani M, Colosimo C. Diabetes mellitus and parkinson’s disease: dangerous liaisons between insulin and dopamine. Neural Regener Res (2022) 17:523–33. doi: 10.4103/1673-5374.320965
99. Sarath Babu N, Murthy CLN, Kakara S, Sharma R, Brahmendra Swamy CV, Idris MM. 1-Methyl-4-phenyl-1,2,3,6-tetrahydropyridine induced parkinson’s disease in zebrafish. Proteomics (2016) 16:1407–20. doi: 10.1002/pmic.201500291
100. Liu L, Arun A, Ellis L, Peritore C, Donmez G. SIRT2 enhances 1-methyl-4-phenyl-1,2,3,6-tetrahydropyridine (MPTP)-induced nigrostriatal damage via apoptotic pathway. Front Aging Neurosci (2014) 6:184. doi: 10.3389/fnagi.2014.00184
101. Ferrari F, Moretti A, Villa RF. Incretin-based drugs as potential therapy for neurodegenerative diseases: current status and perspectives. Pharmacol Ther (2022) 239:108277. doi: 10.1016/j.pharmthera.2022.108277
102. Wang L, Zhai Y-Q, Xu L-L, Qiao C, Sun XL, Ding JH, et al. Metabolic inflammation exacerbates dopaminergic neuronal degeneration in response to acute MPTP challenge in type 2 diabetes mice. Exp Neurol (2014) 251:22–9. doi: 10.1016/j.expneurol.2013.11.001
103. Xu K, Xu Y, Brown-Jermyn D, Chen J-F, Ascherio A, Dluzen DE, et al. Estrogen prevents neuroprotection by caffeine in the mouse 1-methyl-4-phenyl-1,2,3,6-tetrahydropyridine model of parkinson’s disease. J Neurosci (2006) 26:535–41. doi: 10.1523/JNEUROSCI.3008-05.2006
104. Majeski JA, Upshur JK. Asplenia syndrome. a study of congenital anomalies in 16 cases. JAMA (1978) 240:1508–10. doi: 10.1001/jama.240.14.1508
105. Yoon G, Kim Y-K, Song J. Glucagon-like peptide-1 suppresses neuroinflammation and improves neural structure. Pharmacol Res (2020) 152:104615. doi: 10.1016/j.phrs.2019.104615
106. Lee C-H, Jeon SJ, Cho KS, Moon E, Sapkota A, Jun HS, et al. Activation of glucagon-like peptide-1 receptor promotes neuroprotection in experimental autoimmune encephalomyelitis by reducing neuroinflammatory responses. Mol Neurobiol (2018) 55:3007–20. doi: 10.1007/s12035-017-0550-2
107. Diz-Chaves Y, Mastoor Z, Spuch C, González-Matías LC, Mallo F. Anti-inflammatory effects of GLP-1 receptor activation in the brain in neurodegenerative diseases. Int J Mol Sci (2022) 23:9583. doi: 10.3390/ijms23179583
108. Li Y, Perry T, Kindy MS, Harvey BK, Tweedie D, Holloway HW, et al. GLP-1 receptor stimulation preserves primary cortical and dopaminergic neurons in cellular and rodent models of stroke and parkinsonism. Proc Natl Acad Sci U S A (2009) 106:1285–90. doi: 10.1073/pnas.0806720106
109. Harkavyi A, Rampersaud N, Whitton PS. Neuroprotection by exendin-4 is GLP-1 receptor specific but DA D3 receptor dependent, causing altered BrdU incorporation in subventricular zone and substantia nigra. J Neurodegener Dis (2013) 2013:407152. doi: 10.1155/2013/407152
110. Holtzman DM, Morris JC, Goate AM. Alzheimer’s disease: the challenge of the second century. Sci Transl Med (2011) 3:77sr1. doi: 10.1126/scitranslmed.3002369
111. Grieco M, Giorgi A, Gentile MC, d’Erme M, Morano S, Maras B, et al. Glucagon-like peptide-1: a focus on neurodegenerative diseases. Front Neurosci (2019) 13:1112. doi: 10.3389/fnins.2019.01112
112. 2022 alzheimer’s disease facts and figures. Alzheimers Dement (2022) 18:700–89. doi: 10.1002/alz.12638
113. Qiu C, Kivipelto M, von Strauss E. Epidemiology of alzheimer’s disease: occurrence, determinants, and strategies toward intervention. Dialogues Clin Neurosci (2009) 11:111–28. doi: 10.31887/DCNS.2009.11.2/cqiu
114. Plassman BL, Langa KM, Fisher GG, Heeringa SG, Weir DR, Ofstedal MB, et al. Prevalence of dementia in the united states: the aging, demographics, and memory study. Neuroepidemiology (2007) 29:125–32. doi: 10.1159/000109998
115. Fratiglioni L, Launer LJ, Andersen K, Breteler MM, Copeland JR, Dartigues JF, et al. Incidence of dementia and major subtypes in Europe: a collaborative study of population-based cohorts. neurologic diseases in the elderly research group. Neurology (2000) 54:S10–15.
