- 1State Key Laboratory for Diagnosis and Treatment of Infectious Disease, Collaborative Innovation Center for Diagnosis and Treatment of Infectious Diseases, Zhejiang University, Hangzhou, China
- 2Department of Basic Medical Sciences, Shulan International Medical College, Zhejiang Shuren University, Hangzhou, China
- 3Department of Medicine and pharmacy, Ocean University of China, Qingdao, China
- 4Key Laboratory of Endocrine Gland Diseases of Zhejiang Province, Hangzhou, China
- 5Otolaryngology & Head and Neck Center, Cancer Center, Department of Head and Neck Surgery, Zhejiang Provincial People’s Hospital (Affiliated People’s Hospital, Hangzhou Medical College), Hangzhou, China
- 6Institute of Plant Protection and Microbiology, Zhejiang Academy of Agricultural Sciences, Hangzhou, China
Endogenous indole and its derivatives (indoles), considered as promising N-substituted heterocyclic compounds, are tryptophan metabolites derived from intestinal microbiota and exhibit a range of biological activities. Recent studies indicate that indoles contribute to maintaining the biological barrier of the human intestine, which exert the anti-inflammatory activities mainly through activating AhR and PXR receptors to affect the immune system’s function, significantly improving intestinal health (inflammatory bowel disease, hemorrhagic colitis, colorectal cancer) and further promote human health (diabetes mellitus, central system inflammation, and vascular regulation). However, the revealed toxic influences cannot be ignored. Indoxyl sulfate, an indole derivative, performs nephrotoxicity and cardiovascular toxicity. We addressed the interaction between indoles and intestinal microbiota and the indoles’ effects on human health as double-edged swords. This review provides scientific bases for the correlation of indoles with diseases moreover highlights several directions for subsequent indoles-related studies.
1 Introduction
A person’s intestine is filled with trillions of bacteria, far exceeding any other microbial population on the body’s surface (1–3). As a complex microecosystem, intestinal bacteria are closely related to the host and play a core role in regulating physiological functions related to nutrition, immune system activation, and host defense (4). One of the main ways the intestinal flora interacts with the host is through metabolites (5, 6). Short-chain fatty acids (7–9) and bile acids (10) are metabolites from intestinal flora that contribute to intestinal health. Recent data indicate that indoles generated by tryptophan (Trp) metabolism are essential factors in intestinal homeostasis, closely related to intestinal microecology and human health (11, 12).
Trp is an essential nutrient mainly consumed through external foods but cannot be synthesized endogenously. Trp plays an essential part in regulating intestinal immune tolerance, maintaining symbiotic microbial homeostasis, and suppressing inflammation (13). Dietary tryptophan deficiency led to impaired immune function and altered gut in mice (14). Tryptophan-containing diets reduced inflammatory response and improved colonic inflammation and severity induced by sodium dextran sulfate in mice (15). The role of dietary Trp in modulating host immunity was closely related to the endogenous metabolites of Trp (16). Trp metabolism is a multi-path and complex process occurring in the host and its intestinal symbiotic microbiota, which has been extensively studied. In addition to being used for protein synthesis, Trp in the gut is metabolized through three main pathways: 1) the kynurenine pathway (95% of ingested Trp). 2) bacterial Trp metabolism (4-6%). 3) the serotonin pathway (1-2%) (Figure 1). Most of the existing reviews focus on Trp (17–19) and kynurenine (20–22), and few reviews systematically describe the properties of indoles (23).
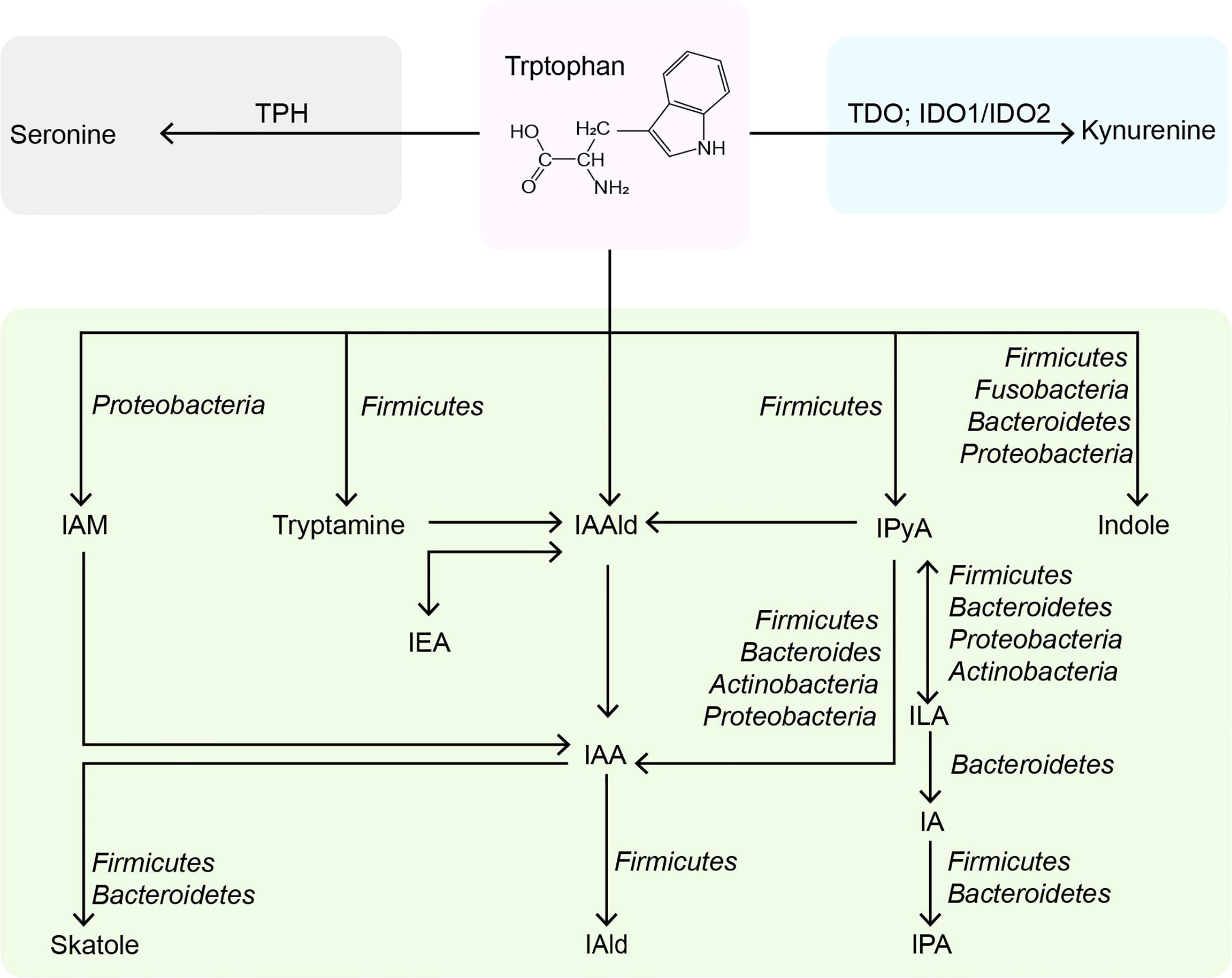
Figure 1 Metabolic pathways of tryptophan in the intestine. TPH, tryptophan hydroxylase; TDO, tryptophan 2,3-dioxygenase; IDO, indoleamine 2,3-dioxygenases; IAM, indole-3-acetamine; IAAld, indole-3-acetaldehyde; IPyA, indole-3-pyruvate; IEA, indole-3-ethanol; IAA, indole-3-acetate; ILA, indole-3-lactate; IAld, indole-3-aldehyde; IA, indole-3-acrylate; IPA, indole-3-propionate.
Endogenous indoles in the human body are mainly the bacterial catabolite of Trp. Bacterial-derived indoles are valued signal molecules between bacterial cells and play a prominent role in the microbial community that influences spore formation, plasmid stability, drug resistance, biofilm formation, and toxicity (24–29). Additionally, indoles strengthen the efficacy of intestinal epithelial cells (IECs) and suppress inflammation, regulate gut insulin secretion, and maintain the youth and health in animals, which may make it useful for humans (30–32). Indole skeleton is considered one of the most promising heterocyclic compound types with significant physiological and biological activities, including anti-cancer, anti-convulsant, anti-microbial, anti-tuberculosis, anti-malaria, and anti-viral (33, 34). Compared with indole from enterobacteria, synthetic indole compounds are more widely used in medicine, pesticides, food additives, etc., but they have toxicity and side effects (35, 36). Indole synthesized by enterobacteria has extensive benefits to human health and plays an increasingly important role in the intestinal tract (11). Thus, we reviewed the latest findings related to indoles from intestinal microbiota, focusing on how they promote intestinal immune homeostasis. We also summarized the beneficial effects of indoles on intestinal inflammation and other systemic diseases, and put forward the potentially toxic side effects. The purpose is to understand and reveal the further influence of indoles on human health. Besides, it could provide a reference for treating related diseases and developing new drugs in the future.
2 Indoles Derived From the Intestinal Flora
Trp is the primary precursor of indole derived from the intestinal flora. Most of the Trp is absorbed in the small intestine, and about 4% ~ 6% of Trp is catabolized into indoles by intestinal flora in the colon (37, 38). Typically, Gram-negative and Gram-positive bacteria, such as Escherichia coli E. coli), Clostridium spp., and Bacteroides spp., expressing tryptophanase, catalyze the direct conversion of Trp to indole (39). The widely studied E. coli import Trp through TnaB, and Trp is reversibly degraded into indole, pyruvic acid, and ammonia by tryptophanase TnaA (40). It has been found that more than 85 kinds of bacteria (Gram-negative and Gram-positive) metabolize dietary Trp to indole by tryptophanase (41). Indole affects bacterial physiology in a concentration-dependent manner (42). For instance, 0.5 mM affects bacterial movement (43), biofilm formation (44), and persister cell formation (45); 1-2 mM indole concentration influences the expression of multidrug exporters (46) and the secretion of specific virulence factors (47); 3-5 mM will inhibit cell division (48) and affect plasmid stability (49). And the concentration of indole is limited by the amount of exogenous Trp, so the concentration of indole in human feces is relatively high, ranging from 0.25 mM to 1.1 mM (31). The Kovács method is the most commonly used to detect the indole concentration in various biological samples (50). Still, it is complicated to operate and lacks specificity, so it is necessary to develop a simpler and more efficient quantitative method in the future. Although TnaA can metabolize the most Trp to indole, its reaction is only a small part of the microbial degradation pathway of Trp. Trp can also be hydrolyzed by decarboxylases, such as Ruminococcus gnavus and Clostridium sporogenes (C. sporogene), which produce the β-arylamine neurotransmitter tryptamine by decarboxylating Trp through the action of tryptophan decarboxylase (51). Tryptamine can regulate intestinal motility and immune function (52). It can also induce intestinal endocrine cells to release serotonin (53), which has impacts on human emotions, immunity, and bone development, and also involves the pathology of many diseases, including inflammatory bowel disease (IBD) and cardiovascular diseases (54).
Additionally, C. sporogenes can transform Trp into idole-3-pyruvic acid (IPyA), which is catalyzed by an indole-3-pyruvate decarboxylase to produce indole-3-acetaldehyde (IAAld) (11). Then, IAAld is decarboxylated by members of the phylum firmicutes, proteobacteria, bacteroides, and actinomycetes to produce indoleacetic acid (IAA) (55, 56). IAA is the precursor of indole-3-aldehyde (IAld) and 3-methylindole (skatole). The skatole is formed by decarboxylation of IAA, and Bacteroides thetaiotaomicron, Eubacterium rectale, and Butyrivibrio fibrisolvens have been proved to produce it. IAld is produced by the catabolism of Lactobacillus acidophilus (L. acidophilus), L. murinus, L. reuteri, and L. johnsonii in the firmicutes (57–59). Moreover, IPyA can produce indole-3-lactic acid (ILA), indoleacrylic acid (IA), and indole 3-propionic acid (IPA) through reduction and dehydration. Intestinal bacteria containing phenyllactate dehydrogenase (fldH) convert IPyA into ILA through a reduction reaction. ILA can produce IA through dehydration of Peptostreptococcus russellii (P. russellii), P. anaerobius and P. stomatis, phenyllactic dehydratase (fldBC) and its activator fldI are involved in this process (11). The acyl-CoA dehydrogenase enzyme converts IA to IPA, the final product of Trp reduction metabolism. Other bacteria, such as Lecheveria aerocoloniae, can make IPA by deaminating Trp with an amino acid oxidase (60). Among the enzymes listed above, fldAIBC should be worth mentioning. This gene cluster is only found in P. russellii and is preserved across the Peptostreptococcus genus (61). Although P. anaerobius also encodes the complete fldAIBC gene cluster, the concentrations of IPA and IA produced by it are significantly reduced. Furthermore, the fldAIBC cluster in C. sporogenes is identical to this gene cluster, which is assumed to be responsible for the conversion of Trp into ILA and IPA in C. sporogenes (39, 62). Additionally, comparable gene clusters have been discovered in C. cadaveris, C. botulinum, and P. anaerobius, indicating that they can produce IPA (62). At the same time, the presence of FLDC, a homologous cluster of the fldBC gene cluster, was discovered to be a reliable marker of IPA-producing bacteria (62). We show the metabolic pathway of indoles in the intestine and related bacteria (detailed to the phylum) in Figure 1.
