- Department of Gynecology and Obstetrics, Key Laboratory of Obstetrics & Gynecologic and Pediatric Diseases and Birth Defects of Ministry of Education, Development and Related Diseases of Women and Children Key Laboratory of Sichuan Province, West China Second Hospital, Sichuan University, Chengdu, China
Ovarian cancer is the most lethal gynecologic tumor, with the highest mortality rate. Numerous studies have been conducted on the treatment of ovarian cancer in the hopes of improving therapeutic outcomes. Immune cells have been revealed to play a dual function in the development of ovarian cancer, acting as both tumor promoters and tumor suppressors. Increasingly, the tumor immune microenvironment (TIME) has been proposed and confirmed to play a unique role in tumor development and treatment by altering immunosuppressive and cytotoxic responses in the vicinity of tumor cells through metabolic reprogramming. Furthermore, studies of immunometabolism have provided new insights into the understanding of the TIME. Targeting or activating metabolic processes of the TIME has the potential to be an antitumor therapy modality. In this review, we summarize the composition of the TIME of ovarian cancer and its metabolic reprogramming, its relationship with drug resistance in ovarian cancer, and recent research advances in immunotherapy.
1 Introduction
Ovarian cancer (OC) is the most lethal gynecological neoplasm due to its high mortality (1–3). Due to inadequate early detection methods and few early symptoms, ovarian cancer is the most challenging disease to identify in the female reproductive system (4). The majority of patients are frequently identified at an advanced stage, while therapeutic interventions are relatively limited. Only approximately 16% of patients are diagnosed at an early stage (FIGO I stage) and have a greater possibility of long-term survival (5, 6). The treatment of OC varies depending on its stage and classification (7). OC has an epithelial origin in more than 90% of cases, and high-grade serous ovarian cancer (HGSOC) makes up 70% of these cases (1, 8). According to the National Comprehensive Cancer Network (NCCN) Guidelines, debulking surgery combined with platinum-based chemotherapy is currently the first line of treatment for OC (7). The mortality of OC has decreased globally over the past decade as a result of lifestyle modifications and technological advancements in treatment (9). However, research has shown that early screening does not appear to reduce mortality from OC (10). More frustratingly, regardless of the type of treatment used, approximately 80% of cases of advanced ovarian cancer eventually recur and result in the progression of the disease and death (11). Given these findings, it is imperative to develop novel and reliable therapies and prevent recurrence.
Immune cells are a cluster of different cells that have differentiated from bone marrow hematopoietic stem cells (12). In tumors, immune cells have a dual role, either killing or promoting them (13). Immune cells can even become accomplices of tumor cells in the event of immune escape (14). In this regard, Stephen Paget put forth the well-known “seed and soil” hypothesis, according to which the environment in which a tumor develops determines how that tumor expresses its phenotype (15). The tumor immune microenvironment (TIME) refers to the immune infiltrative microenvironment, which consists of a large number of immune cells clustered within and around the tumor (16). With the advent of high-throughput and single-cell sequencing, research on the TIME has been fueled.
Immunotherapy is the next generation of treatment that is rapidly developing after traditional treatments such as surgery, radiotherapy, and chemotherapy (17). It functions by activating the immune defenses of the human body to eliminate tumor cells (17). A seemingly endless stream of cancer immunotherapies derived from tumor immunity have been developed, including immune checkpoint inhibitors (ICIs), adoptive cellular immunotherapy, tumor vaccines and others (17–19). Among these, ICIs have made significant progress and demonstrated excellent antitumor activity in gynecological cancer (20). However, not all patients with OC are candidates for immunotherapy, and only a small percentage of them will benefit (21, 22). Due to its cool tumor nature, OC has few infiltrating lymphocytes and responds poorly to immunotherapy (22, 23). Indubitably, OC is an immunogenic disease, and its immunogenicity is solely dependent on approximately 13% of CD8+ tumor-infiltrating T cells with a high affinity for antigens (24). Most likely, the underlying cause of drug resistance and treatment failure is metabolic reprogramming (25). Metabolic reprogramming, a hallmark of cancer, is the reprogramming of specific metabolic pathways inside and outside the cell to meet the demands of rapid proliferation by affecting gene expression, cellular state, and the tumor microenvironment (26). It may be crucial to investigate metabolic reprogramming of the TIME to increase therapeutic effectiveness and improve drug resistance in OC. This review focuses on the TIME in OC with an emphasis on its metabolic reprogramming and concludes with immunotherapy related to TIME metabolic reprogramming, with the goal of providing a new vision for immunotherapy in OC.
2 Overview of TIME metabolism in ovarian cancer
Tumors have a complicated metabolic pattern that is a highly adaptable response to hypoxia and nutritional deficiency (25). They have the ability to select the optimal metabolic phenotype based on the microenvironment. The metabolic overview of the ovarian cancer TIME is a network of substance exchange incorporating biochemical reactions. The metabolism of both tumor cells and immune cells is briefly discussed next.
2.1 Metabolism of ovarian cancer cells
Ovarian cancer cells have a heterogeneous metabolism, which means they are able to modify their metabolic patterns in response to their microenvironment (Figure 1A). The metabolic characteristics of ovarian cancer cells can be described as prominent glucose metabolism and lipid metabolism (Figure 1B).
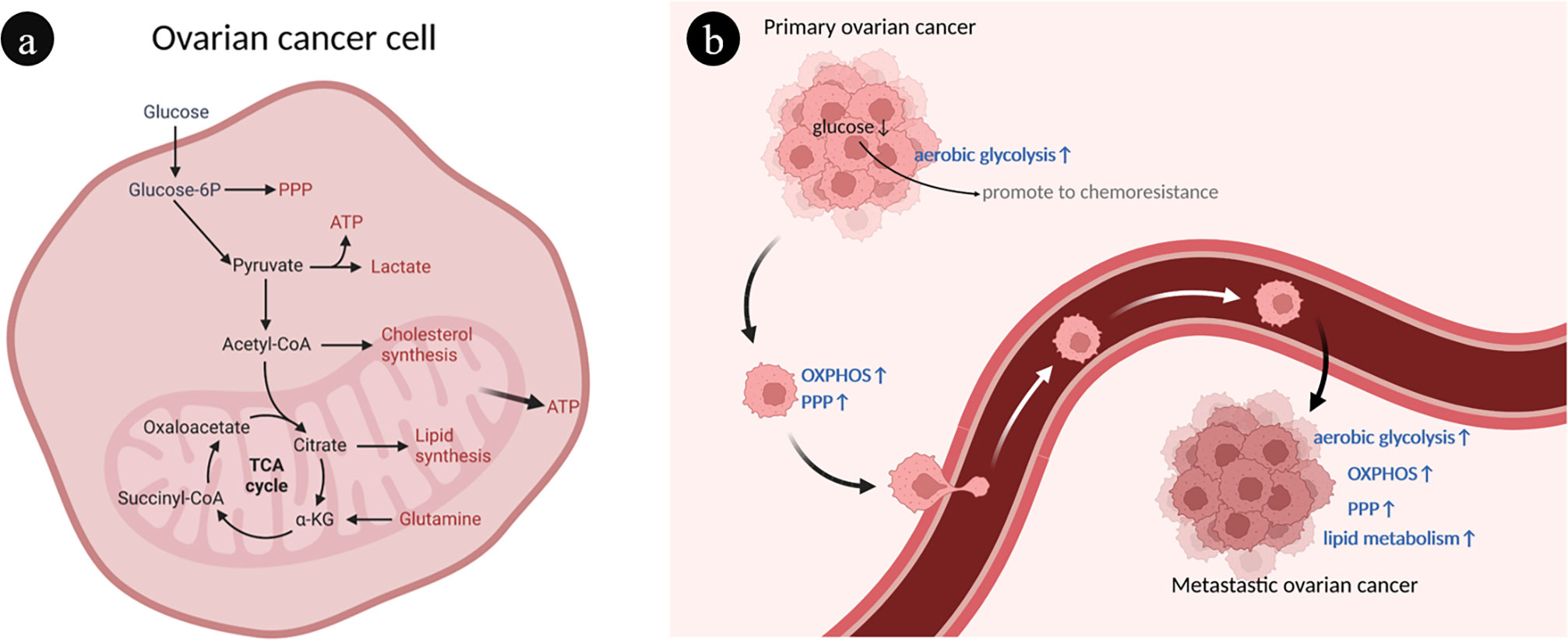
Figure 1 Overview of ovarian cancer cell metabolism. (A) Interaction of different metabolic pathways within ovarian cancer cells. There are connections between different metabolisms through metabolites. (B) The heterogeneity of ovarian cancer metabolism is characterized by abnormal glucose and lipid metabolism. During the development of ovarian cancer, different metabolic phenotypes are gradually acquired, such as enhanced OXPHOS, PPP and FAO. ATP, Adenosine triphosphate; FAO, Fatty acid oxidation; OXPHOS, Oxidative phosphorylation; PPP, Pentose phosphate pathway; TCA, Tricarboxylic acid.