116. Rajan KB, Weuve J, Barnes LL, McAninch EA, Wilson RS, Evans DA. Population estimate of people with clinical alzheimer’s disease and mild cognitive impairment in the united states (2020-2060). Alzheimers Dement (2021) 17:1966–75. doi: 10.1002/alz.12362
117. Meraz-Ríos MA, Toral-Rios D, Franco-Bocanegra D, Villeda-Hernández J, Campos-Peña V. Inflammatory process in alzheimer’s disease. Front Integr Neurosci (2013) 7:59. doi: 10.3389/fnint.2013.00059
118. Goldgaber D, Harris HW, Hla T, Maciag T, Donnelly RJ, Jacobsen JS, et al. Interleukin 1 regulates synthesis of amyloid beta-protein precursor mRNA in human endothelial cells. Proc Natl Acad Sci U S A (1989) 86:7606–10. doi: 10.1073/pnas.86.19.7606
119. Li Q-X, Gao H, Guo Y-X, Wang BY, Hua RX, Gao L, et al. GLP-1 and underlying beneficial actions in alzheimer’s disease, hypertension, and NASH. Front Endocrinol (Lausanne) (2021) 12:721198. doi: 10.3389/fendo.2021.721198
120. Bliss TV, Collingridge GL. A synaptic model of memory: long-term potentiation in the hippocampus. Nature (1993) 361:31–9. doi: 10.1038/361031a0
121. Rebosio C, Balbi M, Passalacqua M, Ricciarelli R, Fedele E. Presynaptic GLP-1 receptors enhance the depolarization-evoked release of glutamate and GABA in the mouse cortex and hippocampus. Biofactors (2018) 44:148–57. doi: 10.1002/biof.1406
122. Wiera G, Nowak D, van Hove I, Dziegiel P, Moons L, Mozrzymas JW. Mechanisms of NMDA receptor- and voltage-gated l-type calcium channel-dependent hippocampal LTP critically rely on proteolysis that is mediated by distinct metalloproteinases. J Neurosci (2017) 37:1240–56. doi: 10.1523/JNEUROSCI.2170-16.2016
123. Huang J, Liu Y, Cheng L, Li J, Zhang T, Zhao G, et al. Glucagon-like peptide-1 cleavage product GLP-1(9-36) reduces neuroinflammation from stroke via the activation of insulin-like growth factor 1 receptor in astrocytes. Eur J Pharmacol (2020) 887:173581. doi: 10.1016/j.ejphar.2020.173581
124. Barreto-Vianna ARC, Aguila MB, Mandarim-de-Lacerda CA. Beneficial effects of liraglutide (GLP1 analog) in the hippocampal inflammation. Metab Brain Dis (2017) 32:1735–45. doi: 10.1007/s11011-017-0059-4
125. Diz-Chaves Y, Toba L, Fandiño J, González-Matías LC, Garcia-Segura LM, Mallo F. The GLP-1 analog, liraglutide prevents the increase of proinflammatory mediators in the hippocampus of male rat pups submitted to maternal perinatal food restriction. J Neuroinflamm (2018) 15:337. doi: 10.1186/s12974-018-1370-7
126. Elder GA, Gama Sosa MA, De Gasperi R. Transgenic mouse models of alzheimer’s disease. Mt Sinai J Med (2010) 77:69–81. doi: 10.1002/msj.20159
127. Kitazawa M, Medeiros R, Laferla FM. Transgenic mouse models of Alzheimer disease: developing a better model as a tool for therapeutic interventions. Curr Pharm Des (2012) 18:1131–47. doi: 10.2174/138161212799315786
128. Fukuda M. The role of GIP receptor in the CNS for the pathogenesis of obesity. Diabetes (2021) 70:1929–37. doi: 10.2337/dbi21-0001
129. Spielman LJ, Gibson DL, Klegeris A. Incretin hormones regulate microglia oxidative stress, survival and expression of trophic factors. Eur J Cell Biol (2017) 96:240–53. doi: 10.1016/j.ejcb.2017.03.004
130. Zhang ZQ, Hölscher C. GIP has neuroprotective effects in Alzheimer and parkinson’s disease models. Peptides (2020) 125:170184. doi: 10.1016/j.peptides.2019.170184