Trp metabolism is complex, and numerous bacteria strains are involved in the manufacturing of indole derivatives (56). However, there are still many undiscovered strains that can catalyze Trp. To identify microorganisms and microbial genes involved in Trp metabolism regulation, it seems a feasible strategy to combine metabolomics with metagenomics and/or metatranscriptomics. Many metabolites mentioned above have been proved to be beneficial to the host, and they have important effects on the intestinal barrier function and even human health (61, 63).
3 Influence on Intestinal Function
The function of the intestinal barrier is strongly linked to intestinal health and plays a critical role in animal health. The gut barrier isolates the host from the microorganisms in the intestinal cavity and limits the movement of microorganisms and molecules from the intestinal lumen (64). The intestinal mucosal barrier consists of 4 main components: immune barrier, biological barrier, mechanical barrier, and mucus barrier (65). In the intestine, lymphoid tissue and immune cells make up the immunological barrier. The biological barrier is made of intestinal flora. The mechanical barrier consists of tight junctions (TJs) and the underlying gut epithelium. Chemicals such as lysozyme and digestive enzymes secreted by the intestine form the mucus barrier (Figure 2). An intact mucosal barrier system prevents microorganisms and products from migrating into the blood (66). Once the gut barrier is damaged and intestinal permeability increases, bacteria and their products, such as endotoxins, will translocate and activate the mononuclear macrophage system, promoting the production of a large number of inflammatory elements, such as interleukin (IL)-6 and tumor necrosis factor-α (TNF-α) leads to a chronic microinflammatory state (67, 68). Indoles are crucial in controlling intestinal barrier efficacy, including modulation of inflammatory and immunological responses, reduction of epithelial permeability, mucus production, and TJ formation. The principal effects of indoles on the intestinal immune barrier are summarized in Table 1.
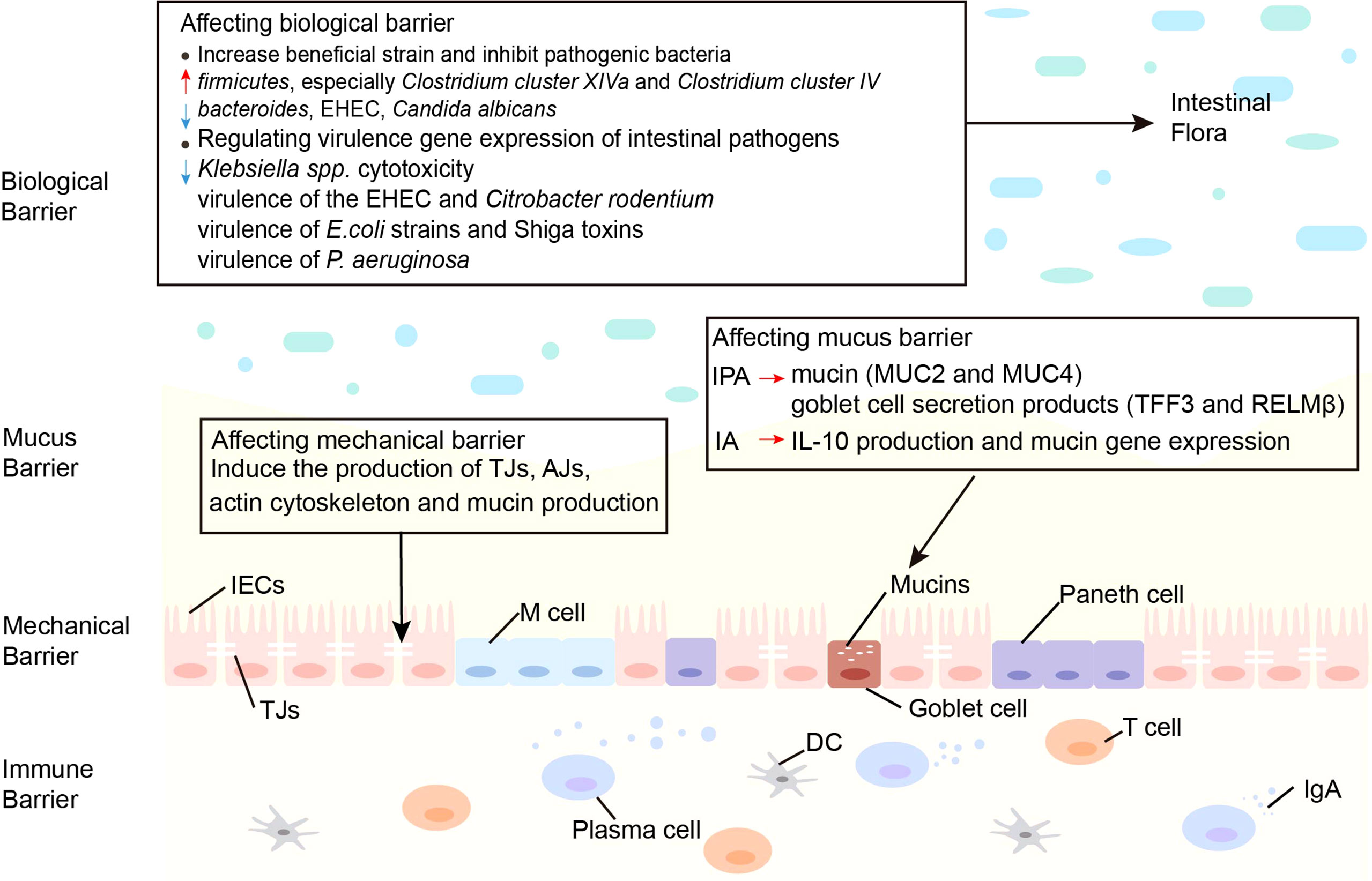
Figure 2 Effects of indoles on three barriers. Indoles affect the biological barrier by increasing beneficial bacteria, inhibiting pathogenic bacteria and regulating virulence gene expression of intestinal pathogens. Indoles enhance intestinal epithelial cell function by regulating several genes involved in mechanical barrier formation. Indoles increase mucin and goblet cell secretion products, which strengthen the mucus barrier. AJs, adherens junctions; DC, dendritic cell; E. coli, Escherichia coli; EHEC, enterohemorrhagic escherichia coli; IA, indoleacrylic acid; IECs, intestinal epithelial cells; IgA, immunoglobulin A; IL-10, interleukin-10; IPA, indole 3-propionic acid; MUC, mucin; M cell, membranous cell; P. aeruginosa, Pseudomonas aeruginosa; TJs, Tight junctions.
3.1 Affecting the Intestinal Immune Barrier
3.1.1 Indole Enhances Immune Barrier
The use of indole as a therapy for non-steroidal anti-inflammatory drugs (NSAIDs) enteropathy was investigated (77). Indole decreases the fecal calprotectin concentrations and the infiltration of neutrophils in the spleen and mesenteric lymph nodes induced by indomethacin. Combining indole with indomethacin leads to a reduction in NSAID-induced mucosal transcriptome changes. Fecal calprotectin is a neutrophil-specific biomarker of intestinal inflammation. And neutrophils are considered the key factor in the pathogenesis of NSAIDs enteropathy, which can cause inflammation and tissue damage by releasing a variety of inflammatory mediators (78). This indicates that indole can reduce the intestinal inflammation caused by indomethacin in mice, and has a potential immunomodulatory effect on NSAID enteropathy.
Bansal et al. found that indole reduced the creation of the pro-inflammatory cytokine IL-8 and the expression of nuclear factor κB (NF-κB) activated by chemokine TNF-α and induced the secretion of the anti-inflammatory cytokine IL-10 (31). Studies have shown that symbiotic bacteria can limit the activation of NF-κB induced by Salmonella typhimurium and reduce inflammation in mice (79). Therefore, indole may be a signal that probiotics reduce intestinal inflammation.
3.1.2 Indole Derivatives Enhance Immune Barrier
IPyA is an aromatic pyruvic acid produced by the action of aromatic amino acid transaminases and is a precursor of ILA, IAA, and IAAld. IPyA had a substantial anti-inflammatory impact on the colon, in the IBD mouse model. IPA administration modified the constitution of T-cell subsets in the colonic lamina propria lymphocytes (LPL) and dendritic cells (DCs) subsets in the mesenteric lymph nodes (MLN). In T cell-mediated colitis model, IPyA induced T regulatory cell 1 (Tr1) differentiation but not Foxp3+ Treg differentiation in the colonic LPL (69). Tr1 secretes a large amount of IL-10 and is important for intestinal immunological homeostasis (80). Simultaneously, IPyA reduces the frequency of Th1 cells by attenuating the ability of MLN DC to induce Th1 cell differentiation. Th1 cells are involved in the etiology of colitis (81), it appears that IPA decreases colonic inflammation by inhibiting Th1 cell production. IPyA administration modified the constitution of MLN DC subpopulations, mainly by decreasing the frequency of CD103-CD11b+ DCs and increasing the frequency of CD103+ CD11b- DCs in MLN. CD103-CD11b+DCs promote the differentiation of effector T cells that produce pro-inflammatory cytokines IFN-γ and IL-17. Besides, CD103- DCs are greatly inflammatory during chronic colitis (82). This is sufficient to demonstrate that changing the composition of MLN DC subsets has an effect on the improvement of intestinal inflammation. IPyA suppresses colonic inflammation by boosting IL-10-producing T cells and lowering Th1 cells in the lamina propria of the colon. IPyA administration alters the composition of the MLN DC subpopulation, suggesting that IPyA has an important effect on improving the intestinal immune barrier.
IA of bacterial origin increased IL-10 production and reduced TNF production in an LPS-stimulated co-culture (a co-culture system consisting of mini-gut spheroid cultures and BMDMs) (61). IL-10 is crucial for cupped cells to keep producing mucin (MUC) (83). According to research, IA may have a considerable anti-inflammatory effect on the colon. In addition to the activation of aryl hydrogen receptor (AhR), treating human PBMCs with IA activated nuclear factor erythroid 2-related factor 2 (NRF2)- antioxidant response element (ARE) pathway and secreted less IL-6 and IL-1β. NRF2 is a transcription factor that stimulates the ARE pathway in cells, inhibits the pro-inflammatory signaling pathway, and activates the AhR signaling pathway (84, 85). Furthermore, RNA sequencing demonstrated that IA therapy resulted in the activation and/or differentiation of innate immune cells (e.g., CD14, CCL2, MT2A, CYBB, IL6, and PTAFR), as well as genes involved in inflammation and oxidative stress (e.g., FPR2, LRRC25, CPM, MS4A7, and SLC7A7) (61). The findings above point to IA inducing potent anti-inflammatory and antioxidant effects in humans.
Production of IAA by Bacteroides ovatus increases the abundance of CD103+/CD11c+ immune populations (70), and immunological cells that are CD103+/CD11c+ are critical for maintaining intestinal immune homeostasis and inducing tolerogenic immune responses (86). In addition, IAA binds to the AhR on DCs and drives the production of IL-22. In individuals with IBD, IL-22 stimulates epithelial regeneration and decreases inflammation, and it plays a key role in the regulation of intestinal inflammation (87). In vivo, activation of the IL-22 receptor increases the expression of genes involved in immune surveillance, epithelial barrier function, inflammation, and homeostasis (88). Indole production by other Bacteroides besides Bacteroides ovatus can also activate AhRs on immune cells, leading to IL-22 production. Still, no research on Bacteroides, indole synthesis, and IL-22 secretion has been conducted, so further studies are needed to verify the possibility that IAA produced by other Bacteroides activates IL-22 and reduces colitis. In the presence of LPS, Reg4 expressed in intestinal epithelial cells maintains immune homeostasis by increasing the proportion of Lactobacillus and its metabolite IAA, which promotes the production and accumulation of IL-35 + B regulatory (Breg) cells in intestinal tissues (71). IL-35-producing Breg cells are key immune regulators of many diseases, including autoimmune and infectious diseases and cancer progression (89). IL-35 expression is dysregulated in inflammatory autoimmune diseases such as IBD, multiple sclerosis, type 1 diabetes, and autoimmune hepatitis (90). These results suggested that IAA can regulate the differentiation and production of IL-35+ cells, and affect the intestinal immune barrier. After being treated with Trp (LAB + Trp), Lactobacillus plantarum KLDS 1.0386 elevated IAA levels in the colon. IAA further upregulated the expression of AhR mRNA to stimulate the IL-22/signal transducer and transcription (STAT) 3 signaling pathwayactivator, increased the formation of antimicrobial peptides like regenerated islet-derived protein 3β and regenerated islet-derived protein 3γ, and improved gut immune function (72).
Lactobacillus reuteri produces ILA, an indole derivative of Trp, which activates AhR in CD4+ T cells and downregulates the transcription factor ThpoK, allowing CD4+ T cells to differentiate into CD4+CD8αα+ double-positive intraepithelial T lymphocytes (DPIELs) with immunomodulatory functions (59). Through these mechanisms, ILA promotes intestinal barrier function and reduces inflammation. Bifidobacterium longum subsp. Infantis living in the gastrointestinal tract of breast-fed infants can also produce ILA, which significantly attenuates TNF-α and LPS-induced increases in the proinflammatory cytokine IL-8 in intestinal epithelial cells. ILA also increases mRNA expression of the AhR-target gene CYP1A1 and NRF2-targeted genes glutathione reductase 2, superoxide dismutase 2, and NAD(P) H dehydrogenase, which may be important modulators of intestinal inflammation in breastfeeding infants (73). In addition, another study showed that ILA secreted by B. infantis has anti-inflammatory impacts on the immature intestine (91). These data provide important insights for the production of ILA-producing probiotics and dietary recommendations.