The selection of aerobic glycolysis and oxidative phosphorylation as energy sources is a marked manifestation of the heterogeneity of ovarian cancer (27). Through aerobic glycolysis, often known as the Warburg effect, ovarian cancer cells primarily consume glucose to obtain energy (28). Ovarian cancer tumor cells use a substantial quantity of glucose during this process, which is finally generated and expelled as lactic acid, resulting in a localized hypoxic, hypoglycemic, and acidic microenvironment (29). The local low-glucose environment contributes to the expansion of chemical resistance to paclitaxel in ovarian cancer cells, creating a vicious cycle (30). Mitochondrial oxidative phosphorylation (OXPHOS) and the pentose phosphate pathway (PPP) were upregulated in ovarian cancer cells with metastatic capacity (31, 32). Glutamate is used by ovarian cancer cells in mitochondrial OXPHOS to produce adenosine triphosphate (ATP) and other biomolecules necessary for aggressive expansion and migration (33). Platinum-based chemotherapy increases mitochondrial OXPHOS activity of ovarian cancer cells but contributes to cancer stem cell enrichment (34).
Another characteristic of ovarian cancer cells is abnormal active lipid metabolism, which is intimately associated with tumor progression and metastasis (35). The preferential metastasis of ovarian cancer to lipid-rich tissues such as the omentum suggests that adipocyte-derived fatty acids are a significant source of energy for the rapid spreading and metastasis of ovarian cancer cells (36, 37).
2.2 Metabolism of immune cells in ovarian cancer
TIME has tissue heterogeneity, which means that the type and function of immune cells entering the microenvironment in various cancer types differs substantially. There is a certain gap between the resting and activated states of immune cells in ovarian cancer, as well as between different stages of tumor development. Tumor-infiltrating immune cells are an essential component of the TIME and can be classified as pro-tumor cells (tumor-associated macrophages and myeloid-derived suppressor cells) and antitumor cells (such as effector T cells and natural killer cells). The proportion and distribution of immune cells are crucial for the development and spread of tumors. Table 1 provides a summary of immune cell metabolic propensity in the ovarian cancer TIME.
2.2.1 Natural killer (NK) cells
Natural killer (NK) cells can be cytotoxic as well as immunomodulatory (38, 39). The primary mechanism by which NK cells attack ovarian cancer cells is antibody-dependent cellular cytotoxicity (52). The activation of NK cells depends on aerobic glycolysis (53, 54).
2.2.2 Tumor-associated macrophages (TAMs)
Tumor-associated macrophages (TAMs) are significantly infiltrated in ovarian cancer, and the extent of their polarization is tightly tied to the local microenvironment (55). Under different conditions, TAMs can polarize into two types: M1-TAMs (proinflammatory/antitumor) and M2-TAMs (anti-inflammatory/protumor) (56). The expression of both M1 and M2 markers was elevated in ovarian cancer according to genome-wide expression profiling of TAMs (57). From this, it may be inferred that the monocyte phenotype of ovarian cancer is mixed polarization. The aggressiveness of ovarian cancer is directly related to the degree of infiltration of M2-TAMs. In ovarian cancer, a high M2/M1 ratio indicates a poor prognosis (58). Typically, M1-TAMs rely on aerobic glycolysis, whereas M2-TAMs are more dependent on OXPHOS (40, 41).
2.2.3 T cells
T cells can be classified into a number of subtypes based on their varied roles, and these subtypes also differ in their corresponding metabolic patterns. When naive T cells differentiate into effector T cells (Teffs) in tumors, they switch from relying mostly on OXPHOS for energy to relying primarily on glycolysis (42). Memory T cells (Tmems) show high OXPHOS levels (42, 43). In particular, regulatory T lymphocytes (Tregs) have an immunosuppressive function in tumors, where their recruitment contributes to the immune escape of ovarian cancer (59). Tregs have higher levels of glucose uptake and glycolysis because their genes associated with glucose metabolism are substantially expressed (46). A high glycolytic level of CD4+ Tregs was likewise found in coculture with SKOV3 ovarian cancer cells (46). In addition, Tregs can also rely on OXPHOS produced by fatty acid oxidation (FAO) to provide energy (60).
2.2.4 Dendritic cells (DCs)
Dendritic cells are antigen-presenting cells that act as a bridge between innate and cellular immunity. Resting immature DCs primarily utilize mitochondrial β-oxidation of lipids and OXPHOS for energy (44). Glycolytic reprogramming takes place when DCs are activated (44). Early glycolytic reprogramming is supported by glycogen metabolism in DCs (45). The TIME inhibits the function of DCs in malignancies. In the ID8 ovarian cancer mouse model, tumor cells led to overexpression of suppressor of cytokine signaling 3 (SOCS3) in DCs and lowered the activity of the corresponding pyruvate kinase (a crucial enzyme for glycolysis), which inhibited the function of DCs (61).
2.2.5 Myeloid-derived suppressor cells (MDSCs)
A group of immature heterogeneous cells from the bone marrow known as myeloid-derived suppressor cells (MDSCs) have immunosuppressive properties (62). It has been proven that enhanced lipid uptake and FAO by MDSCs activate immunosuppressive mechanisms (48). OXPHOS (fueled by glutamine) and tricarboxylic acid (TCA) cycling were elevated in MDSCs, which were demonstrated to have a high-energy metabolic profile in the ID8 ovarian cancer mouse model (49). Additionally, arginine metabolism is of great importance in MDSCs. MDSCs can regulate T cell function by removing essential metabolites such as arginine from the microenvironment (50). MDSCs were found to be dependent on arginase-1 (ARG1) to exert their T cell immunosuppressive phenotype (51).
The metabolism of immune cells in ovarian cancer is heterogeneous. Immune-activated cells are more dependent on anaerobic glycolysis, whereas immunosuppressive cells tend to use multiple metabolic pathways, including OXPHOS, to meet their energy needs. Modulation and intervention of immune cell metabolic patterns can aid in breaking the immunosuppressive TIME of ovarian cancer.
2.3 Overall metabolism of ovarian cancer patients
Compared to healthy individuals, patients with OC have a generally altered metabolism. The analysis of serum metabolomic data from epithelial ovarian cancer (EOC) revealed considerable heterogeneity in the metabolism of distinct subtypes of ovarian cancer (63). This demonstrates the metabolic heterogeneity of various subtypes in ovarian cancer. For instance, Hishinuma, E., et al. found an increase in tryptophan and its metabolite kynurenine by a wide-target metabolomic analysis of plasma from patients with epithelial ovarian cancer, and a larger ratio in its proportion was associated with a worse prognosis (57). The metabolism of ovarian clear cell carcinoma (OCCC), on the other hand, shows increased expression of glycolysis and oxidative stress genes, as well as improved mitochondrial OXPHOS and glycolysis (64). Additionally, the oncogene ARID1A has loss-of-function mutations in more than 50% of OCCC patients, which leads to decreased glutaminase (GLS) inhibition (65). This consequently contributes to a particular metabolic need for glutamine in OCCC.
Furthermore, metabolism differs between stages of ovarian cancer. Altered metabolism occurs even in the early stages of ovarian cancer. High-accuracy metabolomics detection of early-stage ovarian cancer indicated increased manufacture of fatty acids and cholesterol, as well as increased expression of enzymes that block FAO (66).
3 Metabolic reprogramming of the TIME in ovarian cancer based on various factors
How the metabolic reprogramming of the TIME in ovarian cancer occurs is one of the hot topics of research, and multiple factors are now known to influence it. Tumor-derived cytokines, chemokines, and even the metabolic microenvironment (pH, oxygen levels, and nutrition) can have an impact on the metabolism and function of immune cells (29). Metabolic reprogramming is used to carry out the procedure. Here, we will summarize the metabolic reprogramming of the TIME in ovarian cancer in terms of different common metabolic pathways. Finally, the effect of metabolic signaling pathways on TIME reprogramming is added.