131. Parthsarathy V, Hölscher C. The type 2 diabetes drug liraglutide reduces chronic inflammation induced by irradiation in the mouse brain. Eur J Pharmacol (2013) 700:42–50. doi: 10.1016/j.ejphar.2012.12.012
132. Kim S, Jeong J, Jung H-S, Kim B, Kim YE, Lim DS, et al. Anti-inflammatory effect of glucagon like peptide-1 receptor agonist, exendin-4, through modulation of IB1/JIP1 expression and JNK signaling in stroke. Exp Neurobiol (2017) 26:227–39. doi: 10.5607/en.2017.26.4.227
133. Béjot Y, Giroud M. Stroke in diabetic patients. Diabetes Metab (2010) 36 Suppl 3:S84–87. doi: 10.1016/S1262-3636(10)70472-9
134. Marlet IR, Ölmestig JNE, Vilsbøll T, Rungby J, Kruuse C. Neuroprotective mechanisms of glucagon-like peptide-1-based therapies in ischaemic stroke: a systematic review based on pre-clinical studies. Basic Clin Pharmacol Toxicol (2018) 122:559–69. doi: 10.1111/bcpt.12974
135. Teramoto S, Miyamoto N, Yatomi K, Tanaka Y, Oishi H, Arai H, et al. Exendin-4, a glucagon-like peptide-1 receptor agonist, provides neuroprotection in mice transient focal cerebral ischemia. J Cereb Blood Flow Metab (2011) 31:1696–705. doi: 10.1038/jcbfm.2011.51
136. Padmasekar M, Lingwal N, Samikannu B, Chen C, Sauer H, Linn T. Exendin-4 protects hypoxic islets from oxidative stress and improves islet transplantation outcome. Endocrinology (2013) 154:1424–33. doi: 10.1210/en.2012-1983
137. Candelario-Jalil E, González-Falcón A, García-Cabrera M, Alvarez D, Al-Dalain S, Martínez G, et al. Assessment of the relative contribution of COX-1 and COX-2 isoforms to ischemia-induced oxidative damage and neurodegeneration following transient global cerebral ischemia. J Neurochem (2003) 86:545–55. doi: 10.1046/j.1471-4159.2003.01812.x
138. Perry T, Greig NH. The glucagon-like peptides: a double-edged therapeutic sword? Trends Pharmacol Sci (2003) 24:377–83. doi: 10.1016/S0165-6147(03)00160-3
139. Timmers L, Henriques JPS, de Kleijn DPV, Devries JH, Kemperman H, Steendijk P, et al. Exenatide reduces infarct size and improves cardiac function in a porcine model of ischemia and reperfusion injury. J Am Coll Cardiol (2009) 53:501–10. doi: 10.1016/j.jacc.2008.10.033
140. Hui H, Nourparvar A, Zhao X, Perfetti R. Glucagon-like peptide-1 inhibits apoptosis of insulin-secreting cells via a cyclic 5’-adenosine monophosphate-dependent protein kinase a- and a phosphatidylinositol 3-kinase-dependent pathway. Endocrinology (2003) 144:1444–55. doi: 10.1210/en.2002-220897
141. Yang X, Ma X, Don O, Song Y, Chen X, Liu J, et al. Mesenchymal stem cells combined with liraglutide relieve acute lung injury through apoptotic signaling restrained by PKA/β-catenin. Stem Cell Res Ther (2020) 11:182. doi: 10.1186/s13287-020-01689-5
142. Wu AY, Peebles RS. The GLP-1 receptor in airway inflammation in asthma: a promising novel target? Expert Rev Clin Immunol (2021) 17:1053–7. doi: 10.1080/1744666X.2021.1971973
143. Wander PL, Lowy E, Beste LA, Tulloch-Palomino L, Korpak A, et al. Prior glucose-lowering medication use and 30-day outcomes among 64,892 veterans with diabetes and COVID-19. Diabetes Care (2021) 44:2708–13. doi: 10.2337/dc21-1351