IPA downregulated the intestinal epithelial cell-mediated inflammatory cytokine TNF-α, while upregulating the ligand protein-encoding mRNAs (74), thereby regulating the intestinal barrier function and relieving intestinal inflammation. IPA also regulated intestinal immune homeostasis by significantly inducing IL-10 receptor ligand-binding subunit (IL-10R1) on intestinal epithelial cells through activation of AhR (75). IL-10R1 is a receptor for the anti-inflammatory cytokine IL-10, and IL-10 sends anti-inflammatory signals through IL-10R1 that inhibits the excessive release of pro-inflammatory mediators from various cells, including IEC. This further illustrates the protective effect of IPA on the intestinal immune barrier.
IAld produced by Lactobacillus contributed to AhR-dependent IL22 transcription (58), and the IL-22 producer inhibited inflammation and protected the immune physiology of the mucosal surface. IAld could replace probiotics to protect and maintain mucosal integrity during infection or chemical injury. IAld might be used as a supportive therapy during flora processing and intestinal flora dysbiosis. Lactobacillus also stimulated IL-22 secretion by LPL through IAld-induced AhR, which activated the pSTAT3 pathway and inhibited IL-1β and TNF-α secretion to protect the mucosal immune barrier (76).
3.2 Affecting the Intestinal Biological Barrier
3.2.1 Increase Beneficial Strains and Inhibit Pathogenic Bacteria
The gut microbiome is dominated by gram-negative bacteria when taking NSAIDs, a shift that can cause intestinal damage. Co-administration of indole and indomethacin can maintain or even increase the important members of firmicutes, especially C. cluster XIVa and C. cluster IV, which are crucial to intestinal homeostasis. It seems that it can prevent any increase of bacteroides. Intestinal mucosal injury can be reduced with this change (77). Indole may increase the resilience of HCT-8 cells to norepinephrine-mediated enterohemorrhagic Escherichia coli (EHEC) settlement through multiplying expression of the MUC gene (31). IAld activates AhR to produce IL-22, and IL-22 regulated mucosal responses that allow mixed microbial communities to survive and suppress Candida albicans colonization (58).
3.2.2 Regulating Virulence Gene Expression of Intestinal Pathogens
Indoles modulate virulence factors in a variety of intestinal bacteria. Indole enhances the conversion of tilivamycin to tilivalline. Both are produced by Klebsiella spp., with the difference that tilivalline is an indole analogue with reduced cytotoxicity, and tilivalline binds to upregulate progesterone X receptors (PXR) reactive detoxification genes and inhibits microtubule protein directed toxicity. Thus, indole alleviates Klebsiella spp. cytotoxicity in a multifunctional manner (92). Indole generated by the metabolism of intestinal probiotics has a high concentration in the intestinal lumen, which reduces the demonstration of the pathogenic gene of the intestinal germ EHEC and Citrobacter rodentium (29). Indole is also used as ToxR agonist to regulate virulence gene expression and biofilm production of Vibrio cholerae in the intestine (93). Indole, ICA, and IAA also down-regulate the virulence of pathogenic E. coli strains and the production of Shiga toxins (94). Moreover, the virulence gene expression of Pseudomonas aeruginosa is significantly altered by indole and 7‐hydroxyindole, which lower virulence factors and decrease swarming motility. P. aeruginosa less colonizes the guinea pig lung, and there is better clearance in the gastrointestinal tract after treatment with 7-hydroxyindole (95). As a result, indoles may be helpful as therapeutic agents against pathogens such as EHEC.
3.3 Affecting the Intestinal Mechanical Barrier
Indole enhances the barrier function of ECs in vitro by inducing the expression of many genes involved in IECs, including TJ, adherens junction (AJ), actin cytoskeleton, and MUC formation (96). The human intestinal epithelial cell line HCT-8 was exposed to indole to measure changes in its gene expression. Results showed that high doses of indole enhanced molecular profiles associated with MUC production and mucosal barrier strengthening and interepithelial resistance in polarized cells of the intestinal epithelium, HCT-8 (31). Thus, Indole strengthens the mechanical barrier.
3.4 Affecting the Intestinal Mucus Barrier
IPA enhances the mucus barrier function by increasing MUC2 and MUC4, as well as goblet cell secretory products (TFF3 and RELMβ) (97). IPA also promotes IL-10 release, and the signal transduction of IL-10 enhances the mucus barrier function and maintains the steady-state of epithelial cells (75). IA from bacteria enhances the production of IL-10 and MUC gene expression, and IL-10 is critical for maintaining MUC production in goblet cells. Therefore, IA indirectly affects the mucus barrier through IL-10 (61).
4 Mechanism of Indoles Regulating Intestinal Tract
Indoles are critical molecules in host-microorganism interaction, and most of them play a protective role in the intestinal barrier. It mainly regulates the mucosal immune response by activating AhR and regulates the mucosal integrity by activating PXR, thus regulating intestinal homeostasis (98, 99). The mechanism by which indole regulates the intestinal barrier via AhR or PXR is shown in Figure 3.
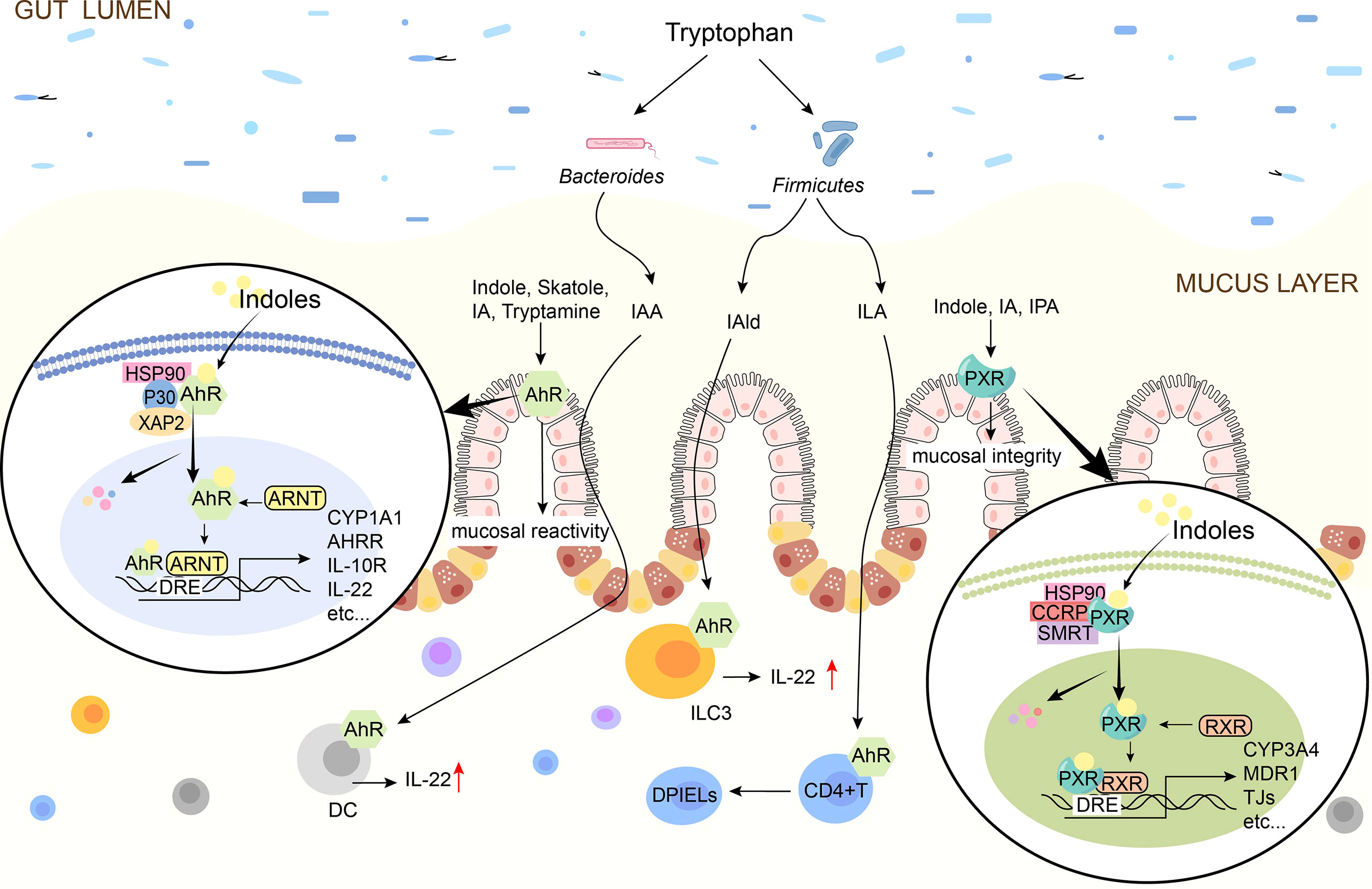
Figure 3 Schematic diagram of indoles signal pathway. Indole, Skatole, IA, Trytamine, IAA, IAld, ILA are AhR ligands. Indoles activates AhR to promote mucosal immunity, and AhR is present on intestinal epithelial cells and immune cells such as ILC3, DC. Indole, IA, IPA are the ligands of PXR and they activate PXR to affect tight junctions. In the absence of ligand, AhR/PXR exists in cytoplasm as an inactive complex. After indoles binding, AhR/PXR enters the nucleus, forms a dimer with ARNT/RXR, and then induces the expression of immune-related genes. IAA, indole-3-acetate; ILA, indole-3-lactate; IAld, indole-3-aldehyde; IA, indole-3-acrylate; IPA, indole-3-propionate; AhR, aryl hydrocarbon receptor; PXR, pregnane X receptor; TJ, tight junctions; IL, interleukin; DC, dendritic cells; DPIELs, double-positive intraepithelial T lymphocytes; ILC3, Group 3 innate lymphoid cells; HSP90, heat shock protein 90; P23, HSP90 co-chaperone p23; XAP2, X-associated protein 2; ARNT, AhR nuclear translocator.
4.1 Intermediation of Aromatic Hydrocarbon Receptor
Indole, tryptamine, Skatole, IAA, IA, and other indolic acid derivatives are considered as ligands of AhR (100) and play immune roles by activating AhR (38, 101). AhR is a key member of the basic helical-helix superfamily and is found in immune cells, ECs, and endothelial cells of intestinal barrier tissues (56, 102). Inactive AhR exists in the cytoplasm and binds to 2 molecules of heat shock protein 90 (Hsp90), 1 molecule of X-associated protein 2 (XAP2), and 1 molecule of Hsp90 co-chaperone p23 (P23) to form complexes, once activated by ligands, the conformation of the complex changes (99). AhR enters the nucleus and joins with AhR Nuclear Translocator (ARNT) to produce a functional heterodimer transcription factor complex (98). The dioxin reaction element is a constant DNA sequence that the AhR/ARNT heterodimer binds to (DRE) (103). The recruitment of coactivators to AhR’s trans-activation domain occurs when AhR/ARNT binds to DRE, which initiates the indication of a series of downstream target genes, such as inflammatory and immune-related cytokines IL-22, IL-17and IL-10, thereby exerting immunomodulatory effects (104, 105).
The cytokine IL-10 is produced by AhR indole and ICA, promoting intestinal regeneration and differentiation of goblet cells by altering intestinal homeostasis, protecting the epithelial barrier, and limiting inflammation associated with bacterial product transport, primarily during aging (32). Accordingly, indole may be useful for treating age-related diseases including inflammation and epithelial barrier disruption. IL-10 signals are induced during inflammation through the IL-10R1, attenuating the proinflammatory mediators produced excessively in various cell types, including IECs, enhancing mucosal barrier function and leading to epithelial cell maintenance and homeostasis. The activity of IL-10R1 in the epithelium of an AhR is modulated by IPA and IAld (75), which has been linked to protective effects in colitis models and improves epithelial wound healing (Table 2).
IL-22 is an essential cytokine that protects the host from inflammatory damage. Its main function in the intestine is to maintain intestinal epithelial integrity and enhance defense mechanisms against bacterial pathogens (106). IAld stimulates the presentation of IL-22 in an AhR-dependent manner and activates innate lymphoid cells, which prevents fungal Candida albicans from colonizing the mucosa and inflaming it (58). IAA acts as an AhR ligand that promotes Reg3 gene expression via stimulating the IL-22/STAT3 signaling pathway, which activates the immune system and improves the intestinal epithelial barrier, resulting in anti-inflammatory benefits (72) (Table 2).
4.2 Modulation of Pregnancy-X Receptor
PXR is one of the members of the nuclear receptor superfamily, is widely expressed in intestinal tissues, can be activated by a multiplicity of endogenous and exogenous substances (107), and regulates intestinal mucosal integrity mediated by toll-like receptor 4 (TLR4) (108). Indoles activate PXR and induce anti-inflammatory responses (109). It has been found that IPA acts as a ligand for PXR in vivo, and IPA downregulates enterocyte TNF-α while upregulating ligand-encoding mRNA and increasing the manifestation of TJs that regulate intestinal barrier function in terms of intestinal permeability and inflammation (74). IAA combined with the PXR receptor can cause the production of IL-35+B cells, promoting potent anti-inflammatory cytokine IL-35, which maintains intestinal homeostasis (71). In addition, indole and indole-3-acetamide have also been suggested as PXR agonists (110) (Table 2).
5 Indoles Improve Diseases
Indoles are absorbed by intestinal epithelial cells and diffuse into the blood, thus circulating to the whole body and affecting various systems, such as the regulation of intestinal and related diseases. We summarize their effects on IBD, hemorrhagic colitis, colorectal cancer (CRC), diabetes mellitus, central system inflammation, and vascular regulation (Figure 4).