3.1 Glucose metabolism
Lactate is a necessary byproduct of glycolysis, and tumor cells in the TIME are extremely dependent on glycolytic capacity, excreting large amounts of lactate, resulting in a localized low glucose, low oxygen, and acidic environment. Kumagai, S., et al. found that in a highly glycolytic tumor microenvironment, Tregs actively take up lactate through monocarboxylate cotransporter 1 (MCT1) and enhance the expression of programmed death receptor 1 (PD-1) by promoting NFAT1 entry into the nucleus (67). However, PD-1 expression was reduced in Teffs (67). As a result, PD-1 treatment was doomed to fail. Furthermore, increased lactate in ovarian cancer promotes the production of VEGF, a potent inducer of angiogenesis in tumors (68). The ensuing cellular invasion is a critical element in the proliferation and metastasis of ovarian cancer. In conjunction with GM-CSF and M-CSF, tumor-derived lactic acidosis in ovarian cancer was discovered to drive macrophage differentiation into a preinflammatory tumor phenotype (VEGFhigh CXCL8+ IL1β+) (69). Furthermore, sphingosine kinase-1 in A2780 ovarian cancer cells is involved in the induction of aerobic glycolysis (70). This was demonstrated by an increase in lactate levels and expression of the MCT1, as well as a decrease in TCA cycle intermediate accumulation and the production of carbon dioxide (70). A recent study by I. Elia et al. found that tumor-derived lactate could redirect glucose metabolism in CD8+ T cells. Specifically, it induced a shift in pyruvate carboxylase activity to pyruvate dehydrogenase (a key enzyme of gluconeogenesis) catalytic reaction, leading to a reduction in the back-supplementation pathway of the TCA cycle, thereby suppressing tumor immunity (71).
Several enzymes involved in glucose metabolism have been implicated in the development of the malignant phenotype of ovarian cancer. Pyruvate kinase is the glycolysis rate-limiting enzyme that catalyzes the conversion of phosphoenolpyruvate and ADP to pyruvate and ATP (72). M-type pyruvate kinase is supportive of anabolic metabolism in cancers, with PKM1 and PKM2 isoforms. Normally proliferating cells are dominated by the high-activity tetrameric form of PKM2, while the low-activity dimeric form is primarily seen in cancer cells (72). TBC1D8 binds to PKM2 via its Rab-GAP TBC domain in invasive ovarian cancer cells, preventing PKM2 tetramerization (73). This leads to a decrease in pyruvate kinase activity, promotes aerobic glycolysis and nuclear translocation of PKM2, and induces activation of glucose metabolism and cell cycle-related genes (73). In contrast, SOCS3, which has been shown to be increased in DCs by tumor-derived substances, interacts with PKM2, resulting in decreased ATP generation under hypoxic conditions and a profound effect on the function of DCs (61). Follicle-stimulating hormone (FSH) was found to upregulate the expression of PKM2 and glycolysis in SKOV3 and OVCAR3 ovarian cancer cells in an in vitro assay (74).
Glyceraldehyde-3-phosphate dehydrogenase (GAPDH), a glycolytic enzyme, controls IFN-γ production in Teffs by binding to AU-rich elements within the 3’ UTR of IFN-γ mRNA (75). As a result, the ability of activated Teffs to produce IFN-γ is severely hampered when they are prohibited from participating in glycolysis even under suitable conditions (75).
In mitochondria, pyruvate is decarboxylated by pyruvate dehydrogenase kinase 1 (PDK1) to generate acetyl coenzyme A (76). It is heavily expressed in ovarian cancer and serves as a crucial hub between glycolysis and the TCA cycle (77). Inhibition of PDK1 could reverse the Warburg effect by switching cytoplasmic glucose metabolism to mitochondrial OXPHOS (78, 79). In ovarian cancer cells, abnormal elevation of PDK1 can upregulate programmed death ligand-1 (PD-L1) expression and induce increased apoptosis of CD8+ T cells, ultimately impairing T cell immune function (80). Pyruvate dehydrogenase kinase 2 (PDK2) is favorably connected with the prognosis of OCCC, and increased PDK2 expression decreases apoptosis, resulting in cisplatin resistance in OCCC (81). By increasing the production of reactive oxygen species although mitochondrial metabolism, PDK2 inhibition can synergistically boost sensitivity to cisplatin (81). Another classical metabolic reprogramming occurs in the TCA cycle of macrophages. Macrophages are transferred from the TCA cycle and transform cis-aconitate to itaconic acid by the activity of aconitic acid decarboxylase 1 in the TIME of ovarian cancer (82). Itaconic acid, a metabolite with anti-inflammatory activity, can exert an inhibitory effect on glycolysis (83). A brief schematic of the reprogramming of glycolytic metabolism in ovarian cancer TIME is shown in Figure 2.
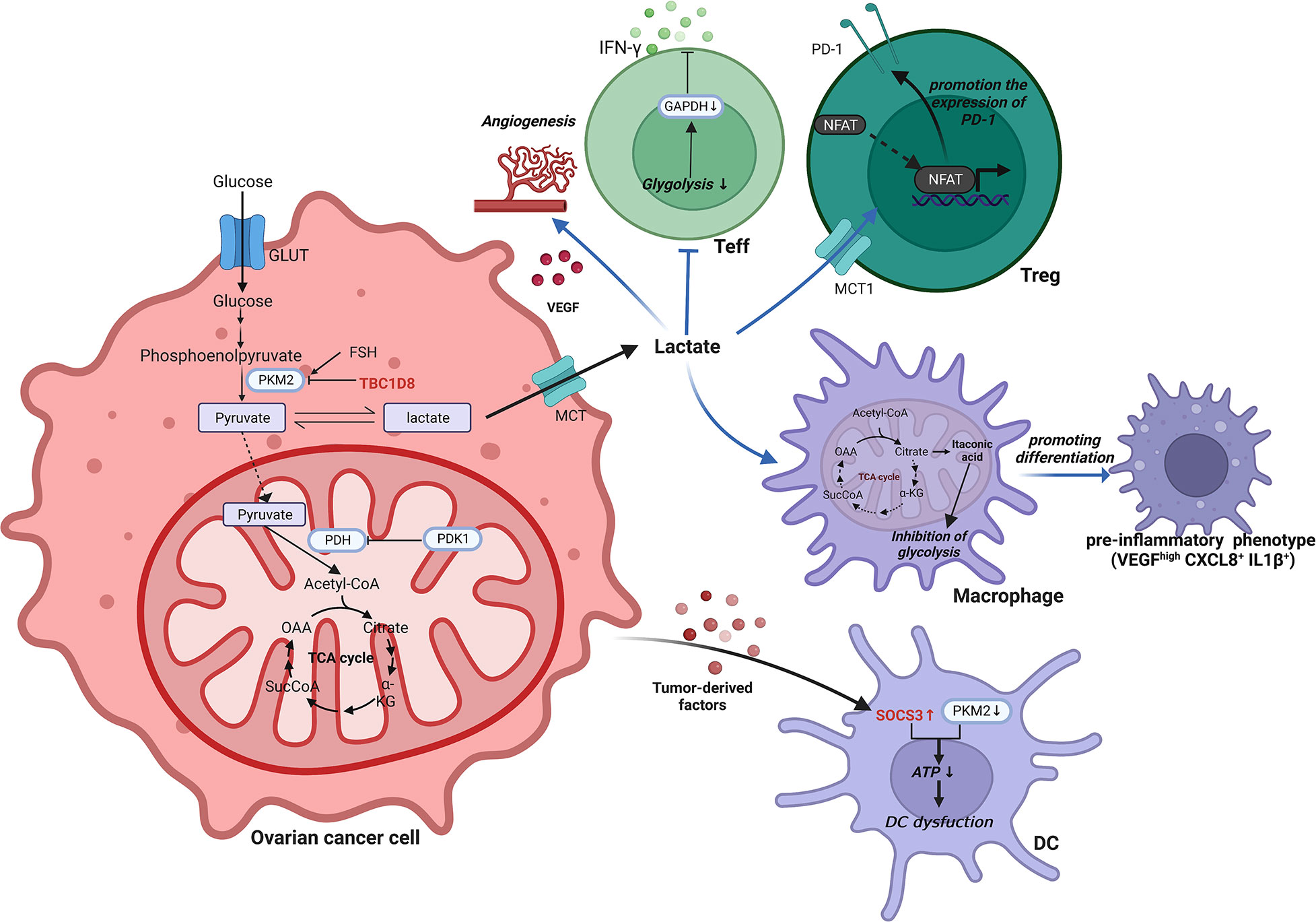
Figure 2 Reprogramming of glycolytic metabolism in the TIME of ovarian cancer. In the TIME of ovarian cancer, the reprogramming of glycolytic metabolism occurs in cells such as ovarian cancer cells, T cells, macrophages and DCs, resulting in a suppressive immune microenvironment that facilitates tumorigenesis and progression. ATP, Adenosine triphosphate; CXCL, C-X-C motif chemokine ligand; DC, Dendritic cell; FSH, Follicle-stimulating hormone; GAPDH, Glyceraldehyde-3-phosphate dehydrogenase; GLUT, Glucose transporter; IL, Interleukin; INF-γ, interferon-γ; MCT, Monocarboxylate cotransporter; NFAT, Nuclear factor of activated T cells; OAA, Oxalacetate; PDH, pyruvate dehydrogenase; PDK1, Pyruvate dehydrogenase kinase 1; PD-1, Programmed death receptor 1; PKM2, Pyruvate kinase M2; SOCS3, Cytokine signaling 3; TBC1D8, TBC1 domain family member 8; TCA, Tricarboxylic acid; Teff, effector T cell; Treg, Regulatory T cell; VEGF, Vascular endothelial growth factor.