144. Rogliani P, Calzetta L, Capuani B, Facciolo F, Cazzola M, Lauro D, et al. Glucagon-like peptide 1 receptor: a novel pharmacological target for treating human bronchial hyperresponsiveness. Am J Respir Cell Mol Biol (2016) 55:804–14. doi: 10.1165/rcmb.2015-0311OC
145. Toki S, Newcomb DC, Printz RL, Cahill KN, Boyd KL, Niswender KD, et al. Glucagon-like peptide-1 receptor agonist inhibits aeroallergen-induced activation of ILC2 and neutrophilic airway inflammation in obese mice. Allergy (2021) 76:3433–45. doi: 10.1111/all.14879
146. Skurikhin EG, Pershina OV, Pakhomova AV, Pan ES, Krupin VA, Ermakova NN, et al. Endothelial progenitor cells as pathogenetic and diagnostic factors, and potential targets for GLP-1 in combination with metabolic syndrome and chronic obstructive pulmonary disease. Int J Mol Sci (2019) 20:1105. doi: 10.3390/ijms20051105
147. López-Cano C, Ciudin A, Sánchez E, Tinahones FJ, Barbé F, Dalmases M, et al. Liraglutide improves forced vital capacity in individuals with type 2 diabetes: data from the randomized crossover LIRALUNG study. Diabetes (2022) 71:315–20. doi: 10.2337/db21-0688
148. Nguyen D-V, Linderholm A, Haczku A, Kenyon N. Glucagon-like peptide 1: a potential anti-inflammatory pathway in obesity-related asthma. Pharmacol Ther (2017) 180:139–43. doi: 10.1016/j.pharmthera.2017.06.012
149. Vara E, Arias-Díaz J, Garcia C, Balibrea JL, Blázquez E. Glucagon-like Peptide-1(7–36) amide stimulates surfactant secretion in human type II pneumocytes. Am J Respir Crit Care Med (2001) 163:840–6. doi: 10.1164/ajrccm.163.4.9912132
150. Benito E, Blazquez E, Bosch MA. Glucagon-like Peptide-1-(7–36)Amide increases pulmonary surfactant secretion through a cyclic adenosine 3 3′,5′--Monophosphate-Dependent protein kinase mechanism in rat type II pneumocytes. Endocrinology (1998) 139:6. doi: 10.1210/endo.139.5.5998
151. Zhu T, Li C, Zhang X, Ye C, Tang S, Zhang W, et al. GLP-1 analogue liraglutide enhances SP-a expression in LPS-induced acute lung injury through the TTF-1 signaling pathway. Mediators Inflamm (2018) 2018:e3601454. doi: 10.1155/2018/3601454
152. Zhou F, Zhang Y, Chen J, Hu X, Xu Y. Liraglutide attenuates lipopolysaccharide-induced acute lung injury in mice. Eur J Pharmacol (2016) 791:735–40. doi: 10.1016/j.ejphar.2016.10.016
153. Bai Y, Lian P, Li J, Zhang Z, Qiao J. The active GLP-1 analogue liraglutide alleviates H9N2 influenza virus-induced acute lung injury in mice. Microbial Pathogene (2021) 150:104645. doi: 10.1016/j.micpath.2020.104645
154. Choi W, Choe S, Lin J, Borchers MT, Kosmider B, Vassallo R, et al. Exendin-4 restores airway mucus homeostasis through the GLP1R-PKA-PPARγ-FOXA2-phosphatase signaling. Mucosal Immunol (2020) 13:637–51. doi: 10.1038/s41385-020-0262-1
155. Oztay F, Sancar-Bas S, Gezginci-Oktayoglu S, Ercin M, Bolkent S. Exendin-4 partly ameliorates - hyperglycemia-mediated tissue damage in lungs of streptozotocin-induced diabetic mice. Peptides (2018) 99:99–107. doi: 10.1016/j.peptides.2017.12.007
156. Gou S, Zhu T, Wang W, Xiao M, Wang X, Chen Z. Glucagon like peptide-1 attenuates bleomycin-induced pulmonary fibrosis, involving the inactivation of NF-κB in mice. Int Immunopharmacol (2014) 22:498–504. doi: 10.1016/j.intimp.2014.07.010
157. Richter G, Feddersen O, Wagner U, Barth P, Göke R, Göke B. GLP-1 stimulates secretion of macromolecules from airways and relaxes pulmonary artery. Am J Physiol (1993) 265:L374–381. doi: 10.1152/ajplung.1993.265.4.L374