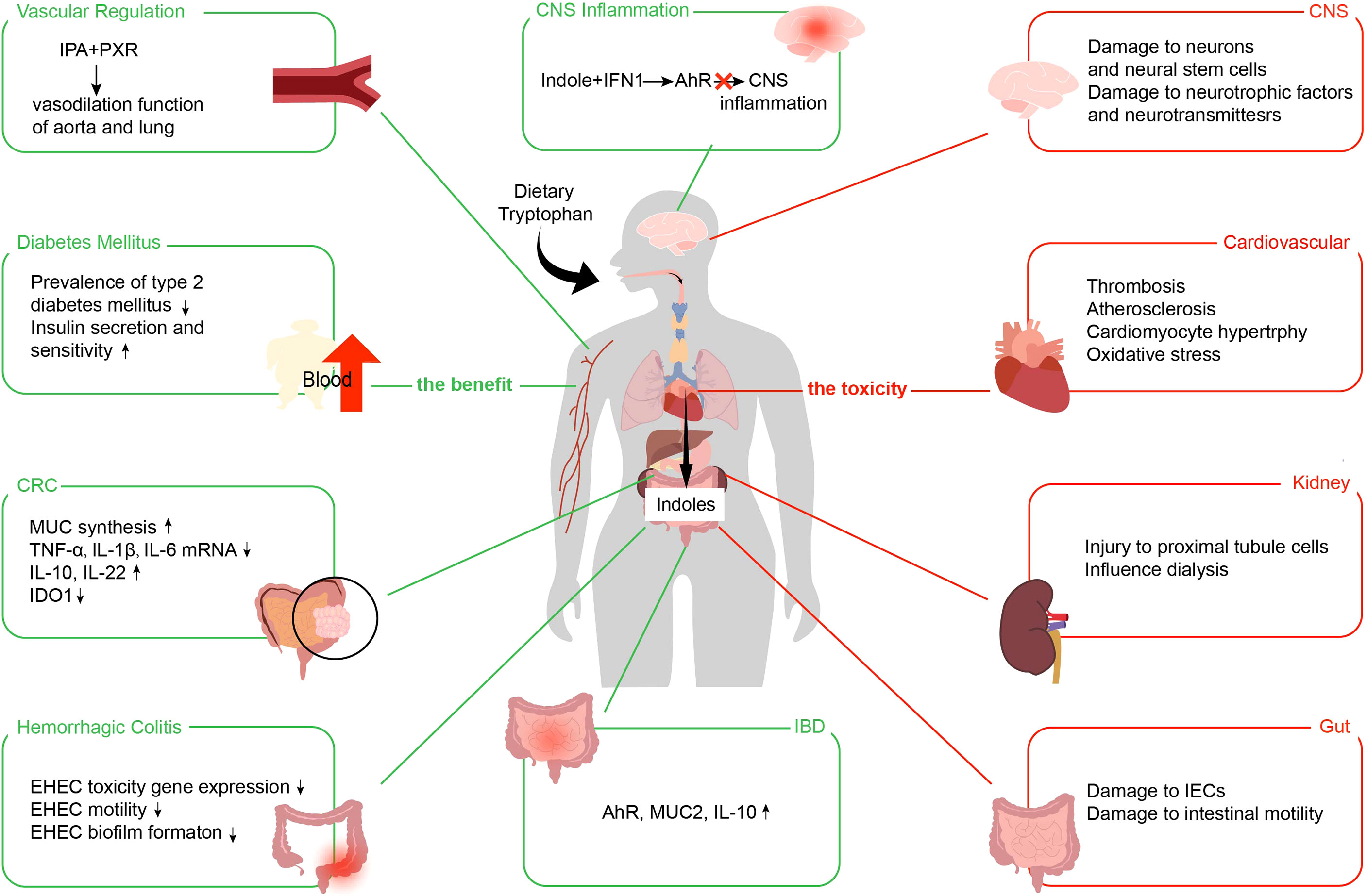
Figure 4 Benefits and toxic effects of indoles. The benefits of indoles use the green line. The toxicities of indoles use the red line. IPA, indole 3-propionic acid; PXR, pregnane X receptor; IFN, type I interferon; AhR, aryl hydrocarbon receptor; CNS, central nervous system; CRC, colorectal cancer; MUC, mucin; TNF-α, tumor necrosis factor-α; IL, interleukin; IDO1, indoleamine 2,3-dioxygenase; EHEC, enterohemorrhagic Escherichia coli; IBD, inflammatory bowel disease; IECs, intestinal epithelial cells.
5.1 Indoles Relieves IBD
IBD is the general name of a group of diseases, including Crohn’s disease and ulcerative colitis, and it is an important chronic gastrointestinal inflammatory disease clinically (111). However, its pathogenesis is still unclear, and its treatment methods are limited. A large number of studies have shown that indoles play a certain role in relieving symptoms of IBD (101, 112).
IBD is linked to the mucus layer, and IBD patients have a thinner internal mucus layer and lower MUC2 glycosylation. It shows that IA treatment of murine-derived colonic spheroids significantly increases AhR activation and MUC2 gene expression. IA maintained its effect on MUC2 gene expression and increased IL-10 production even in the presence of LPS-stimulated co-culture. The generation of MUC by goblet cells is dependent on IL-10. As a result, IA produced from bacteria has the potential to increase IL-10 production and MUC gene expression, which may be advantageous to IBD patients (61).
The fecal samples from patients with IBD have been reported to induce less AhR activation than the fecal samples from healthy subjects, which has been ascribed to reduced amounts of IAA, a microbial AhR agonist, in the fecal samples from patients with IBD. Besides, research on mice lacking the IBD susceptibility gene Card9 showed that the absence of an altered microbiome capable of generating AhR agonists was linked to an increased risk of colitis (113). These data suggest that delivering adequate AhR agonists to the gut might be a promising technique for treating IBD. Indoles such as IAld have been proven to be effective AhR activators and may be effective immunomodulatory therapies for patients with IBD (58). Therefore, whether the products of indole metabolic pathway can be used as clinical drugs for IBD intervention is a scientific problem worth exploring.
5.2 Indoles Alleviate Hemorrhagic Colitis
Indole is vital for the pathogenesis of EHEC. Hemorrhagic colitis, a bloody type of diarrhea and can lead to a hemolytic uremic syndrome, is caused by the human pathogen E. coli O157:H7. During gastrointestinal infection, E. coli O157:H7 is revealed to a series of signaling molecules, containing bacterial signaling molecules like indoles, that have been implicated in the control of phenotypes important to EHEC infection, including virulence and infection (114). Indole decreased gene expression in EHEC implicated in surface colonization and pathogenicity, according to a DNA microarray study of surface-associated EHEC (43).
Indole can be oxidized by oxygenase to produce new interspecific biofilm signals, which affecting the same phenotypic (biofilm production) in a wide range of ways. Indole generated by E. coli K-12 and other symbiotic bacteria in the intestine might limit the production of E. coli K-12 biofilm and diminish the motility of non-pathogenic E. coli by changing the expression of SdiA, thus influencing EHEC chemotaxis and adhesion (114). Adhesion assays confirmed that indoles reduce the attachment of EHEC to ECs in vitro (43).
In conclusion, indoles attenuated EHEC infection and improved hemorrhagic colitis by changing the expression of EHEC toxicity genes, reducing its motility and biofilm formation, and reducing adhesion.
5.3 Indoles Remit Colorectal Cancer
According to mounting data, intestinal indoles, particularly Trp metabolites, appear to play an important role in CRC (37). Indoles stimulate AhR, which can work directly on intestinal stem cells to sustain MUC synthesis and improve intestinal barrier function (115). However, blocking the indole-AhR signaling pathway increased TNF-α, IL-1β, and IL-6 mRNA levels considerably in the inflammation-associated colorectal carcinogenesis model (115). Indoles also boost the production of the anti-inflammatory cytokines IL-10 (31) and IL-22 (58). In addition, tryptamine, the indole derivative, inhibits the enzyme indoleamine 2,3-dioxygenase (IDO1), which is involved in tumor immune tolerance (116). These findings imply that indoles generated by gut flora may slow the progression of CRC.
Alterations in microbial Trp metabolism are also characteristic of CRC. CRC patients had a lower indole/Trp ratio and a greater kynurenine (kyn)/Trp ratio than healthy individuals (115). In CRC, the expression of Kyn and IDO1 increase, and the production of indole decreases (117, 118). Increased IDO1 activity and increased Trp depletion cause activated T lymphocytes to enter a cell cycle arrest, leading to apoptotic T cell death and promoting immunosuppression of the tumor microenvironment increases and indole production decreases (119–121). The decline in indole production attenuated the inhibition of colon cancer. Overall, evidence suggests that the altered microbial Trp-indole metabolic pathway plays a role in CRC pathogenesis. As a result, identifying the role of indoles in CRC pathogenesis is critical for developing possible treatment methods.
5.4 Indoles Mitigate Diabetes Mellitus
A higher serum concentration of IPA reduces the possibility of developing type 2 diabetes mellitus and enhances insulin secretion and sensitivity. Studies have found that compared with rats fed the control diet, the fasting glucose level of rats fed the IPA diet was significantly reduced (122). According to additional research, indole can modulate the release of glucagon-like peptide-1 (GLP-1) from mouse fibroblasts in the colon (30).
Indole increases GLP-1 release during a short exposure period, reducing its secretion over a longer period. These action results arise because indole can influence the two critical chemical pathways of L cells. To some degree, indole can inhibit volt-gated K+ channels, increase action potentials duration induced by L cells, and cause a large increase in Ca2+ entry, which boosts GLP-1 secretion quickly. Indole inhibits NADH dehydrogenase, slowing ATP generation and lowering GLP-1 release over time (30).
Exposure to similar indole concentrations in the human colon can regulate incretin secretion by l-secreting cells and modify GLP-1 released by endocrine cells, which is critical for increasing insulin secretion by pancreatic beta cells, lowering appetite, and slowing stomach emptying.Therefore, indole at the intestinal level may affect appetite (123). At the same time, IPA has a potent ability to resist oxidative stress, suggesting that this metabolite may protect beta cells from metabolic and oxidative stress-related damage and amyloid accumulation (124). Therefore, IPA produced by intestinal microbiota metabolism is protective in against type 2 diabetes.
5.5 Indoles Abate Central System Inflammation
A neurological condition caused by an autoimmune response is multiple sclerosis (MS). As a central nervous system (CNS) cell, astrocytes are thought to play an important role in MS progression. Indole can be used as a precursor for synthesising AhR agonist Indoxyl sulfate (IS) in the liver and combining it with a type I interferon (IFN1) signal. Indole produced by the breakdown of intestinal flora bind to IFN1 produced in the CNS, which causes CNS inflammation and is activated and suppressed by AhR signaling in astrocytes. Activating AhR signals in astrocytes inhibits CNS inflammation in animal models of MS experimental autoimmune encephalomyelitis (125).
Indole, a gut microbiota metabolite, can reduce inflammation in the central nervous system by modulating AhR (39). Therefore, targeting gut microbiota associated with indole by regulating endogenous gut microbiota may be an alternative strategy for preventing and treating MS and other neurological diseases.
5.6 Indoles Lighten Vascular Regulation
IPA could trigger the PXR, a biomass-activated nuclear receptor in various tissues, comprising the vascular endothelium, to regulate endothelial function. IPA regulates agonist-induced endothelium-dependent relaxation in aorta and pulmonary artery catheters through PXR. This regulation is mainly due to the alteration of nitric oxide produced by endothelial nitric oxide synthase, which is repressed by the IPA-mediated activation of PXR (126). However, an antibiotic treatment that destroys the intestinal microbiota and reduces IPA abundance changes the vasodilator effects of IPA by changing the endothelium PXR pathway. The IPA supplement of microbial metabolism could raise systemic IPA levels as well as stimulate PXR expression, thereby reversing the agonist-induced enhancement of endothelium-dependent vasodilator in the aorta and pulmonary arteries caused by antibiotic treatment (127). The interaction between indole and blood vessels may significantly change the antibiotic treatment related to traditional infectious diseases or colon surgery, resulting in disorder in the microbial community.
6 Potential Side Effects of Indoles
Although indoles are essential in improving intestinal and even systemic diseases, they still cause negative effects, such as IS, indolyl-β-d-glucosinolate, and IAA (128). IS is co-metabolized by intestinal flora and host. Intestinal flora decomposes Trp in food with an enzyme to generate indoles, which are then carried by the portal vein to the liver and transformed into IS under the action of cytochrome P450 enzyme and sulfotransferase (129). IS is one of the most important nephrotoxic metabolites, and its nephrotoxicity has been widely confirmed in basic and clinical studies (130, 131). In addition, indoles emerge toxicity in the gastrointestinal systems, nervous and cardiovascular. We show the toxic effects of indoles in Figure 4.
IS induces IEC damage by up-regulating IRF1 expression, inhibiting dynamin-related protein 1 expression, and interfering with mitochondrial autophagy flux. IS causes oxidative stress in IEC-6 cells by increasing the release of reactive oxygen species in a concentration-dependent way (132). IS also suppresses NRF2 activation, reducing the antioxidant defense cell system and suppressing heme oxygenase-1, NAD(P)H dehydrogenase, and superoxide dismutase expression. In IS-treated IEC-6 cells, connexin 43 is more prevalent in the cytoplasm and nucleus than at the membrane level, resulting in decreased gap junction communication and cell motility. In addition, nucleoconnexin 43 is associated with reduced cell proliferation and significantly promotes intestinal changes associated with chronic kidney disease (CKD) (133). In IEC-6 cells, IS treatment induced significantly increased TNF-α release, cyclooxygenase-2 and inducible nitric oxide synthase production, and nitrotyrosine synthesis, suggesting that IECs are targets of IS-induced intestinal inflammation (134). Colons incubated with IS showed reduced contractility, suggesting that the toxin may have deleterious effects on colonic smooth muscle cells and cause injured intestinal motility (135).