3.2 Lipid metabolism
Reprogramming of ovarian cancer cells toward lipid metabolism is the initiating step for metastasis to the peritoneal cavity (84). Ovarian cancer cells can take up exogenous fatty acids (FAs) to promote dissemination in the peritoneal cavity. CD36 (FA receptor) is upregulated in metastatic human ovarian tumors (85). It was shown that ovarian cancer cells that were cocultured with primary human omental adipocytes underwent lipid metabolic reprogramming in the plasma membrane and expressed high levels of CD36 (85). Lipidomic analysis demonstrated that the high levels of polyunsaturated fatty acids, particularly linoleic acid, in ovarian cancer contributed to the tumor-promoting activity of TAMs as an efficient PPARβ/δ agonist (86).
Lysophosphatidic acid (LPA) is an important intermediate in glycerophospholipid metabolism. Through the transcriptional activation of VEGF, stimulation of Fas translocation, and other mechanisms, LPA in ovarian cancer ascites can promote tumor invasion, metastasis, and immune evasion (87, 88). TAMs were found to be a significant source of LPA in ovarian cancer by metabolomics (89). In T lymphoma cells, LPA was found to mediate apoptosis and glucose metabolism, supporting tumor cell survival (90). The corresponding metabolic regulation has yet to be demonstrated in ovarian cancer.
Prostaglandin E-2 (PGE2) is a hormone-like lipid metabolite generated by arachidonic acid via cyclooxygenase catalysis (91). Ovarian cancer can enhance proliferation and invasion through the PGE2/nuclear factor-kappa B signaling pathway (92). At the same time, tumor-derived PGE2 controls the production of CXCL12 and CXCR4, thereby inducing the migration of MDSCs to ascites (93, 94). In contrast, MDSC-derived PGE2 not only increases the stem cell-like properties of EOC but also increases PD-L1 expression in tumor cells (95).
3.3 Amino acid metabolism
The metabolism of amino acids has also been dramatically altered in ovarian cancer TIME to accommodate rapid growth. Figure 3 illustrates how amino acid metabolites connect various cells. This entire process entails reprogramming glutamine, arginine, tryptophan, aspartate, and one-carbon metabolism. The details are discussed as follows.
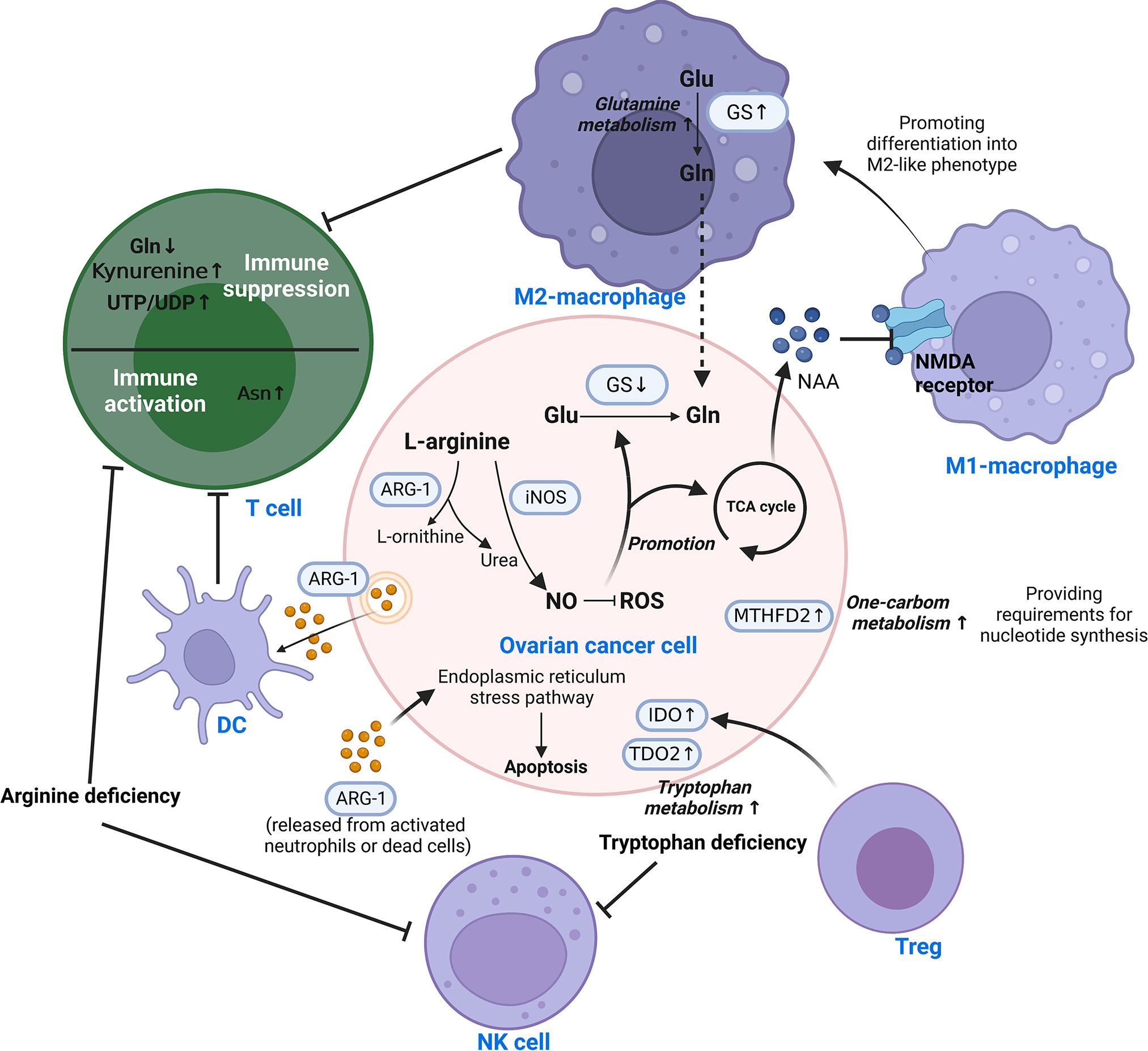
Figure 3 Reprogramming of amino acid metabolism in the TIME of ovarian cancer. Amino acid metabolism of ovarian cancer cells and immune cells in TIME is complementarily enhanced by metabolic reprogramming, which is responsible for mediating tumor progression and immune escape. Different cells are interconnected by amino acid metabolites. This involves the reprogramming of glutamine, arginine, tryptophan, aspartate, and one-carbon metabolism. Asn, Asparagine; ARG-1, Arginase-1; DC, Dendritic cell; Glu, Glutamate; Gln, Glutamine; GS, Glutamine synthetase; IDO, Indoleamine 2,3-dioxygenase; iNOS/NOS2, Nitric oxide synthase-2; ROS, Reactive oxygen species; TCA, Tricarboxylic acid; TDO2, tryptophan 2,3-dioxygenase; Teff, effector T cell; Treg, Regulatory T cell; MTHFD2, Methylenetetrahydrofolate dehydrogenase 2; NAA, N-acetylaspartate; NAMD receptor, N-methyl-D-aspartate receptor; NK, Natural killer; NO, nitric oxide; UDP, Uridine Diphosphate; UTP, Uridine Triphosphate.
3.3.1 Glutamine
Glutamine is a significant nutrient source for the development of tumor cells (96). Its metabolism is significantly associated with the aggressiveness of OC, where glutamine synthetase (GS) is silenced in ovarian cancer cells in favor of extracellular glutamine addiction (96, 97). In highly invasive ovarian cancer, macrophages were found to be driven toward the M2-like subtype by glutamine metabolism (98). N-acetylaspartate (NAA) is secreted by ovarian cancer cells as an antagonist to suppress NMDA receptors on macrophages, causing macrophages to assume an M2-like phenotype with increased GS expression (98). Malignant ascites in ovarian cancer patients have reduced glucose uptake, decreased mitochondrial activity and downregulated glutamine carrier abundance in T cells (99, 100). This leads to poor T cell mitochondrial function and evasion of immunity under low glucose conditions.