158. Golpon HA, Puechner A, Welte T, Wichert PV, Feddersen CO. Vasorelaxant effect of glucagon-like peptide-ž7-36/amide and amylin on the pulmonary circulation of the rat. Regul Pept (2001) 6:81–6. doi: 10.1016/S0167-0115(01)00300-7
159. Fandiño J, Vaz AA, Toba L, Romaní-Pérez M, González-Matías L, Mallo F, et al. Liraglutide enhances the activity of the ACE-2/Ang(1–7)/Mas receptor pathway in lungs of Male pups from food-restricted mothers and prevents the reduction of SP-a. Int J Endocrinol (2018) 2018:e6920620. doi: 10.1155/2018/6920620
160. Charlton A, Garzarella J, Jandeleit-Dahm KAM, Jha JC. Oxidative stress and inflammation in renal and cardiovascular complications of diabetes. Biol (Basel) (2020) 10:18. doi: 10.3390/biology10010018
161. Jha JC, Banal C, Chow BSM, Cooper ME, Jandeleit-Dahm K. Diabetes and kidney disease: role of oxidative stress. Antioxid Redox Signal (2016) 25:657–84. doi: 10.1089/ars.2016.6664
162. Fujita H, Morii T, Fujishima H, Sato T, Shimizu T, Hosoba M, et al. The protective roles of GLP-1R signaling in diabetic nephropathy: possible mechanism and therapeutic potential. Kidney Int (2014) 85:579–89. doi: 10.1038/ki.2013.427
163. Greco EV, Russo G, Giandalia A, Viazzi F, Pontremoli R, De Cosmo S. GLP-1 receptor agonists and kidney protection. Med (Kaunas) (2019) 55:233. doi: 10.3390/medicina55060233
164. Sourris KC, Yao H, Jerums G, Cooper ME, Ekinci EI, Coughlan MT. Can targeting the incretin pathway dampen RAGE-mediated events in diabetic nephropathy? Curr Drug Targets (2016) 17:1252–64. doi: 10.2174/1389450116666150722141418
165. Jha JC, Ho F, Dan C, Jandeleit-Dahm K. A causal link between oxidative stress and inflammation in cardiovascular and renal complications of diabetes. Clin Sci (Lond) (2018) 132:1811–36. doi: 10.1042/CS20171459
166. Tessaro FHG, Ayala TS, Martins JO. Lipid mediators are critical in resolving inflammation: a review of the emerging roles of eicosanoids in diabetes mellitus. BioMed Res Int (2015) 2015:568408. doi: 10.1155/2015/568408
167. Kodera R, Shikata K, Kataoka HU, Takatsuka T, Miyamoto S, Sasaki M, et al. Glucagon-like peptide-1 receptor agonist ameliorates renal injury through its anti-inflammatory action without lowering blood glucose level in a rat model of type 1 diabetes. Diabetologia (2011) 54:965–78. doi: 10.1007/s00125-010-2028-x
168. Liljedahl L, Pedersen MH, McGuire JN, James P. The impact of the glucagon-like peptide 1 receptor agonist liraglutide on the streptozotocin-induced diabetic mouse kidney proteome. Physiol Rep (2019) 7:e13994. doi: 10.14814/phy2.13994
169. Choi JH, Kim SJ, Kwon SK, Kim H-Y, Jeon H. Renal tubular glucagon-like peptide-1 receptor expression is increased in early sepsis but reduced in chronic kidney disease and sepsis-induced kidney injury. Int J Mol Sci (2019) 20:E6024. doi: 10.3390/ijms20236024
170. Yin W, Xu S, Wang Z, Liu H, Peng L, Fang Q, et al. Recombinant human GLP-1(rhGLP-1) alleviating renal tubulointestitial injury in diabetic STZ-induced rats. Biochem Biophys Res Commun (2018) 495:793–800. doi: 10.1016/j.bbrc.2017.11.076
171. Xu L, Ren Y. [Sitagliptin inhibits cell apoptosis and inflammation of renal tissues in diabetic nephropathy model rats]. Xi Bao Yu Fen Zi Mian Yi Xue Za Zhi (2019) 35:217–22.