The microbiota metabolite, IS, has a pathogenic role in developing CNS diseases. It is well known that CKD and cardiovascular disease are frequently caused by IS, a protein-bound uremic toxin (136). Additionally, renal insufficiency causes uremic toxins to accumulate in the brain, resulting in aberrant CNS function (137, 138). Secondly, IS can damage neurons and neural stem cells, impair neurotrophic factors and neurotransmitters, and induce oxidative stress and neuroinflammation. For example, by acting on CNS glial cells, IS promotes neuroinflammation and exhibits pro-inflammatory effects (139, 140). Intraperitoneal administration of IS to male C57BL/6 mice undergoing mono-kidney removal (141) revealed an accumulation of IS in blood, prefrontal cortex tissue, and liquor cerebrospinal. In contrast, the mice exhibited behavioral evidence of emotion disorder and neuron degeneration, such as anxiety, depression, and cognitive dysfunction. These Corresponding organic lesions accompanied these behavioral changes. This also suggests a seemingly pathological link between IS and CNS disorders.
In individuals with CKD, IS is linked to cardiovascular and all-cause mortality (142, 143). IS is an essential factor in developing cardiovascular disease in patients with hemodialysis. In hemodialysis, free form IS levels were found that have a positive correlation with Fibroblast growth factor 23 and inversely correlated with C-C motif chemokine 15, complement component C1q receptor, perlecan, bleomycin hydrolase, Cluster of differentiation 166 antigens, and signaling lymphocytic activation molecule family member 5 (144). These proteins serve a vital role in vascular repair and endothelial growth. IS can also cause thrombosis and atherosclerosis by increasing platelet hyperactivity, raising plasma procoagulant levels, and producing procoagulant particles (145, 146). IS affects heart tissue through increased inflammation, cardiac fibrosis, cardiomyocyte proliferation (147).
IS has been linked to the advancement of kidney disease (148), and there is strong evidence that it is harmful when accumulated under renal insufficiency. IS damages proximal tubule cells and induces inflammation and fibrosis development (149–152). Kidney achieves high clearance of IS by renal tubular secretion (153), whereas IS to plasma protein binds over 90%, which is limited by protein binding (154–157), and the plasma level of hemodialysis patients is relatively high, which also suggests the close link with renal disease.
7 Discussion and Conclusion
As intestinal bacteria are common metabolites, the important biological role of indoles cannot be ignored. Indoles are directly linked to the homeostasis of intestinal flora and the intestinal tract’s health, so indoles could indirectly affect other systems and the overall health of the human body. A recent study shows that, IAA, an indole derivative, can alleviate ankylosing spondylitis in mice by restoring intestinal microflora balance and decreasing the inflammatory response (158). Indoles have beneficial effects on human health, modulating the intestinal barrier and helping to maintain intestinal homeostasis by activating immune cells to release anti-inflammatory factors such as IL-22 (58), inhibiting the colonization of pathogenic bacteria including EHEC (31), inducing gene expression of TJs and AJ to reduce intestinal permeability (96), and increasing MUC expression to enhance mucus barrier function (22). Mainly, indoles in the regulation of intestinal microecology also play a key role. They inhibit harmful strains of bacteria and alter the virulence of intestinal pathogenic bacteria in a way that affects gene expression, which can help alleviate diseases such as hemorrhagic colitis (114). Simultaneously, indoles and their derivatives are crucial in activating AhR and PXR-mediated anti-inflammatory pathways. Examples include that IPA mediates downregulation of enterocyte TNF-α via PXR and upregulates mRNAs encoding for somatostatin to regulate intestinal permeability and intestinal barrier function in inflammation (74). These imply promising therapeutic pathways for indole and its derivatives.
Nevertheless, indole is also a double-edged sword, and a few derivatives also have certain disadvantages. For example, IS generated by the liver metabolism of indole has renal toxicity and cardiovascular toxicity at high concentrations (143), which can cause multiple system dysfunction by promoting oxidative stress, inflammation, and other pathological changes.
There is growing evidence that indoles play a crucial role in intestinal homeostasis and human health, but many questions remain addressed. Many intestinal bacteria were identified to metabolize Trp to indoles. Still, there may be unidentified bacteria, so the use of metabolomics and macro-genomics are needed in the future to further characterize unknown indole-producing bacteria and their associated metabolic pathways. Different indole concentrations exert other physiological functions. In addition, fecal indole concentrations are known in healthy adults, while the concentrations of many indole derivatives in the human intestine and blood remain unknown. Determining the concentrations of indole and its derivatives in different environments is obvious, but a quantitative method specifically for the detection of indole is lacking and simple and rapid assays need to be developed in the future. Most of the studies are based on animal models and involve only one indole. There are limitations in the experimental models and subjects, so further studies on the relationship between indoles and human health are still needed. In the future, there is a need to investigate the effects of multiple indole combinations on host physiology, and there is a need to integrate these findings with the clinical setting to develop new therapies for related diseases.
Author Contributions
Conceptualization, LL, WL, and XY. Writing – Original Draft Preparation, XY, HL, XZ, and SM. Writing – Review and Editing, XY, HL, XZ, SM, GZ and KA. All authors contributed to the article and approved the submitted version.
Funding
This work was supported by the National Key Research and Development Program of China (2018YFC2000500); start-up funds from Zhejiang Shuren University (2018R006); National Innovation and Entrepreneurship Program for College Students (202011842025).
Conflict of Interest
The authors declare that the research was conducted in the absence of any commercial or financial relationships that could be construed as a potential conflict of interest.
Publisher’s Note
All claims expressed in this article are solely those of the authors and do not necessarily represent those of their affiliated organizations, or those of the publisher, the editors and the reviewers. Any product that may be evaluated in this article, or claim that may be made by its manufacturer, is not guaranteed or endorsed by the publisher.
Supplementary Material
The Supplementary Material for this article can be found online at: https://www.frontiersin.org/articles/10.3389/fimmu.2022.903526/full#supplementary-material
References
1. Sender R, Fuchs S, Milo R. Are We Really Vastly Outnumbered? Revisiting the Ratio of Bacterial to Host Cells in Humans. Cell (2016) 164(3):337–40. doi: 10.1016/j.cell.2016.01.013
2. Chen ZR, Jin SF, Ma WB, Jiang RL. Intestinal Microecology: A Crucial Strategy for Targeted Therapy of Liver Diseases. Hepatobil pancreatic Dis Int HBPD Int (2021) 20(5):499–500. doi: 10.1016/j.hbpd.2021.07.007
3. Abdul Rahim MBH, Chilloux J, Martinez-Gili L, Neves AL, Myridakis A, Gooderham N, et al. Diet-Induced Metabolic Changes of the Human Gut Microbiome: Importance of Short-Chain Fatty Acids, Methylamines and Indoles. Acta Diabetol (2019) 56(5):493–500. doi: 10.1007/s00592-019-01312-x
4. Rooks MG, Garrett WS. Gut Microbiota, Metabolites and Host Immunity. Nat Rev Immunol (2016) 16(6):341–52. doi: 10.1038/nri.2016.42
5. Blacher E, Levy M, Tatirovsky E, Elinav E. Microbiome-Modulated Metabolites at the Interface of Host Immunity. J Immunol (Baltimore Md 1950) (2017) 198(2):572–80. doi: 10.4049/jimmunol.1601247
6. Sittipo P, Shim JW, Lee YK. Microbial Metabolites Determine Host Health and the Status of Some Diseases. Int J Mol Sci (2019) 20(21):5296. doi: 10.3390/ijms20215296
7. Rauf A, Khalil AA, Rahman UU, Khalid A, Naz S, Shariati MA, et al. Recent Advances in the Therapeutic Application of Short-Chain Fatty Acids (Scfas): An Updated Review. Crit Rev Food Sci Nutr (2021) 1–21. doi: 10.1080/10408398.2021.1895064
8. Donohoe DR, Garge N, Zhang X, Sun W, O'Connell TM, Bunger MK, et al. The Microbiome and Butyrate Regulate Energy Metabolism and Autophagy in the Mammalian Colon. Cell Metab (2011) 13(5):517–26. doi: 10.1016/j.cmet.2011.02.018
9. Sun M, Wu W, Liu Z, Cong Y. Microbiota Metabolite Short Chain Fatty Acids, Gpcr, and Inflammatory Bowel Diseases. J Gastroenterol (2017) 52(1):1–8. doi: 10.1007/s00535-016-1242-9
10. Wahlström A, Sayin SI, Marschall HU, Bäckhed F. Intestinal Crosstalk Between Bile Acids and Microbiota and Its Impact on Host Metabolism. Cell Metab (2016) 24(1):41–50. doi: 10.1016/j.cmet.2016.05.005
11. Li X, Zhang B, Hu Y, Zhao Y. New Insights Into Gut-Bacteria-Derived Indole and Its Derivatives in Intestinal and Liver Diseases. Front Pharmacol (2021) 12:769501. doi: 10.3389/fphar.2021.769501
12. Zhang B, Jiang M, Zhao J, Song Y, Du W, Shi J. The Mechanism Underlying the Influence of Indole-3-Propionic Acid: A Relevance to Metabolic Disorders. Front Endocrinol (2022) 13:841703. doi: 10.3389/fendo.2022.841703
13. Li X, Zhang ZH, Zabed HM, Yun J, Zhang G, Qi X. An Insight Into the Roles of Dietary Tryptophan and Its Metabolites in Intestinal Inflammation and Inflammatory Bowel Disease. Mol Nutr Food Res (2021) 65(5):e2000461. doi: 10.1002/mnfr.202000461
14. Hashimoto T, Perlot T, Rehman A, Trichereau J, Ishiguro H, Paolino M, et al. Ace2 Links Amino Acid Malnutrition to Microbial Ecology and Intestinal Inflammation. Nature (2012) 487(7408):477–81. doi: 10.1038/nature11228
15. Islam J, Sato S, Watanabe K, Watanabe T, Ardiansyah, Hirahara K, et al. Dietary Tryptophan Alleviates Dextran Sodium Sulfate-Induced Colitis Through Aryl Hydrocarbon Receptor in Mice. J Nutr Biochem (2017) 42:43–50. doi: 10.1016/j.jnutbio.2016.12.019
16. Agus A, Planchais J, Sokol H. Gut Microbiota Regulation of Tryptophan Metabolism in Health and Disease. Cell Host Microbe (2018) 23(6):716–24. doi: 10.1016/j.chom.2018.05.003
17. Hyland NP, Cavanaugh CR, Hornby PJ. Emerging Effects of Tryptophan Pathway Metabolites and Intestinal Microbiota on Metabolism and Intestinal Function. Amino Acids (2022) 54(1):57–70. doi: 10.1007/s00726-022-03123-x