3.3.2 Arginine
Arginase converts L-arginine to L-ornithine and urea, which is known as the urea cycle. In the early stages of ovarian cancer, arginine metabolism is essential for the activation of T cells and control of immunological responses. In animal experiments, it was discovered that ovarian cancer cells express and release extracellular vesicles (EVs) containing ARG-1, which are taken up by DCs and prevent the proliferation of T cells (101). In contrast, ARG-1 released from activated neutrophils or dead cells induced apoptosis of cancer cells via the endoplasmic reticulum stress pathway (102). High amounts of arginine-1 boosted arginine metabolism in Tim-4+ TAMs (refilled from circulating monocytes), which in turn improved mitochondrial phagocytic activity in TAMs and ultimately inhibited T cell function (103). So do MDSCs. Strong arginine-1 expression and the production of ROS by MDSCs in human and mouse peritoneal ovarian cancers contribute to the immunosuppression of T cells (51). Arginine deficiency not only causes T cell malfunction but also decreases NK cell survival and cytotoxicity. In vitro experiments demonstrated that low L-Arg concentrations reduced the expression of activating receptors, NKp46 and NKp30, as well as the development of the NK zeta chain and the generation of IFN-gamma in NK-92 cell lines (104).
The production of nitric oxide (NO) by nitric oxide synthase is another catabolic mode of arginine. By raising NADPH and glutathione levels, NO lowers ROS levels and promotes glutamine and TCA cycling in ovarian cancer cells (105). Th17 cell growth is aided by physiological NO concentrations produced by MDSCs from ovarian cancer patients (106). Nitric oxide synthase 1 (NOS1) modulates S-nitrosylation at Cys351 of PFKM, which contributes to the reprogramming of glucose metabolism in ovarian cancer cells (107). Nitric oxide synthase-2 (NOS2/iNOS) expressed by endogenous T cells and NO generated by T cells are required for de novo Th17 differentiation from naive precursors and for induction of the Th17 phenotype in memory cells (106).
3.3.3 Tryptophan
Tryptophan is an essential amino acid that can be catabolized or used to make tissue proteins. Patients with ovarian cancer showed accelerated tryptophan breakdown (108). There is evidence that in ovarian cancer, the enzyme indoleamine 2,3-dioxygenase (IDO) catalyzes the breakdown of tryptophan through the kynurenine pathway, resulting in immunosuppressive compounds that accelerate the growth of tumor cells and reduce antitumor immunity (109, 110). Specifically, it reduces tryptophan availability in immune cells, resulting in immunological escape by inhibiting the recruitment of NK cells and the infiltration of T cells (110, 111). Tregs can enhance IDO expression in ovarian cancer cells and synergize with hypoxia to extend the aggressiveness of OC (112). Meanwhile, tryptophan 2,3-dioxygenase (TDO2), the rate-limiting enzyme of the kynurenine pathway, was found to be upregulated in ovarian cancer tissues, which promotes tumor cell proliferation, migration and invasion (113).
3.3.4 Aspartic acid and asparagine
Asparagine (Asn) is a metabolic byproduct that is released into the extracellular compartment by tumor cells in large amounts. A metabolomics-based study of EOC serum metabolites found that Asn may be an important factor influencing the pathogenesis of ovarian cancer (114). However, specific mechanisms have yet to be explored. The presence of Asn in the microenvironment dramatically enhances the activation, proliferation and tumor killing ability of CD8+ T cells both in vivo and in vitro (115).
3.3.5 One-carbon metabolism
Large quantities of pyrimidines, thymidines, S-adenosylmethionine, and glutathione are necessary for rapid tumor proliferation for the synthesis of nucleotides. One-carbon (1C) metabolism backs up these substances required by tumors (116, 117). By affecting T cell functions, the folate-coupled metabolic enzyme methylenetetrahydrofolate dehydrogenase 2 (MTHFD2) serves as a crucial metabolic checkpoint in the 1C metabolic pathway (118). As a nicotinamide adenine dinucleotide (NAD+)-dependent enzyme with a considerable level of expression in ovarian cancer tissues, MTHFD2 positively correlates with both malignancy and prognosis (119). The synthesis of uridine-related metabolites, including UTP/UDP, is enhanced in the TIME by MTHFD2-mediated high levels of folate metabolism (120). It consequently stimulates PD-L1 transcription for the purpose of immune evasion (120). Furthermore, in IC metabolism, the methionine cycle is a major methyl donor, which is required for protein and nucleic acid methylation. Research has revealed the important role of methionine metabolism in T cell proliferation and differentiation (121). Cysteine is another significant metabolite in IC metabolism. It was discovered that ovarian cancer enhances tolerance to hypoxia through cysteine-mediated reprogramming of sulfur and carbon metabolism (122).
3.4 Metabolic signaling pathways in TIME reprogramming
The signaling pathways are linked to the shift of the metabolic landscape in ovarian cancer. Cancer cells exhibit persistent proliferative signals, and metabolic reprogramming facilitates this behavior.
The PI3K/Akt signaling pathway stimulates gluconeogenesis and lipid metabolism and activates tumor metabolism (123, 124). Salt-inducible kinase 2 (SIK2) overexpression in ovarian cancer cells activates the PI3K/AKT/HIF-1α pathway, upregulates HIF-1α expression, directly upregulates the transcription of major glycolytic genes and promotes glycolysis (123). SIK2 can promote mitochondrial fission and inhibit mitochondrial oxidative phosphorylation through phosphorylation of Drp1 at the Ser616 site (123). Moreover, SIK2 activates the PI3K/Akt signaling pathway and upregulates sterol regulatory element binding protein 1c (SREBP1c) and sterol regulatory element binding protein 2 (SREBP2), thus promoting the transcription of lipase FASN and cholesterol synthase HMGCR (124). This process improved the synthesis of cholesterol and FAs in ovarian cancer cells (124). In addition, ovarian cancer cells are capable of producing several forms of laminins (125). The Akt and MEK signaling pathways are activated in DCs cultivated with laminins in vitro, and a shift in the metabolic state of DCs induces the development of their reprogramming from bone marrow precursors to a suppressive phenotype (125).
The metabolic reprogramming of tumors is strongly correlated with the PI3K/Akt/mTOR pathway, which regulates cell growth and metabolism. The protein kinase mTOR is a serine/threonine enzyme that is a component of the mTORC1 and mTORC2 signaling complexes. In ovarian cancer, mTORC1 regulates glucose metabolism during CD8+ Treg differentiation by modulating HIF1α expression (126). Research has revealed that inhibiting GLScan synergize with the therapeutic effect of mTOR inhibitors on ovarian cancer (127).
Additionally, an aberrant MAPK signaling pathway is inextricably tied to metabolic reprogramming in ovarian cancer. It was found that TGF-β1 secreted by ovarian cancer cells could induce CD8+ Tregs through the p38 MAPK signaling pathway (128). In ovarian cancer, the widespread degradation of HIF-1 is encouraged by the silencing of TRPM7, which possesses ion channel and kinase activity. This promotes AMPK activation and changes glycolysis into oxidative phosphorylation (129).
As previously discussed, the Wingless (Wnt)/β-catenin pathway is hypothesized to be a driver of altered glycolysis, glutaminolysis, and lipogenesis (130). In ovarian cancer, hexokinase 2 increases CyclinD1/c-myc through the Wnt/β-catenin pathway, which in turn enhances oncogenesis and proliferation (131). In addition, macrophages that support ovarian cancer metastasis are linked to β-catenin expression (132). Inhibition of the Wnt/β-catenin pathway decelerates ovarian cancer progression and regulates the TIME by increasing cytotoxic T cell infiltration and decreasing MDSC infiltration (133).
4 Immunotherapeutic strategies targeting TIME metabolic reprogramming in ovarian cancer
Not only does metabolic adaptation of tumors take place throughout the course of OC, but it also happens during the course of therapy, which results in drug resistance (134–137). Metabolic reprogramming of the TIME in ovarian cancer leads to chemoresistance. M2-TAMs have a higher glucose uptake and utilization capacity, which stimulate the O-GlcNAcylation of lysosomal Cathepsin B (138). And it has been demonstrated that M2-TAMs could promote cancer metastasis and chemoresistance by increasing the activity of the hexosamine biosynthesis pathway (138). High levels of G6PD and glutathione-producing oxidoreductase are favorably associated with cisplatin resistance in OC (135). Moreover, paclitaxel resistance in ovarian cancer is associated with choline metabolism reprogramming. The expression of glycerophosphocholine phosphodiesterase 1 and glycerophosphodiester phosphodiesterase 1 in EOC was discovered to be elevated using proton magnetic resonance spectroscopy, and total choline was found to be elevated (136). Owing to the cunning metabolic adaptations of OC, monotherapy is frequently ineffective. For instance, when exposed to IDO1 inhibitors, ovarian cancer cells develop a metabolic adaptation that switches tryptophan catabolism to the 5-hydroxytryptamine pathway (137). NAD+ is increased as a result, which reduces T cell function and proliferation (137). The extracellular vesicles secreted by ovarian cancer can confer carboplatin resistance to tumor cells through hypoxia-induced metabolic dysregulation (mainly glycolysis and FAO) (139). Based on the metabolic adaptations described above in ovarian cancer, it is essential to take action to inhibit metabolic reprogramming during the treatment.