172. Xue S-N, Lei J, Lin D-Z, Yang C, Yan L. Changes in biological behaviors of rat dermal fibroblasts induced by high expression of MMP9. World J Emerg Med (2014) 5:139–43. doi: 10.5847/wjem.j.issn.1920-8642.2014.02.011
173. Wolak M, Drobnik J, Bojanowska E. Exendin-4 differentially modulates essential functions of human dermal fibroblasts under normoglycemic and hyperglycemic conditions. J Physiol Pharmacol (2021) 72(3):461–7. doi: 10.26402/jpp.2021.3.14
174. Murphy G. Tissue inhibitors of metalloproteinases. Genome Biol (2011) 12:233. doi: 10.1186/gb-2011-12-11-233
175. Reiss MJ, Han Y-P, Garcia E, Goldberg M, Yu H, Garner WL. Matrix metalloproteinase-9 delays wound healing in a murine wound model. Surgery (2010) 147:295–302. doi: 10.1016/j.surg.2009.10.016
176. Wysocki AB, Staiano-Coico L, Grinnell F. Wound fluid from chronic leg ulcers contains elevated levels of metalloproteinases MMP-2 and MMP-9. J Invest Dermatol (1993) 101:64–8. doi: 10.1111/1523-1747.ep12359590
177. Lao G, Ren M, Wang X, Zhang J, Huang Y, Liu D, et al. Human tissue inhibitor of metalloproteinases-1 improved wound healing in diabetes through its anti-apoptotic effect. Exp Dermatol (2019) 28:528–35. doi: 10.1111/exd.13442
178. Li Z, Guo S, Yao F, Zhang Y, Li T. Increased ratio of serum matrix metalloproteinase-9 against TIMP-1 predicts poor wound healing in diabetic foot ulcers. J Diabetes its Complications (2013) 27:380–2. doi: 10.1016/j.jdiacomp.2012.12.007
179. Zayani Y, Allal-Elasmi M, Jacob MP, Zidi W, Ftouhi B, Feki M, et al. Abnormal circulating levels of matrix metalloproteinases and their inhibitors in diabetes mellitus. Clin Lab (2013) 59:7–8. doi: 10.7754/Clin.Lab.2011.110904
180. Mouës CM, Van Toorenenbergen AW, Heule F, Hop WC, Hovius SER. The role of topical negative pressure in wound repair: expression of biochemical markers in wound fluid during wound healing. Wound Repair Regeneration (2008) 16:488–94. doi: 10.1111/j.1524-475X.2008.00395.x
181. Dubský M, Jirkovská A, Bem R, Fejfarová V, Skibová J, Schaper NC, et al. Risk factors for recurrence of diabetic foot ulcers: prospective follow-up analysis in the eurodiale subgroup. Int Wound J (2013) 10:555–61. doi: 10.1111/j.1742-481X.2012.01022.x
182. Wolak M, Staszewska T, Juszczak M, Gałdyszyńska M, Bojanowska E. Anti-inflammatory and pro-healing impacts of exendin-4 treatment in zucker diabetic rats: effects on skin wound fibroblasts. Eur J Pharmacol (2019) 842:262–9. doi: 10.1016/j.ejphar.2018.10.053
183. Tecilazich F, Dinh T, Pradhan-Nabzdyk L, Leal E, Tellechea A, Kafanas A, et al. Role of endothelial progenitor cells and inflammatory cytokines in healing of diabetic foot ulcers. PloS One (2013) 8:e83314. doi: 10.1371/journal.pone.0083314
184. Al-Badri MR, Azar ST. Effect of glucagon-like peptide-1 receptor agonists in patients with psoriasis. Ther Adv Endocrinol Metab (2014) 5:34–8. doi: 10.1177/2042018814543483
185. Farshchian M, Ansar A, Sobhan M, Hoseinpoor V. C-reactive protein serum level in patients with psoriasis before and after treatment with narrow-band ultraviolet b. Bras Dermatol (2016) 91:580–3. doi: 10.1590/abd1806-4841.20164655
186. Faurschou A, Pedersen J, Gyldenløve M, Poulsen SS, Holst JJ, Thyssen JP, et al. Increased expression of glucagon-like peptide-1 receptors in psoriasis plaques. Exp Dermatol (2013) 22:150–2. doi: 10.1111/exd.12081