18. Modoux M, Rolhion N, Mani S, Sokol H. Tryptophan Metabolism as a Pharmacological Target. Trends Pharmacol Sci (2021) 42(1):60–73. doi: 10.1016/j.tips.2020.11.006
19. Melhem NJ, Taleb S. Tryptophan: From Diet to Cardiovascular Diseases. Int J Mol Sci (2021) 22(18):9904. doi: 10.3390/ijms22189904
20. Liang Y, Xie S, He Y, Xu M, Qiao X, Zhu Y, et al. Kynurenine Pathway Metabolites as Biomarkers in Alzheimer's Disease. Dis Markers (2022) 2022:9484217. doi: 10.1155/2022/9484217
21. Cervenka I, Agudelo LZ, Ruas JL. Kynurenines: Tryptophan's Metabolites in Exercise, Inflammation, and Mental Health. Sci (New York NY) (2017) 357(6349):eaaf9794. doi: 10.1126/science.aaf9794
22. Chen LM, Bao CH, Wu Y, Liang SH, Wang D, Wu LY, et al. Tryptophan-Kynurenine Metabolism: A Link Between the Gut and Brain for Depression in Inflammatory Bowel Disease. J Neuroinflamm (2021) 18(1):135. doi: 10.1186/s12974-021-02175-2
23. Hendrikx T, Schnabl B. Indoles: Metabolites Produced by Intestinal Bacteria Capable of Controlling Liver Disease Manifestation. J Internal Med (2019) 286(1):32–40. doi: 10.1111/joim.12892
24. Lee JH, Wood TK, Lee J. Roles of Indole as an Interspecies and Interkingdom Signaling Molecule. Trends Microbiol (2015) 23(11):707–18. doi: 10.1016/j.tim.2015.08.001
25. Kim YG, Lee JH, Cho MH, Lee J. Indole and 3-Indolylacetonitrile Inhibit Spore Maturation in Paenibacillus Alvei. BMC Microbiol (2011) 11:119. doi: 10.1186/1471-2180-11-119
26. Chant EL, Summers DK. Indole Signalling Contributes to the Stable Maintenance of Escherichia Coli Multicopy Plasmids. Mol Microbiol (2007) 63(1):35–43. doi: 10.1111/j.1365-2958.2006.05481.x
27. Lee HH, Molla MN, Cantor CR, Collins JJ. Bacterial Charity Work Leads to Population-Wide Resistance. Nature (2010) 467(7311):82–5. doi: 10.1038/nature09354
28. Martino PD, Fursy R, Bret L, Sundararaju B, Phillips RS. Indole Can Act as an Extracellular Signal to Regulate Biofilm Formation of Escherichia Coli and Other Indole-Producing Bacteria. Can J Microbiol (2003) 49(7):443–9. doi: 10.1139/w03-056
29. Kumar A, Sperandio V. Indole Signaling at the Host-Microbiota-Pathogen Interface. mBio (2019) 10(3):e01031–19. doi: 10.1128/mBio.01031-19
30. Chimerel C, Emery E, Summers DK, Keyser U, Gribble FM, Reimann F. Bacterial Metabolite Indole Modulates Incretin Secretion From Intestinal Enteroendocrine L Cells. Cell Rep (2014) 9(4):1202–8. doi: 10.1016/j.celrep.2014.10.032
31. Bansal T, Alaniz RC, Wood TK, Jayaraman A. The Bacterial Signal Indole Increases Epithelial-Cell Tight-Junction Resistance and Attenuates Indicators of Inflammation. Proc Natl Acad Sci USA (2010) 107(1):228–33. doi: 10.1073/pnas.0906112107
32. Powell DN, Swimm A, Sonowal R, Bretin A, Gewirtz AT, Jones RM, et al. Indoles From the Commensal Microbiota Act Via the Ahr and Il-10 to Tune the Cellular Composition of the Colonic Epithelium During Aging. Proc Natl Acad Sci USA (2020) 117(35):21519–26. doi: 10.1073/pnas.2003004117
33. Garg V, Maurya RK, Thanikachalam PV, Bansal G, Monga V. An Insight Into the Medicinal Perspective of Synthetic Analogs of Indole: A Review. Eur J medicinal Chem (2019) 180:562–612. doi: 10.1016/j.ejmech.2019.07.019
34. Kumari A, Singh RK. Medicinal Chemistry of Indole Derivatives: Current to Future Therapeutic Prospectives. Bioorganic Chem (2019) 89:103021. doi: 10.1016/j.bioorg.2019.103021
35. Ma Q, Zhang X, Qu Y. Biodegradation and Biotransformation of Indole: Advances and Perspectives. Front Microbiol (2018) 9:2625. doi: 10.3389/fmicb.2018.02625
36. Han Y, Dong W, Guo Q, Li X, Huang L. The Importance of Indole and Azaindole Scaffold in the Development of Antitumor Agents. Eur J medicinal Chem (2020) 203:112506. doi: 10.1016/j.ejmech.2020.112506
37. Wyatt M, Greathouse KL. Targeting Dietary and Microbial Tryptophan-Indole Metabolism as Therapeutic Approaches to Colon Cancer. Nutrients (2021) 13(4):1189. doi: 10.3390/nu13041189
38. Gao J, Xu K, Liu H, Liu G, Bai M, Peng C, et al. Impact of the Gut Microbiota on Intestinal Immunity Mediated by Tryptophan Metabolism. Front Cell infection Microbiol (2018) 8:13. doi: 10.3389/fcimb.2018.00013
39. Roager HM, Licht TR. Microbial Tryptophan Catabolites in Health and Disease. Nat Commun (2018) 9(1):3294. doi: 10.1038/s41467-018-05470-4
40. Watanabe T, Snell EE. Reversibility of the Tryptophanase Reaction: Synthesis of Tryptophan From Indole, Pyruvate, and Ammonia. Proc Natl Acad Sci USA (1972) 69(5):1086–90. doi: 10.1073/pnas.69.5.1086
41. Lee JH, Lee J. Indole as an Intercellular Signal in Microbial Communities. FEMS Microbiol Rev (2010) 34(4):426–44. doi: 10.1111/j.1574-6976.2009.00204.x
42. Li G, Young KD. Indole Production by the Tryptophanase Tnaa in Escherichia Coli Is Determined by the Amount of Exogenous Tryptophan. Microbiol (Reading England) (2013) 159(Pt 2):402–10. doi: 10.1099/mic.0.064139-0
43. Bansal T, Englert D, Lee J, Hegde M, Wood TK, Jayaraman A. Differential Effects of Epinephrine, Norepinephrine, and Indole on Escherichia Coli O157:H7 Chemotaxis, Colonization, and Gene Expression. Infection Immun (2007) 75(9):4597–607. doi: 10.1128/iai.00630-07
44. Lee J, Jayaraman A, Wood TK. Indole Is an Inter-Species Biofilm Signal Mediated by Sdia. BMC Microbiol (2007) 7:42. doi: 10.1186/1471-2180-7-42
45. Vega NM, Allison KR, Khalil AS, Collins JJ. Signaling-Mediated Bacterial Persister Formation. Nat Chem Biol (2012) 8(5):431–3. doi: 10.1038/nchembio.915
46. Nikaido E, Shirosaka I, Yamaguchi A, Nishino K. Regulation of the Acrab Multidrug Efflux Pump in Salmonella Enterica Serovar Typhimurium in Response to Indole and Paraquat. Microbiol (Reading England) (2011) 157(Pt 3):648–55. doi: 10.1099/mic.0.045757-0
47. Nikaido E, Giraud E, Baucheron S, Yamasaki S, Wiedemann A, Okamoto K, et al. Effects of Indole on Drug Resistance and Virulence of Salmonella Enterica Serovar Typhimurium Revealed by Genome-Wide Analyses. Gut Pathog (2012) 4(1):5. doi: 10.1186/1757-4749-4-5
48. Chimerel C, Field CM, Piñero-Fernandez S, Keyser UF, Summers DK. Indole Prevents Escherichia Coli Cell Division by Modulating Membrane Potential. Biochim Biophys Acta (2012) 1818(7):1590–4. doi: 10.1016/j.bbamem.2012.02.022
49. Field CM, Summers DK. Indole Inhibition of Cole1 Replication Contributes to Stable Plasmid Maintenance. Plasmid (2012) 67(2):88–94. doi: 10.1016/j.plasmid.2011.11.004
50. Darkoh C, Chappell C, Gonzales C, Okhuysen P. A Rapid and Specific Method for the Detection of Indole in Complex Biological Samples. Appl Environ Microbiol (2015) 81(23):8093–7. doi: 10.1128/aem.02787-15
51. Williams BB, Van Benschoten AH, Cimermancic P, Donia MS, Zimmermann M, Taketani M, et al. Discovery and Characterization of Gut Microbiota Decarboxylases That Can Produce the Neurotransmitter Tryptamine. Cell Host Microbe (2014) 16(4):495–503. doi: 10.1016/j.chom.2014.09.001
52. Wlodarska M, Kostic AD, Xavier RJ. An Integrative View of Microbiome-Host Interactions in Inflammatory Bowel Diseases. Cell Host Microbe (2015) 17(5):577–91. doi: 10.1016/j.chom.2015.04.008
53. Takaki M, Mawe GM, Barasch JM, Gershon MD, Gershon MD. Physiological Responses of Guinea-Pig Myenteric Neurons Secondary to the Release of Endogenous Serotonin by Tryptamine. Neuroscience (1985) 16(1):223–40. doi: 10.1016/0306-4522(85)90059-4
54. Oliphant K, Allen-Vercoe E. Macronutrient Metabolism by the Human Gut Microbiome: Major Fermentation by-Products and Their Impact on Host Health. Microbiome (2019) 7(1):91. doi: 10.1186/s40168-019-0704-8
55. Russell WR, Duncan SH, Scobbie L, Duncan G, Cantlay L, Calder AG, et al. Major Phenylpropanoid-Derived Metabolites in the Human Gut Can Arise From Microbial Fermentation of Protein. Mol Nutr Food Res (2013) 57(3):523–35. doi: 10.1002/mnfr.201200594
56. Gasaly N, de Vos P, Hermoso MA. Impact of Bacterial Metabolites on Gut Barrier Function and Host Immunity: A Focus on Bacterial Metabolism and Its Relevance for Intestinal Inflammation. Front Immunol (2021) 12:658354. doi: 10.3389/fimmu.2021.658354
57. Wilck N, Matus MG, Kearney SM, Olesen SW, Forslund K, Bartolomaeus H, et al. Salt-Responsive Gut Commensal Modulates T(H)17 Axis and Disease. Nature (2017) 551(7682):585–9. doi: 10.1038/nature24628
58. Zelante T, Iannitti RG, Cunha C, De Luca A, Giovannini G, Pieraccini G, et al. Tryptophan Catabolites From Microbiota Engage Aryl Hydrocarbon Receptor and Balance Mucosal Reactivity Via Interleukin-22. Immunity (2013) 39(2):372–85. doi: 10.1016/j.immuni.2013.08.003
59. Cervantes-Barragan L, Chai JN, Tianero MD, Di Luccia B, Ahern PP, Merriman J, et al. Lactobacillus Reuteri Induces Gut Intraepithelial Cd4(+)Cd8αα(+) T Cells. Sci (New York NY) (2017) 357(6353):806–10. doi: 10.1126/science.aah5825
60. Nishizawa T, Aldrich CC, Sherman DH. Molecular Analysis of the Rebeccamycin L-Amino Acid Oxidase From Lechevalieria Aerocolonigenes Atcc 39243. J Bacteriol (2005) 187(6):2084–92. doi: 10.1128/jb.187.6.2084-2092.2005
61. Wlodarska M, Luo C, Kolde R, d'Hennezel E, Annand JW, Heim CE, et al. Indoleacrylic Acid Produced by Commensal Peptostreptococcus Species Suppresses Inflammation. Cell Host Microbe (2017) 22(1):25–37.e6. doi: 10.1016/j.chom.2017.06.007
62. Dodd D, Spitzer MH, Van Treuren W, Merrill BD, Hryckowian AJ, Higginbottom SK, et al. A Gut Bacterial Pathway Metabolizes Aromatic Amino Acids Into Nine Circulating Metabolites. Nature (2017) 551(7682):648–52. doi: 10.1038/nature24661
63. Konopelski P, Mogilnicka I. Biological Effects of Indole-3-Propionic Acid, a Gut Microbiota-Derived Metabolite, and Its Precursor Tryptophan in Mammals' Health and Disease. Int J Mol Sci (2022) 23(3):1222. doi: 10.3390/ijms23031222
64. Gao B, Emami A, Nath S, Schnabl B. Microbial Products and Metabolites Contributing to Alcohol-Related Liver Disease. Mol Nutr Food Res (2021) 65(5):e2000023. doi: 10.1002/mnfr.202000023
65. Mu Q, Kirby J, Reilly CM, Luo XM. Leaky Gut as a Danger Signal for Autoimmune Diseases. Front Immunol (2017) 8:598. doi: 10.3389/fimmu.2017.00598
66. Sabatino A, Regolisti G, Brusasco I, Cabassi A, Morabito S, Fiaccadori E. Alterations of Intestinal Barrier and Microbiota in Chronic Kidney Disease. Nephrol Dial Transplant (2015) 30(6):924–33. doi: 10.1093/ndt/gfu287
67. Andersen K, Kesper MS, Marschner JA, Konrad L, Ryu M, Kumar Vr S, et al. Intestinal Dysbiosis, Barrier Dysfunction, and Bacterial Translocation Account for Ckd-Related Systemic Inflammation. J Am Soc Nephrol (2017) 28(1):76–83. doi: 10.1681/ASN.2015111285
68. Vaziri ND, Zhao YY, Pahl MV. Altered Intestinal Microbial Flora and Impaired Epithelial Barrier Structure and Function in Ckd: The Nature, Mechanisms, Consequences and Potential Treatment. Nephrol Dial Transplant (2016) 31(5):737–46. doi: 10.1093/ndt/gfv095
69. Aoki R, Aoki-Yoshida A, Suzuki C, Takayama Y. Indole-3-Pyruvic Acid, an Aryl Hydrocarbon Receptor Activator, Suppresses Experimental Colitis in Mice. J Immunol (Baltimore Md 1950) (2018) 201(12):3683–93. doi: 10.4049/jimmunol.1701734