Immunotherapy allows the restoration of the T cell antitumor immune response through targeted metabolic reprogramming. Previously, we outlined the pertinent medications that target the metabolism of ovarian cancer as well as their clinical trials (29). Since there is an overlap of agents for the immunosuppressive microenvironment in ovarian cancer, we focused on immunotherapeutic strategies related to metabolic reprogramming targeting the TIME. Table 2 summarizes the immunotherapy therapies and medicines listed herein for TIME in ovarian cancer.
4.1 Supplementation or deprivation of metabolism
Certain antitumor effects can be achieved by directly supplementing metabolites. For example, L-asparaginase is a potential therapeutic enzyme. When cocultured with OVCAR-8 ovarian cancer cells, asparagine is rapidly depleted both inside and outside the cells, converting it to aspartic acid and assisting in tumor death (140). However, supplementation with amino acids in vitro shows no discernible benefit against malignancies. However, a potential involvement in disease recurrence and metastasis cannot be excluded. In vivo experiments on ovarian cancer-bearing mice showed that intraperitoneal injection of glutamine could enhance immune function and synergistically enhance paclitaxel’s antitumor effects (141).
On the other hand, metabolic deprivation is a form of starvation therapy for the treatment of ovarian cancer. The use of the specific glycolysis inhibitor 2-deoxyglucose triggers caspase-dependent apoptosis, reduces lactate production, and blocks the expression of resistance-associated proteins under low glucose conditions (30). AMPK activators can imitate the cytotoxicity induced by glucose starvation (142). Through arginine deprivation, human recombinant arginase I [HuArgI (Co)-PEG5000] can trigger autophagy and influence the motility and adhesion of ovarian cancer cells (143). Yu, J., et al. also discovered that simultaneous dual deprivation of lactate and glucose improved the antitumor effect (152). Furthermore, dietary changes might be beneficial in cancer prevention and treatment. A low-fat, high-fiber diet can help in the prevention and treatment of ovarian cancer (153, 154).
However, this adjustment of metabolic endpoint levels is extremely restricted, and most investigations have revealed no meaningful contribution to anticancer therapy in OC. It only works in a few ovarian cancer subtypes. For example, galactose intake may play a role in the development of borderline ovarian cancer in women who carry the galactose-1-phosphate uridyl transferase N314D polymorphism (155). Due to its accessibility and convenience, even though its therapeutic effect is modest, it is worthwhile to continue researching.
Adoptive cell transfer therapy (ACT) is a popular field of immunotherapy research. ACT is the collection of the patient’s own immune cells, followed by identification and in vitro cultivation to increase their quantity or improve their capacity for targeted killing (156). It can be helpful to improve the immune escape of ovarian cancer, especially for patients with recurrent ovarian cancer. An adoptive cell therapy of autologous T cells induced by a humanized anti-adiotypic antibody 6B11 minibody plus DCs and cytokines demonstrated preliminary safety and potential clinical efficacy in a phase I clinical trial of platinum-resistant recurrent or refractory ovarian cancer (144). A phase II study of allogeneic NK cell therapy for recurrent ovarian cancer showed suboptimal efficacy, and Treg cells were revealed to represent a treatment barrier (157). Allogeneic NK cell infusion in the most recent phase 1 clinical trial is still recruiting participants (158). In ovarian cancer TIME, the self-generated nanosystem known as KT-NE (KIRA6 loaded α-Tocopherol nanoemulsion) dramatically reduced lipid buildup in DCs and restored their immunological activity (159). In tumor-bearing mice, adoptive transfer of KT-NE-treated ID8-DCs increased host progression-free survival (159). Furthermore, PD-1 immunotherapy and KT-NE had synergistic effects (159). Additional clinical studies are needed to explore the therapeutic effects of ACT in ovarian cancer.
4.2 Immune checkpoint inhibitors
Checkpoint receptors on the surface of T cells, such as PD-1 and cytotoxic T lymphocyte-associated antigen 4 (CTLA-4), can suppress energy and metabolic changes in T cells and mediate immunosuppression when activated by the corresponding ligands (160). Immune checkpoint blockade (ICB) therapy enhances the tumor infiltration and effector functions of T cells by reprogramming metabolism. Classical PD-1, PD-L1, and CTLA-4 inhibitors have made great progress in the treatment of ovarian cancer. Immune checkpoints show a positive modulatory effect on metabolism (160, 161). PD-1 and CTLA-4 inhibitors can modulate the amount and activity of ARG-1, which prevents MDSCs from suppressing the immune system in ovarian cancer (161).
The IDO and kynurenine pathways are emerging metabolic checkpoints that promote T cell proliferation by preventing the synthesis of kynurenine (162). The aryl hydrocarbon receptor (AHR) antagonist CH223191 significantly decreased AHR-induced PD-1 expression in T cells (145).
However, the efficacy of ICB in the treatment of ovarian cancer is not obvious (163). In patients with advanced malignancies, combinations of PD-L1 inhibitors and IDO inhibitors have demonstrated acceptable safety and resistance (146). But there is no proof to back up the benefits of the combination. The metabolic reprogramming of the TIME is probably an important cause for the weak efficacy of ICB. The combination of drugs targeting metabolic reprogramming and ICIs is expected to improve the efficacy of ICB. Based on the glutamine dependence of ovarian cancer, the GLS inhibitor 968 increased the infiltration of CD3+ T cells and enhanced the apoptosis-inducing ability of cancer cells by CD8+ T cells. Combination with PD-L1 blockade enhanced the immune response to ovarian cancer (147).
The in-depth exploration of metabolic reprogramming has allowed for the continuous refinement of immunotherapy in ovarian cancer. For example, ICB induces not only IDO1 but also interleukin-4-induced-1 (IL4I1). The failure of clinical studies of ICB combined with IDO1 inhibition may be due to the presence of IL4I1 (164). The discovery of new metabolic immune checkpoints opens up a new path for cancer therapy.
4.3 Others
By targeting intracellular metabolic reprogramming, the metabolic state of T cells was induced to change, resulting in a more effective ovarian cancer treatment. Specific metabolism-related cell surface receptors can be identified as targets for selective delivery therapies based on the metabolic reprogramming properties of ovarian cancer. Folate receptor (FR) β in one-carbon metabolism can also be used as a regulatory target. It is expressed on activated macrophages in patients with EOC, and its blockade can inhibit nucleotide production, leading to significant immunotherapeutic effects (165, 166).
As more is known about tumor immunity, cancer vaccines have received increasing attention. Various cancer vaccines targeting immune cells have demonstrated a promising therapeutic landscape in ovarian cancer. In a phase II clinical trial, an autologous DC-based vaccination (DCVAC) significantly increased progression-free survival, and peripheral blood analysis revealed that DCVAC increases anti-cancer immunity in ovarian cancer patients with cool tumor nature (148, 149). A single-arm open-label phase I clinical trial indicates the safety of a Th17-induced FR-loaded autologous DC vaccination in inducing antigen-specific immunity and prolonging remission in ovarian cancer patients (150). Oncolytic virotherapy is an emerging immunotherapy. Oncolytic viruses invade tumor cells through cell surface molecules, infect and kill tumor cells, activate the immune response, and thus exert their tumor-killing effect (167). Lysozyme virus has high selectivity to tumor cells and high immune response intensity, thus maximizing the effect of immunotherapy. Gene set enrichment analysis showed that SV. IL12 in combination with anti-OX40 increased intracellular glycolysis and OXPHOS in T cells at the genetic level (151). Hulin-Curtis, S. L., et al. designed a phage peptide that binds to FRα on SKOV3 cell lines based on the selective high expression of FRα on ovarian cancer cells (168). It binds specifically to FRα, however, due to defective intracellular transport, it cannot yet be effectively targeted through FRα (168).
The above researches show promising therapeutic effects of tumor vaccines and oncolytic virotherapy for the treatment of ovarian cancer. However, because the relevant technology and exploration are not yet very mature, most of the current development is still only at the preclinical stage. More immunotherapy targeting TIME need to be developed in the future.
5 Perspectives and prospects
New insights suggest that tumors are not only a genetic disease but also highly associated with a suppressive immune microenvironment (16). Cellular metabolism is the key link between the extracellular environment and intracellular processes. Therefore, it is essential to explore the immunity and metabolism of ovarian cancer. By exploring the immunosuppressive microenvironment of ovarian cancer, we decipher what significant role environmental factors, especially immune cells, play in tumorigenesis, development, treatment and prognosis. Single-cell sequencing and integrated bioinformatics analysis have led to a greater understanding of ovarian cancer. J. Sun et al. described the immunogenomic landscape of HGSOC and identified immunological subtypes appropriate for immunotherapy (169). The application of immunogenomics, immunogenomics and molecular typing in ovarian cancer will contribute to subsequent targeted therapies. By deeply researching different immune subtypes, the application of precision therapy in ovarian cancer can be further expanded.