187. Hogan AE, Tobin AM, Ahern T, Corrigan MA, Gaoatswe G, Jackson R, et al. Glucagon-like peptide-1 (GLP-1) and the regulation of human invariant natural killer T cells: lessons from obesity, diabetes and psoriasis. Diabetologia (2011) 54:2745. doi: 10.1007/s00125-011-2232-3
188. Wellen KE, Hotamisligil GS. Inflammation, stress, and diabetes. J Clin Invest (2005) 115:1111–9. doi: 10.1172/JCI25102
189. Armstrong AW, Harskamp CT, Armstrong EJ. The association between psoriasis and obesity: a systematic review and meta-analysis of observational studies. Nutr Diabetes (2012) 2:e54–4. doi: 10.1038/nutd.2012.26
190. Xu X, Lin L, Chen P, Yu Y, Chen S, Chen X, et al. Treatment with liraglutide, a glucagon-like peptide-1 analogue, improves effectively the skin lesions of psoriasis patients with type 2 diabetes: a prospective cohort study. Diabetes Res Clin Prac (2019) 150:167–73. doi: 10.1016/j.diabres.2019.03.002
191. Lin L, Xu X, Yu Y, Ye H, He X, Chen S, et al. Glucagon-like peptide-1 receptor agonist liraglutide therapy for psoriasis patients with type 2 diabetes: a randomized-controlled trial. J Dermatol Treat (2022) 33:1428–34. doi: 10.1080/09546634.2020.1826392
192. Costanzo G, Curatolo S, Busà B, Belfiore A, Gullo D. Two birds one stone: semaglutide is highly effective against severe psoriasis in a type 2 diabetic patient. Endocrinol Diabetes Metab Case Rep (2021) 2021:21–0007. doi: 10.1530/EDM-21-0007
193. Savchenko LG, Digtiar NI, Selikhova LG, Kaidasheva EI, Shlykova OA, Vesnina LE, et al. Liraglutide exerts an anti-inflammatory action in obese patients with type 2 diabetes. Rom J Intern Med (2019) 57:233–40. doi: 10.2478/rjim-2019-0003
194. Zobel EH, Ripa RS, von Scholten BJ, Rotbain Curovic V, Kjaer A, Hansen TW, et al. Effect of liraglutide on expression of inflammatory genes in type 2 diabetes. Sci Rep (2021) 11:18522. doi: 10.1038/s41598-021-97967-0
195. Inaishi J, Saisho Y. Exenatide once weekly for management of type 2 diabetes: a review. CPAA (2022) 14:19–26. doi: 10.2147/CPAA.S288846
196. Frías JP, Davies MJ, Rosenstock J, Pérez Manghi FC, Fernández Landó L, Bergman BK, et al. Tirzepatide versus semaglutide once weekly in patients with type 2 diabetes. N Engl J Med (2021) 385:503–15. doi: 10.1056/NEJMoa2107519
197. Abdalla MA, Deshmukh H, Atkin S, Sathyapalan T. A review of therapeutic options for managing the metabolic aspects of polycystic ovary syndrome. Ther Adv Endocrinol Metab (2020) 11:2042018820938305. doi: 10.1177/2042018820938305
198. Torres Fernandez ED, Huffman AM, Syed M, Romero DG, Yanes Cardozo LL. Effect of GLP-1 receptor agonists in the cardiometabolic complications in a rat model of postmenopausal PCOS. Endocrinology (2019) 160:2787–99. doi: 10.1210/en.2019-00450
199. Frøssing S, Nylander M, Chabanova E, Frystyk J, Holst JJ, Kistorp C, et al. Effect of liraglutide on ectopic fat in polycystic ovary syndrome: a randomized clinical trial. Diabetes Obes Metab (2018) 20:215–8. doi: 10.1111/dom.13053
200. Elkind-Hirsch KE, Chappell N, Seidemann E, Storment J, Bellanger D. Exenatide, dapagliflozin, or Phentermine/Topiramate differentially affect metabolic profiles in polycystic ovary syndrome. J Clin Endocrinol Metab (2021) 106:3019–33. doi: 10.1210/clinem/dgab408
201. Ma R-L, Deng Y, Wang Y-F, Zhu S-Y, Ding X-S, Sun A-J, et al. Short-term combined treatment with exenatide and metformin for overweight/obese women with polycystic ovary syndrome. (Engl) (2021) 134:2882–9. doi: 10.1097/CM9.0000000000001712