70. Ihekweazu FD, Engevik MA, Ruan W, Shi Z, Fultz R, Engevik KA, et al. Bacteroides Ovatus Promotes Il-22 Production and Reduces Trinitrobenzene Sulfonic Acid-Driven Colonic Inflammation. Am J Pathol (2021) 191(4):704–19. doi: 10.1016/j.ajpath.2021.01.009
71. Su X, Zhang M, Qi H, Gao Y, Yang Y, Yun H, et al. Gut Microbiota-Derived Metabolite 3-Idoleacetic Acid Together With Lps Induces Il-35(+) B Cell Generation. Microbiome (2022) 10(1):13. doi: 10.1186/s40168-021-01205-8
72. Shi J, Du P, Xie Q, Wang N, Li H, Smith EE, et al. Protective Effects of Tryptophan-Catabolizing Lactobacillus Plantarum Klds 1.0386 Against Dextran Sodium Sulfate-Induced Colitis in Mice. Food Funct (2020) 11(12):10736–47. doi: 10.1039/d0fo02622k
73. Ehrlich AM, Pacheco AR, Henrick BM, Taft D, Xu G, Huda MN, et al. Indole-3-Lactic Acid Associated With Bifidobacterium-Dominated Microbiota Significantly Decreases Inflammation in Intestinal Epithelial Cells. BMC Microbiol (2020) 20(1):357. doi: 10.1186/s12866-020-02023-y
74. Venkatesh M, Mukherjee S, Wang H, Li H, Sun K, Benechet AP, et al. Symbiotic Bacterial Metabolites Regulate Gastrointestinal Barrier Function Via the Xenobiotic Sensor Pxr and Toll-Like Receptor 4. Immunity (2014) 41(2):296–310. doi: 10.1016/j.immuni.2014.06.014
75. Alexeev EE, Lanis JM, Kao DJ, Campbell EL, Kelly CJ, Battista KD, et al. Microbiota-Derived Indole Metabolites Promote Human and Murine Intestinal Homeostasis Through Regulation of Interleukin-10 Receptor. Am J Pathol (2018) 188(5):1183–94. doi: 10.1016/j.ajpath.2018.01.011
76. Hou Q, Ye L, Liu H, Huang L, Yang Q, Turner JR, et al. Lactobacillus Accelerates Iscs Regeneration to Protect the Integrity of Intestinal Mucosa Through Activation of Stat3 Signaling Pathway Induced by Lpls Secretion of Il-22. Cell Death Differentiation (2018) 25(9):1657–70. doi: 10.1038/s41418-018-0070-2
77. Whitfield-Cargile CM, Cohen ND, Chapkin RS, Weeks BR, Davidson LA, Goldsby JS, et al. The Microbiota-Derived Metabolite Indole Decreases Mucosal Inflammation and Injury in a Murine Model of Nsaid Enteropathy. Gut Microbes (2016) 7(3):246–61. doi: 10.1080/19490976.2016.1156827
78. Kolaczkowska E, Kubes P. Neutrophil Recruitment and Function in Health and Inflammation. Nat Rev Immunol (2013) 13(3):159–75. doi: 10.1038/nri3399
79. O'Mahony C, Scully P, O'Mahony D, Murphy S, O'Brien F, Lyons A, et al. Commensal-Induced Regulatory T Cells Mediate Protection Against Pathogen-Stimulated Nf-Kappab Activation. PLos Pathog (2008) 4(8):e1000112. doi: 10.1371/journal.ppat.1000112
80. Barnes MJ, Powrie F. Regulatory T Cells Reinforce Intestinal Homeostasis. Immunity (2009) 31(3):401–11. doi: 10.1016/j.immuni.2009.08.011
81. Liu Z, Geboes K, Heremans H, Overbergh L, Mathieu C, Rutgeerts P, et al. Role of Interleukin-12 in the Induction of Mucosal Inflammation and Abrogation of Regulatory T Cell Function in Chronic Experimental Colitis. Eur J Immunol (2001) 31(5):1550–60. doi: 10.1002/1521-4141(200105)31:5<1550::Aid-immu1550>3.0.Co;2-3
82. Fortin G, Raymond M, Van VQ, Rubio M, Gautier P, Sarfati M, et al. A Role for Cd47 in the Development of Experimental Colitis Mediated by Sirpalpha+Cd103- Dendritic Cells. J Exp Med (2009) 206(9):1995–2011. doi: 10.1084/jem.20082805
83. Hasnain SZ, Tauro S, Das I, Tong H, Chen AC, Jeffery PL, et al. Il-10 Promotes Production of Intestinal Mucus by Suppressing Protein Misfolding and Endoplasmic Reticulum Stress in Goblet Cells. Gastroenterology (2013) 144(2):357–68.e9. doi: 10.1053/j.gastro.2012.10.043
84. Tsuji G, Takahara M, Uchi H, Matsuda T, Chiba T, Takeuchi S, et al. Identification of Ketoconazole as an Ahr-Nrf2 Activator in Cultured Human Keratinocytes: The Basis of Its Anti-Inflammatory Effect. J Invest Dermatol (2012) 132(1):59–68. doi: 10.1038/jid.2011.194
85. Zhang T, Kimura Y, Jiang S, Harada K, Yamashita Y, Ashida H. Luteolin Modulates Expression of Drug-Metabolizing Enzymes Through the Ahr and Nrf2 Pathways in Hepatic Cells. Arch Biochem biophysics (2014) 557:36–46. doi: 10.1016/j.abb.2014.05.023
86. Ruane DT, Lavelle EC. The Role of Cd103⁺ Dendritic Cells in the Intestinal Mucosal Immune System. Front Immunol (2011) 2:25. doi: 10.3389/fimmu.2011.00025
87. Mizoguchi A, Yano A, Himuro H, Ezaki Y, Sadanaga T, Mizoguchi E. Clinical Importance of Il-22 Cascade in Ibd. J Gastroenterol (2018) 53(4):465–74. doi: 10.1007/s00535-017-1401-7
88. Wolk K, Kunz S, Witte E, Friedrich M, Asadullah K, Sabat R. Il-22 Increases the Innate Immunity of Tissues. Immunity (2004) 21(2):241–54. doi: 10.1016/j.immuni.2004.07.007
89. Shen P, Roch T, Lampropoulou V, O'Connor RA, Stervbo U, Hilgenberg E, et al. Il-35-Producing B Cells Are Critical Regulators of Immunity During Autoimmune and Infectious Diseases. Nature (2014) 507(7492):366–70. doi: 10.1038/nature12979
90. Su LC, Liu XY, Huang AF, Xu WD. Emerging Role of Il-35 in Inflammatory Autoimmune Diseases. Autoimmun Rev (2018) 17(7):665–73. doi: 10.1016/j.autrev.2018.01.017
91. Meng D, Sommella E, Salviati E, Campiglia P, Ganguli K, Djebali K, et al. Indole-3-Lactic Acid, a Metabolite of Tryptophan, Secreted by Bifidobacterium Longum Subspecies Infantis Is Anti-Inflammatory in the Immature Intestine. Pediatr Res (2020) 88(2):209–17. doi: 10.1038/s41390-019-0740-x
92. Ledala N, Malik M, Rezaul K, Paveglio S, Provatas A, Kiel A, et al. Bacterial Indole as a Multifunctional Regulator of Klebsiella Oxytoca Complex Enterotoxicity. mBio (2022) 13(1):e0375221. doi: 10.1128/mbio.03752-21
93. Howard MF, Bina XR, Bina JE. Indole Inhibits Toxr Regulon Expression in Vibrio Cholerae. Infect Immun (2019) 87(3):e00776–18. doi: 10.1128/IAI.00776-18
94. Bommarius B, Anyanful A, Izrayelit Y, Bhatt S, Cartwright E, Wang W, et al. A Family of Indoles Regulate Virulence and Shiga Toxin Production in Pathogenic E. Coli. PLos One (2013) 8(1):e54456. doi: 10.1371/journal.pone.0054456
95. Lee J, Attila C, Cirillo SL, Cirillo JD, Wood TK. Indole and 7-Hydroxyindole Diminish Pseudomonas Aeruginosa Virulence. Microb Biotechnol (2009) 2(1):75–90. doi: 10.1111/j.1751-7915.2008.00061.x
96. Shimada Y, Kinoshita M, Harada K, Mizutani M, Masahata K, Kayama H, et al. Commensal Bacteria-Dependent Indole Production Enhances Epithelial Barrier Function in the Colon. PLos One (2013) 8(11):e80604. doi: 10.1371/journal.pone.0080604
97. Li J, Zhang L, Wu T, Li Y, Zhou X, Ruan Z. Indole-3-Propionic Acid Improved the Intestinal Barrier by Enhancing Epithelial Barrier and Mucus Barrier. J Agric Food Chem (2021) 69(5):1487–95. doi: 10.1021/acs.jafc.0c05205
98. Dong F, Perdew GH. The Aryl Hydrocarbon Receptor as a Mediator of Host-Microbiota Interplay. Gut Microbes (2020) 12(1):1859812. doi: 10.1080/19490976.2020.1859812
99. Kim CH. Immune Regulation by Microbiome Metabolites. Immunology (2018) 154(2):220–9. doi: 10.1111/imm.12930
100. Vyhlídalová B, Krasulová K, Pečinková P, Marcalíková A, Vrzal R, Zemánková L, et al. Gut Microbial Catabolites of Tryptophan Are Ligands and Agonists of the Aryl Hydrocarbon Receptor: A Detailed Characterization. Int J Mol Sci (2020) 21(7):2614. doi: 10.3390/ijms21072614
101. Scott SA, Fu J, Chang PV. Microbial Tryptophan Metabolites Regulate Gut Barrier Function Via the Aryl Hydrocarbon Receptor. Proc Natl Acad Sci U S A (2020) 117(32):19376–87. doi: 10.1073/pnas.2000047117
102. Lamas B, Natividad JM, Sokol H. Aryl Hydrocarbon Receptor and Intestinal Immunity. Mucosal Immunol (2018) 11(4):1024–38. doi: 10.1038/s41385-018-0019-2
103. Hubbard TD, Murray IA, Perdew GH. Indole and Tryptophan Metabolism: Endogenous and Dietary Routes to Ah Receptor Activation. Drug Metab Disposition: Biol Fate Chemicals (2015) 43(10):1522–35. doi: 10.1124/dmd.115.064246
104. Sun M, Ma N, He T, Johnston LJ, Ma X. Tryptophan (Trp) Modulates Gut Homeostasis Via Aryl Hydrocarbon Receptor (Ahr). Crit Rev Food Sci Nutr (2020) 60(10):1760–8. doi: 10.1080/10408398.2019.1598334
105. Grifka-Walk HM, Jenkins BR, Kominsky DJ. Amino Acid Trp: The Far Out Impacts of Host and Commensal Tryptophan Metabolism. Front Immunol (2021) 12:653208. doi: 10.3389/fimmu.2021.653208
106. Hasegawa M, Yada S, Liu MZ, Kamada N, Munoz-Planillo R, Do N, et al. Interleukin-22 Regulates the Complement System to Promote Resistance Against Pathobionts After Pathogen-Induced Intestinal Damage. Immunity (2014) 41(4):620–32. doi: 10.1016/j.immuni.2014.09.010
107. Koutsounas I, Theocharis S, Patsouris E, Giaginis C. Pregnane X Receptor (Pxr) at the Crossroads of Human Metabolism and Disease. Curr Drug Metab (2013) 14(3):341–50. doi: 10.2174/1389200211314030009
108. Huang K, Mukherjee S, DesMarais V, Albanese JM, Rafti E, Draghi Ii A, et al. Targeting the Pxr-Tlr4 Signaling Pathway to Reduce Intestinal Inflammation in an Experimental Model of Necrotizing Enterocolitis. Pediatr Res (2018) 83(5):1031–40. doi: 10.1038/pr.2018.14
109. Ranhotra HS, Flannigan KL, Brave M, Mukherjee S, Lukin DJ, Hirota SA, et al. Xenobiotic Receptor-Mediated Regulation of Intestinal Barrier Function and Innate Immunity. Nucl receptor Res (2016) 3:101199. doi: 10.11131/2016/101199
110. Illes P, Krasulova K, Vyhlidalova B, Poulikova K, Marcalikova A, Pecinkova P, et al. Indole Microbial Intestinal Metabolites Expand the Repertoire of Ligands and Agonists of the Human Pregnane X Receptor. Toxicol Lett (2020) 334:87–93. doi: 10.1016/j.toxlet.2020.09.015
111. Wang ZK, Yang YS, Chen Y, Yuan J, Sun G, Peng LH. Intestinal Microbiota Pathogenesis and Fecal Microbiota Transplantation for Inflammatory Bowel Disease. World J Gastroenterol (2014) 20(40):14805–20. doi: 10.3748/wjg.v20.i40.14805
112. Lavelle A, Sokol H. Gut Microbiota-Derived Metabolites as Key Actors in Inflammatory Bowel Disease. Nat Rev Gastroenterol Hepatol (2020) 17(4):223–37. doi: 10.1038/s41575-019-0258-z
113. Lamas B, Richard ML, Leducq V, Pham HP, Michel ML, Da Costa G, et al. Card9 Impacts Colitis by Altering Gut Microbiota Metabolism of Tryptophan Into Aryl Hydrocarbon Receptor Ligands. Nat Med (2016) 22(6):598–605. doi: 10.1038/nm.4102
114. Lee J, Bansal T, Jayaraman A, Bentley WE, Wood TK. Enterohemorrhagic Escherichia Coli Biofilms Are Inhibited by 7-Hydroxyindole and Stimulated by Isatin. Appl Environ Microbiol (2007) 73(13):4100–9. doi: 10.1128/aem.00360-07
115. Sun XZ, Zhao DY, Zhou YC, Wang QQ, Qin G, Yao SK. Alteration of Fecal Tryptophan Metabolism Correlates With Shifted Microbiota and May Be Involved in Pathogenesis of Colorectal Cancer. World J Gastroenterol (2020) 26(45):7173–90. doi: 10.3748/wjg.v26.i45.7173
116. Tourino MC, de Oliveira EM, Bellé LP, Knebel FH, Albuquerque RC, Dörr FA, et al. Tryptamine and Dimethyltryptamine Inhibit Indoleamine 2,3 Dioxygenase and Increase the Tumor-Reactive Effect of Peripheral Blood Mononuclear Cells. Cell Biochem Funct (2013) 31(5):361–4. doi: 10.1002/cbf.2980