Ovarian cancer patients inevitably develop resistance to drugs, leading to treatment failure, which is the leading cause of death. Metabolic reprogramming of tumors occurs not only in tumorigenesis, progression, and metastasis, but different therapeutic measures also reshape tumor metabolism. It has been proven that surgery could increase the immune suppression of first-line ovarian cancer treatment (21). Metabolic reprogramming is extremely complex. In addition to the discussion in this review, epigenetics also plays an indelible role in the process of metabolic reprogramming (170). For example, ubiquitination and deubiquitination could alter cancer metabolism as one of the types of posttranslational modifications (171). Ovarian cancer restricts methyltransferase EZH2 expression in T cells by limiting aerobic glycolysis, thereby impairing T-cell-mediated antitumor immunity (172). Epigenetic regulation, in turn, can also affect cellular metabolism by modifying kinase activity. To illustrate, the DNA demethylating agent 5-aza-2-deoxycytidine reduces chemoresistance in cisplatin-resistant A2780cis cells and restores GS expression (173). Therefore, integrating the mechanism of interaction between metabolic reprogramming and epigenetics is also an important direction for future metabolic research in ovarian cancer, which could provide a theoretical foundation for treatment.
However, the metabolic phenotype of cancer is not invariant. Treatment resistance and metastases also cause metabolic reprogramming. It is feasible to increase the sensitivity of immunotherapy and chemotherapy as a new complement to the treatment of malignancies by targeting this feature. It is extremely promising to create medications to postpone the progression of tumors and to improve the sensitivity of cancer treatment based on the relevant theories. Therefore, it is crucial to continue researching metabolic reprogramming to enhance tumor immunotherapy.
Author contributions
Conceptualization, YL and XL. Writing, review, and editing, YL and XtZ. Visualization, YL and YN. Supervision, XL and XZ. Funding acquisition, XZ and XL. All authors have read and agreed to the published version of the manuscript.
Funding
This research was funded by the National Natural Science Foundation of China, grant number No. 81902662, the Natural Science Foundation of Sichuan Province, grant number No.2022NSFSC1531, the National Natural Science Foundation of China, grant number No. 81821002, Sichuan Science and Technology Program, grant number 2021YJ0011, and Foundation of Development and Related Diseases of Women and Children Key Laboratory of Sichuan Province, grant number No. 2022003. The APC was funded by West China Second Hospital.
Acknowledgments
Figures in this review were created with BioRender.com.
Conflict of interest
The authors declare that the research was conducted in the absence of any commercial or financial relationships that could be construed as a potential conflict of interest.
Publisher’s note
All claims expressed in this article are solely those of the authors and do not necessarily represent those of their affiliated organizations, or those of the publisher, the editors and the reviewers. Any product that may be evaluated in this article, or claim that may be made by its manufacturer, is not guaranteed or endorsed by the publisher.
Glossary
References
1. Torre LA, Trabert B, DeSantis CE, Miller KD, Samimi G, Runowicz CD, et al. Ovarian cancer statistics, 2018. CA Cancer J Clin (2018) 68(4):284–96. doi: 10.3322/caac.21456
2. Coburn SB, Bray F, Sherman ME, Trabert B. International patterns and trends in ovarian cancer incidence, overall and by histologic subtype. Int J Cancer (2017) 140(11):2451–60. doi: 10.1002/ijc.30676
3. Siegel RL, Miller KD, Fuchs HE, Jemal A. Cancer statistics, 2021. CA Cancer J Clin (2021) 71(1):7–33. doi: 10.3322/caac.21654
4. Stewart C, Ralyea C, Lockwood S. Ovarian cancer: An integrated review. Semin Oncol Nurs (2019) 35(2):151–6. doi: 10.1016/j.soncn.2019.02.001
5. Bogani G, Ditto A, Pinelli C, Lopez S, Chiappa V, Raspagliesi F. Ten-year follow-up study of long-term outcomes after conservative surgery for early-stage ovarian cancer. Int J Gynaecol Obstet (2020) 150(2):169–76. doi: 10.1002/ijgo.13199
6. Mazzoni A, Bronte V, Visintin A, Spitzer JH, Apolloni E, Serafini P, et al. Myeloid suppressor lines inhibit T cell responses by an NO-dependent mechanism. J Immunol (2002) 168(2):689–95. doi: 10.4049/jimmunol.168.2.689
7. Armstrong DK, Alvarez RD, Bakkum-Gamez JN, Barroilhet L, Behbakht K, Berchuck A, et al. Ovarian cancer, version 2.2020, NCCN clinical practice guidelines in oncology. J Natl Compr Canc Netw (2021) 19(2):191–226. doi: 10.6004/jnccn.2021.0007
8. Shih IM, Wang Y, Wang TL. The origin of ovarian cancer species and precancerous landscape. Am J Pathol (2021) 191(1):26–39. doi: 10.1016/j.ajpath.2020.09.006
9. Dalmartello M, La Vecchia C, Bertuccio P, Boffetta P, Levi F, Negri E, et al. European Cancer mortality predictions for the year 2022 with focus on ovarian cancer. Ann Oncol (2022) 33(3):330–9. doi: 10.1016/j.annonc.2021.12.007
10. Menon U, Gentry-Maharaj A, Burnell M, Singh N, Ryan A, Karpinskyj C, et al. Ovarian cancer population screening and mortality after long-term follow-up in the UK collaborative trial of ovarian cancer screening (UKCTOCS): A randomised controlled trial. Obstet Gynecol Survey (2021) 76(9):537–8. doi: 10.1097/01.ogx.0000792624.55995.3e
11. Luvero D, Milani A, Ledermann JA. Treatment options in recurrent ovarian cancer: latest evidence and clinical potential. Ther Adv Med Oncol (2014) 6(5):229–39. doi: 10.1177/1758834014544121
12. Granata V, Crisafulli L, Nastasi C, Ficara F, Sobacchi C. Bone marrow niches and tumour cells: Lights and shadows of a mutual relationship. Front Immunol (2022) 13:884024. doi: 10.3389/fimmu.2022.884024
13. Lakshmi Narendra B, Eshvendar Reddy K, Shantikumar S, Ramakrishna S. Immune system: a double-edged sword in cancer. Inflamm Res (2013) 62(9):823–34. doi: 10.1007/s00011-013-0645-9
14. Bhatia A, Kumar Y. Cellular and molecular mechanisms in cancer immune escape: a comprehensive review. Expert Rev Clin Immunol (2014) 10(1):41–62. doi: 10.1586/1744666X.2014.865519
15. Paget S. The distribution of secondary growths in cancer of the breast. 1889. Cancer Metastasis Rev (1989) 8(2):98–101. doi: 10.1016/s0140-6736(00)49915-0
16. Binnewies M, Roberts EW, Kersten K, Chan V, Fearon DF, Merad M, et al. Understanding the tumor immune microenvironment (TIME) for effective therapy. Nat Med (2018) 24(5):541–50. doi: 10.1038/s41591-018-0014-x
17. Odunsi K. Immunotherapy in ovarian cancer. Ann Oncol (2017) 28(suppl_8):viii1–7. doi: 10.1093/annonc/mdx444
18. Tan S, Li D, Zhu X. Cancer immunotherapy: Pros, cons and beyond. BioMed Pharmacother (2020) 124:109821. doi: 10.1016/j.biopha.2020.109821
19. Sobhani N, Scaggiante B, Morris R, Chai D, Catalano M, Tardiel-Cyril DR, et al. Therapeutic cancer vaccines: From biological mechanisms and engineering to ongoing clinical trials. Cancer Treat Rev (2022) 109:102429. doi: 10.1016/j.ctrv.2022.102429
20. Pirs B, Skof E, Smrkolj V, Smrkolj S. Overview of immune checkpoint inhibitors in gynecological cancer treatment. Cancers (Basel) (2022) 14(3):631. doi: 10.3390/cancers14030631