202. Han Y, Li Y, He B. GLP-1 receptor agonists versus metformin in PCOS: a systematic review and meta-analysis. Reprod BioMed Online (2019) 39:332–42. doi: 10.1016/j.rbmo.2019.04.017
203. Xing C, Li C, He B. Insulin sensitizers for improving the endocrine and metabolic profile in overweight women with PCOS. J Clin Endocrinol Metab (2020) 105:2950–63. doi: 10.1210/clinem/dgaa337
204. Grill HJ. A role for GLP-1 in treating hyperphagia and obesity. Endocrinology (2020) 161:bqaa093. doi: 10.1210/endocr/bqaa093
205. Fryar CD, Carroll MD, Ogden CL. Prevalence of overweight, obesity, and extreme obesity among adults: united states, trends 1960–1962 through 2009–2010 (2019). Available at: https://www.cdc.gov/nchs/data/hestat/obesity_adult_09_10/obesity_adult_09_10.htm (Accessed January 18, 2023).
206. Hales CM, Carroll MD, Fryar CD, Ogden CL. Prevalence of obesity and severe obesity among adults: united states, 2017–2018 (2020). Available at: https://www.cdc.gov/nchs/products/databriefs/db360.htm (Accessed January 18, 2023).
207. Sanders DM. Efficacy of GLP-1 agonists for weight loss in adults without diabetes . Available at: https://www.clinicaladvisor.com/home/topics/obesity-information-center/glp-1-agonists-weight-loss-adults-without-diabetes-liraglutide-semaglutide/ (Accessed January 18, 2023).
208. Michałowska J, Miller-Kasprzak E, Bogdański P. Incretin hormones in obesity and related cardiometabolic disorders: the clinical perspective. Nutrients (2021) 13:351. doi: 10.3390/nu13020351
209. Mehta A, Marso SP, Neeland IJ. Liraglutide for weight management: a critical review of the evidence. Obes Sci Pract (2017) 3:3–14. doi: 10.1002/osp4.84
210. Ceriello A, Novials A, Ortega E, Canivell S, La Sala L, Pujadas G, et al. Glucagon-like peptide 1 reduces endothelial dysfunction, inflammation, and oxidative stress induced by both hyperglycemia and hypoglycemia in type 1 diabetes. Diabetes Care (2013) 36:2346–50. doi: 10.2337/dc12-2469
Keywords: GLP-1 - glucagon-like peptide-1, incretin, GLP-1 agonists, hormone, inflammation, anti-inflammation
Citation: Mehdi SF, Pusapati S, Anwar MS, Lohana D, Kumar P, Nandula SA, Nawaz FK, Tracey K, Yang H, LeRoith D, Brownstein MJ and Roth J (2023) Glucagon-like peptide-1: a multi-faceted anti-inflammatory agent. Front. Immunol. 14:1148209. doi: 10.3389/fimmu.2023.1148209
Received: 19 January 2023; Accepted: 26 April 2023;
Published: 17 May 2023.
Edited by:
Christoph Thiemermann, Queen Mary University of London, United KingdomReviewed by:
Marcin Filip Osuchowski, Ludwig Boltzmann Institute for Experimental and Clinical Traumatology, AustriaBasilia Zingarelli, Cincinnati Children’s Hospital Medical Center, United States
Copyright © 2023 Mehdi, Pusapati, Anwar, Lohana, Kumar, Nandula, Nawaz, Tracey, Yang, LeRoith, Brownstein and Roth. This is an open-access article distributed under the terms of the Creative Commons Attribution License (CC BY). The use, distribution or reproduction in other forums is permitted, provided the original author(s) and the copyright owner(s) are credited and that the original publication in this journal is cited, in accordance with accepted academic practice. No use, distribution or reproduction is permitted which does not comply with these terms.
*Correspondence: Jesse Roth, jroth2@northwell.edu
†These authors have contributed equally to this work and share first authorship