117. Ferdinande L, Decaestecker C, Verset L, Mathieu A, Moles Lopez X, Negulescu AM, et al. Clinicopathological Significance of Indoleamine 2,3-Dioxygenase 1 Expression in Colorectal Cancer. Br J Cancer (2012) 106(1):141–7. doi: 10.1038/bjc.2011.513
118. Murphy AG, Zheng L. Small Molecule Drugs With Immunomodulatory Effects in Cancer. Hum Vaccines immunother (2015) 11(10):2463–8. doi: 10.1080/21645515.2015.1057363
119. Labadie BW, Bao R, Luke JJ. Reimagining Ido Pathway Inhibition in Cancer Immunotherapy Via Downstream Focus on the Tryptophan-Kynurenine-Aryl Hydrocarbon Axis. Clin Cancer Res (2019) 25(5):1462–71. doi: 10.1158/1078-0432.CCR-18-2882
120. Munn DH, Sharma MD, Lee JR, Jhaver KG, Johnson TS, Keskin DB, et al. Potential Regulatory Function of Human Dendritic Cells Expressing Indoleamine 2,3-Dioxygenase. Science (2002) 297(5588):1867–70. doi: 10.1126/science.1073514
121. Munn DH, Sharma MD, Mellor AL. Ligation of B7-1/B7-2 by Human Cd4+ T Cells Triggers Indoleamine 2,3-Dioxygenase Activity in Dendritic Cells. J Immunol (2004) 172(7):4100–10. doi: 10.4049/jimmunol.172.7.4100
122. Abildgaard A, Elfving B, Hokland M, Wegener G, Lund S. The Microbial Metabolite Indole-3-Propionic Acid Improves Glucose Metabolism in Rats, But Does Not Affect Behaviour. Arch Physiol Biochem (2018) 124(4):306–12. doi: 10.1080/13813455.2017.1398262
123. Holst JJ. The Physiology of Glucagon-Like Peptide 1. Physiol Rev (2007) 87(4):1409–39. doi: 10.1152/physrev.00034.2006
124. de Mello VD, Paananen J, Lindstrom J, Lankinen MA, Shi L, Kuusisto J, et al. Indolepropionic Acid and Novel Lipid Metabolites Are Associated With a Lower Risk of Type 2 Diabetes in the Finnish Diabetes Prevention Study. Sci Rep (2017) 7:46337. doi: 10.1038/srep46337
125. Rothhammer V, Mascanfroni ID, Bunse L, Takenaka MC, Kenison JE, Mayo L, et al. Type I Interferons and Microbial Metabolites of Tryptophan Modulate Astrocyte Activity and Central Nervous System Inflammation Via the Aryl Hydrocarbon Receptor. Nat Med (2016) 22(6):586–97. doi: 10.1038/nm.4106
126. Bosi A, Banfi D, Bistoletti M, Giaroni C, Baj A. Tryptophan Metabolites Along the Microbiota-Gut-Brain Axis: An Interkingdom Communication System Influencing the Gut in Health and Disease. Int J Tryptophan Res (2020) 13:1178646920928984. doi: 10.1177/1178646920928984
127. Pulakazhi Venu VK, Saifeddine M, Mihara K, Tsai YC, Nieves K, Alston L, et al. The Pregnane X Receptor and Its Microbiota-Derived Ligand Indole 3-Propionic Acid Regulate Endothelium-Dependent Vasodilation. Am J Physiol Endocrinol Metab (2019) 317(2):E350–E61. doi: 10.1152/ajpendo.00572.2018
128. Sallée M, Dou L, Cerini C, Poitevin S, Brunet P, Burtey S. The Aryl Hydrocarbon Receptor-Activating Effect of Uremic Toxins From Tryptophan Metabolism: A New Concept to Understand Cardiovascular Complications of Chronic Kidney Disease. Toxins (Basel) (2014) 6(3):934–49. doi: 10.3390/toxins6030934
129. Devlin AS, Marcobal A, Dodd D, Nayfach S, Plummer N, Meyer T, et al. Modulation of a Circulating Uremic Solute Via Rational Genetic Manipulation of the Gut Microbiota. Cell Host Microbe (2016) 20(6):709–15. doi: 10.1016/j.chom.2016.10.021
130. Shimizu H, Yisireyili M, Nishijima F, Niwa T. Indoxyl Sulfate Enhances P53-Tgf-β1-Smad3 Pathway in Proximal Tubular Cells. Am J Nephrol (2013) 37(2):97–103. doi: 10.1159/000346420
131. Bolati D, Shimizu H, Yisireyili M, Nishijima F, Niwa T. Indoxyl Sulfate, a Uremic Toxin, Downregulates Renal Expression of Nrf2 Through Activation of Nf-κb. BMC Nephrol (2013) 14:56. doi: 10.1186/1471-2369-14-56
132. Huang Y, Zhou J, Wang S, Xiong J, Chen Y, Liu Y, et al. Indoxyl Sulfate Induces Intestinal Barrier Injury Through Irf1-Drp1 Axis-Mediated Mitophagy Impairment. Theranostics (2020) 10(16):7384–400. doi: 10.7150/thno.45455
133. Adesso S, Ruocco M, Rapa SF, Piaz FD, Raffaele Di Iorio B, Popolo A, et al. Effect of Indoxyl Sulfate on the Repair and Intactness of Intestinal Epithelial Cells: Role of Reactive Oxygen Species' Release. Int J Mol Sci (2019) 20(9):2280. doi: 10.3390/ijms20092280
134. Rapa SF, Prisco F, Popolo A, Iovane V, Autore G, Di Iorio BR, et al. Pro-Inflammatory Effects of Indoxyl Sulfate in Mice: Impairment of Intestinal Homeostasis and Immune Response. Int J Mol Sci (2021) 22(3):1135. doi: 10.3390/ijms22031135
135. Hoibian E, Florens N, Koppe L, Vidal H, Soulage CO. Distal Colon Motor Dysfunction in Mice With Chronic Kidney Disease: Putative Role of Uremic Toxins. Toxins (Basel) (2018) 10(5):204. doi: 10.3390/toxins10050204
136. Sun CY, Lin CJ, Pan HC, Lee CC, Lu SC, Hsieh YT, et al. Clinical Association Between the Metabolite of Healthy Gut Microbiota, 3-Indolepropionic Acid and Chronic Kidney Disease. Clin Nutr (2019) 38(6):2945–8. doi: 10.1016/j.clnu.2018.11.029
137. Müting D. Studies on the Pathogenesis of Uremia. Comparative Determinations of Glucuronic Acid, Indican, Free and Bound Phenols in the Serum, Cerebrospinal Fluid, and Urine of Renal Diseases With and Without Uremia. Clinica chimica acta; Int J Clin Chem (1965) 12(5):551–4. doi: 10.1016/0009-8981(65)90171-3
138. Schoots AC, De Vries PM, Thiemann R, Hazejager WA, Visser SL, Oe PL. Biochemical and Neurophysiological Parameters in Hemodialyzed Patients With Chronic Renal Failure. Clinica Chimica Acta; Int J Clin Chem (1989) 185(1):91–107. doi: 10.1016/0009-8981(89)90134-4
139. Adesso S, Magnus T, Cuzzocrea S, Campolo M, Rissiek B, Paciello O, et al. Indoxyl Sulfate Affects Glial Function Increasing Oxidative Stress and Neuroinflammation in Chronic Kidney Disease: Interaction Between Astrocytes and Microglia. Front Pharmacol (2017) 8:370. doi: 10.3389/fphar.2017.00370
140. Adesso S, Paterniti I, Cuzzocrea S, Fujioka M, Autore G, Magnus T, et al. Ast-120 Reduces Neuroinflammation Induced by Indoxyl Sulfate in Glial Cells. J Clin Med (2018) 7(10):365. doi: 10.3390/jcm7100365
141. Sun CY, Li JR, Wang YY, Lin SY, Ou YC, Lin CJ, et al. Indoxyl Sulfate Caused Behavioral Abnormality and Neurodegeneration in Mice With Unilateral Nephrectomy. Aging (Albany NY) (2021) 13(5):6681–701. doi: 10.18632/aging.202523
142. Gao H, Liu S. Role of Uremic Toxin Indoxyl Sulfate in the Progression of Cardiovascular Disease. Life Sci (2017) 185:23–9. doi: 10.1016/j.lfs.2017.07.027
143. Falconi CA, Junho C, Fogaça-Ruiz F, Vernier ICS, da Cunha RS, Stinghen AEM, et al. Uremic Toxins: An Alarming Danger Concerning the Cardiovascular System. Front Physiol (2021) 12:686249. doi: 10.3389/fphys.2021.686249
144. Wu PH, Lin YT, Chiu YW, Baldanzi G, Huang JC, Liang SS, et al. The Relationship of Indoxyl Sulfate and P-Cresyl Sulfate With Target Cardiovascular Proteins in Hemodialysis Patients. Sci Rep (2021) 11(1):3786. doi: 10.1038/s41598-021-83383-x
145. Yang K, Du C, Wang X, Li F, Xu Y, Wang S, et al. Indoxyl Sulfate Induces Platelet Hyperactivity and Contributes to Chronic Kidney Disease-Associated Thrombosis in Mice. Blood (2017) 129(19):2667–79. doi: 10.1182/blood-2016-10-744060
146. Addi T, Dou L, Burtey S. Tryptophan-Derived Uremic Toxins and Thrombosis in Chronic Kidney Disease. Toxins (2018) 10(10):412. doi: 10.3390/toxins10100412
147. Yisireyili M, Shimizu H, Saito S, Enomoto A, Nishijima F, Niwa T. Indoxyl Sulfate Promotes Cardiac Fibrosis With Enhanced Oxidative Stress in Hypertensive Rats. Life Sci (2013) 92(24-26):1180–5. doi: 10.1016/j.lfs.2013.05.008
148. Niwa T. Indoxyl Sulfate Is a Nephro-Vascular Toxin. J Ren Nutr (2010) 20(5 Suppl):S2–6. doi: 10.1053/j.jrn.2010.05.002
149. Sun CY, Chang SC, Wu MS. Suppression of Klotho Expression by Protein-Bound Uremic Toxins Is Associated With Increased DNA Methyltransferase Expression and DNA Hypermethylation. Kidney Int (2012) 81(7):640–50. doi: 10.1038/ki.2011.445
150. Sun CY, Hsu HH, Wu MS. P-Cresol Sulfate and Indoxyl Sulfate Induce Similar Cellular Inflammatory Gene Expressions in Cultured Proximal Renal Tubular Cells. Nephrol Dialysis Transplant Off Publ Eur Dialysis Transplant Assoc - Eur Renal Assoc (2013) 28(1):70–8. doi: 10.1093/ndt/gfs133
151. Bolati D, Shimizu H, Higashiyama Y, Nishijima F, Niwa T. Indoxyl Sulfate Induces Epithelial-To-Mesenchymal Transition in Rat Kidneys and Human Proximal Tubular Cells. Am J Nephrol (2011) 34(4):318–23. doi: 10.1159/000330852
152. Miyazaki T, Ise M, Hirata M, Endo K, Ito Y, Seo H, et al. Indoxyl Sulfate Stimulates Renal Synthesis of Transforming Growth Factor-Beta 1 and Progression of Renal Failure. Kidney Int Supplement (1997) 63:S211–4.
153. Sirich TL, Aronov PA, Plummer NS, Hostetter TH, Meyer TW. Numerous Protein-Bound Solutes Are Cleared by the Kidney With High Efficiency. Kidney Int (2013) 84(3):585–90. doi: 10.1038/ki.2013.154
154. Gulyassy PF, Jarrard E, Stanfel LA. Contributions of Hippurate, Indoxyl Sulfate, and O-Hydroxyhippurate to Impaired Ligand Binding by Plasma in Azotemic Humans. Biochem Pharmacol (1987) 36(24):4215–20. doi: 10.1016/0006-2952(87)90661-7
155. Bowmer CJ, Lindup WE. Decreased Drug Binding in Uraemia: Effect of Indoxyl Sulphate and Other Endogenous Substances on the Binding of Drugs and Dyes to Human Albumin. Biochem Pharmacol (1982) 31(3):319–23. doi: 10.1016/0006-2952(82)90177-0
156. Niwa T, Takeda N, Tatematsu A, Maeda K. Accumulation of Indoxyl Sulfate, an Inhibitor of Drug-Binding, in Uremic Serum as Demonstrated by Internal-Surface Reversed-Phase Liquid Chromatography. Clin Chem (1988) 34(11):2264–7. doi: 10.1093/clinchem/34.11.2264
157. Leong SC, Sirich TL. Indoxyl Sulfate-Review of Toxicity and Therapeutic Strategies. Toxins (Basel) (2016) 8(12):358. doi: 0.3390/toxins8120358
Keywords: indole, inflammation, intestinal flora, indoxyl sulfate, dual role
Citation: Ye X, Li H, Anjum K, Zhong X, Miao S, Zheng G, Liu W and Li L (2022) Dual Role of Indoles Derived From Intestinal Microbiota on Human Health. Front. Immunol. 13:903526. doi: 10.3389/fimmu.2022.903526
Received: 24 March 2022; Accepted: 23 May 2022;
Published: 17 June 2022.
Edited by:
Qixiao Zhai, Jiangnan University, ChinaReviewed by:
Tingtao Chen, Nanchang University, ChinaTeresa Zelante, University of Perugia, Italy
Jiachao Zhang, Hainan University, China
Copyright © 2022 Ye, Li, Anjum, Zhong, Miao, Zheng, Liu and Li. This is an open-access article distributed under the terms of the Creative Commons Attribution License (CC BY). The use, distribution or reproduction in other forums is permitted, provided the original author(s) and the copyright owner(s) are credited and that the original publication in this journal is cited, in accordance with accepted academic practice. No use, distribution or reproduction is permitted which does not comply with these terms.
*Correspondence: Lanjuan Li, ljli@zju.edu.cn; Wei Liu, biolwei@sina.com
†These authors have contributed equally to this work. The author order was determined by their equal but gradated contributions for this paper