21. De Bruyn C, Ceusters J, Landolfo C, Baert T, Thirion G, Claes S, et al. Neo-adjuvant chemotherapy reduces, and surgery increases immunosuppression in first-line treatment for ovarian cancer. Cancers (Basel) (2021) 13(23):5899. doi: 10.3390/cancers13235899
22. Le Saux O, Ray-Coquard I, Labidi-Galy SI. Challenges for immunotherapy for the treatment of platinum resistant ovarian cancer. Semin Cancer Biol (2021) 77:127–43. doi: 10.1016/j.semcancer.2020.08.017
23. Morand S, Devanaboyina M, Staats H, Stanbery L, Nemunaitis J. Ovarian cancer immunotherapy and personalized medicine. Int J Mol Sci (2021) 22(12):6532. doi: 10.3390/ijms22126532
24. Anadon CM, Yu X, Hanggi K, Biswas S, Chaurio RA, Martin A, et al. Ovarian cancer immunogenicity is governed by a narrow subset of progenitor tissue-resident memory T cells. Cancer Cell (2022) 40(5):545–557e13. doi: 10.1016/j.ccell.2022.03.008
25. Yoshida GJ. Metabolic reprogramming: The emerging concept and associated therapeutic strategies. J Exp Clin Cancer Res (2015) 34:111. doi: 10.1186/s13046-015-0221-y
26. Hanahan D. Hallmarks of cancer: New dimensions. Cancer Discovery (2022) 12(1):31–46. doi: 10.1158/2159-8290.CD-21-1059
27. Dar S, Chhina J, Mert I, Chitale D, Buekers T, Kaur H, et al. Bioenergetic adaptations in chemoresistant ovarian cancer cells. Sci Rep (2017) 7(1):8760. doi: 10.1038/s41598-017-09206-0
28. Liberti MV, Locasale JW. The warburg effect: How does it benefit cancer cells? Trends Biochem Sci (2016) 41(3):211–8. doi: 10.1016/j.tibs.2015.12.001
29. Lin Y, Liang X, Zhang X, Ni Y, Zhou X, Zhao X. Metabolic cross-talk between ovarian cancer and the tumor microenvironment-providing potential targets for cancer therapy. Front Biosci (Landmark Ed) (2022) 27(4):139. doi: 10.31083/j.fbl2704139
30. Park GB, Jeong JY, Choi S, Yoon YS, Kim D. Glucose deprivation enhances resistance to paclitaxel via ELAVL2/4-mediated modification of glycolysis in ovarian cancer cells. Anticancer Drugs (2022) 33(1):e370–80. doi: 10.1097/CAD.0000000000001215
31. Ding Y, Labitzky V, Legler K, Qi M, Schumacher U, Schmalfeldt B, et al. Molecular characteristics and tumorigenicity of ascites-derived tumor cells: Mitochondrial oxidative phosphorylation as a novel therapy target in ovarian cancer. Mol Oncol (2021) 15(12):3578–95. doi: 10.1002/1878-0261.13028
32. Bose S, Huang Q, Ma Y, Wang L, Rivera GO, Ouyang Y, et al. G6PD inhibition sensitizes ovarian cancer cells to oxidative stress in the metastatic omental microenvironment. Cell Rep (2022) 39(13):111012. doi: 10.1016/j.celrep.2022.111012
33. Prasad P, Roy SS. Glutamine regulates ovarian cancer cell migration and invasion through ETS1. Heliyon (2021) 7(5):e07064. doi: 10.1016/j.heliyon.2021.e07064
34. Sriramkumar S, Sood R, Huntington TD, Ghobashi AH, Vuong TT, Metcalfe TX, et al. Platinum-induced mitochondrial OXPHOS contributes to cancer stem cell enrichment in ovarian cancer. J Transl Med (2022) 20(1):246. doi: 10.1186/s12967-022-03447-y
35. Ji Z, Shen Y, Feng X, Kong Y, Shao Y, Meng J, et al. Deregulation of lipid metabolism: The critical factors in ovarian cancer. Front Oncol (2020) 10:593017. doi: 10.3389/fonc.2020.593017
36. Dogra S, Neelakantan D, Patel MM, Griesel B, Olson A, Woo S. Adipokine Apelin/APJ pathway promotes peritoneal dissemination of ovarian cancer cells by regulating lipid metabolism. Mol Cancer Res (2021) 19(9):1534–45. doi: 10.1158/1541-7786.MCR-20-0991
37. Nieman KM, Kenny HA, Penicka CV, Ladanyi A, Buell-Gutbrod R, Zillhardt MR, et al. Adipocytes promote ovarian cancer metastasis and provide energy for rapid tumor growth. Nat Med (2011) 17(11):1498–503. doi: 10.1038/nm.2492
38. Nersesian S, Glazebrook H, Toulany J, Grantham SR, Boudreau JE. Naturally killing the silent killer: NK cell-based immunotherapy for ovarian cancer. Front Immunol (2019) 10:1782. doi: 10.3389/fimmu.2019.01782
39. Pugh-Toole M, Nicolela AP, Nersesian S, Leung BM, Boudreau JE. Natural killer cells: The missing link in effective treatment for high-grade serous ovarian carcinoma. Curr Treat Options Oncol (2022) 23(2):210–26. doi: 10.1007/s11864-021-00929-x
40. Mortezaee K, Majidpoor J. Dysregulated metabolism: A friend-to-foe skewer of macrophages. Int Rev Immunol (2022) 135:1–17. doi: 10.1080/08830185.2022.2095374
41. Liu Y, Xu R, Gu H, Zhang E, Qu J, Cao W, et al. Metabolic reprogramming in macrophage responses. biomark Res (2021) 9(1):1. doi: 10.1186/s40364-020-00251-y
42. Franco F, Jaccard A, Romero P, Yu YR, Ho PC. Metabolic and epigenetic regulation of T-cell exhaustion. Nat Metab (2020) 2(10):1001–12. doi: 10.1038/s42255-020-00280-9
43. Martin MD, Badovinac VP. Defining memory CD8 T cell. Front Immunol (2018) 9:2692. doi: 10.3389/fimmu.2018.02692
44. Dong H, Bullock TN. Metabolic influences that regulate dendritic cell function in tumors. Front Immunol (2014) 5:24. doi: 10.3389/fimmu.2014.00024
45. Thwe PM, Pelgrom LR, Cooper R, Beauchamp S, Reisz JA, D'Alessandro A, et al. Cell-intrinsic glycogen metabolism supports early glycolytic reprogramming required for dendritic cell immune responses. Cell Metab (2017) 26(3):558–567e5. doi: 10.1016/j.cmet.2017.08.012
46. Xu R, Wu M, Liu S, Shang W, Li R, Xu J, et al. Glucose metabolism characteristics and TLR8-mediated metabolic control of CD4(+) treg cells in ovarian cancer cells microenvironment. Cell Death Dis (2021) 12(1):22. doi: 10.1038/s41419-020-03272-5
47. Yin M, Li X, Tan S, Zhou HJ, Ji W, Bellone S, et al. Tumor-associated macrophages drive spheroid formation during early transcoelomic metastasis of ovarian cancer. J Clin Invest (2016) 126(11):4157–73. doi: 10.1172/JCI87252
48. Al-Khami AA, Zheng L, Del Valle L, Hossain F, Wyczechowska D, Zabaleta J, et al. Exogenous lipid uptake induces metabolic and functional reprogramming of tumor-associated myeloid-derived suppressor cells. Oncoimmunology (2017) 6(10):e1344804. doi: 10.1080/2162402X.2017.1344804
49. Udumula MP, Sakr S, Dar S, Alvero AB, Ali-Fehmi R, Abdulfatah E, et al. Ovarian cancer modulates the immunosuppressive function of CD11b(+)Gr1(+) myeloid cells via glutamine metabolism. Mol Metab (2021) 53:101272. doi: 10.1016/j.molmet.2021.101272
50. Leonard W, Dufait I, Schwarze JK, Law K, Engels B, Jiang H, et al. Myeloid-derived suppressor cells reveal radioprotective properties through arginase-induced l-arginine depletion. Radiother Oncol (2016) 119(2):291–9. doi: 10.1016/j.radonc.2016.01.014
51. Bak SP, Alonso A, Turk MJ, Berwin B. Murine ovarian cancer vascular leukocytes require arginase-1 activity for T cell suppression. Mol Immunol (2008) 46(2):258–68. doi: 10.1016/j.molimm.2008.08.266
52. Zhu H, Blum RH, Bjordahl R, Gaidarova S, Rogers P, Lee TT, et al. Pluripotent stem cell-derived NK cells with high-affinity noncleavable CD16a mediate improved antitumor activity. Blood (2020) 135(6):399–410. doi: 10.1182/blood.2019000621
53. Sheppard S, Santosa EK, Lau CM, Violante S, Giovanelli P, Kim H, et al. Lactate dehydrogenase a-dependent aerobic glycolysis promotes natural killer cell anti-viral and anti-tumor function. Cell Rep (2021) 35(9):109210. doi: 10.1016/j.celrep.2021.109210
54. Deng M, Wu D, Zhang Y, Jin Z, Miao J. MiR-29c downregulates tumor-expressed B7-H3 to mediate the antitumor NK-cell functions in ovarian cancer. Gynecol Oncol (2021) 162(1):190–9. doi: 10.1016/j.ygyno.2021.04.013
55. Biswas SK, Sica A, Lewis CE. Plasticity of macrophage function during tumor progression: regulation by distinct molecular mechanisms. J Immunol (2008) 180(4):2011–7. doi: 10.4049/jimmunol.180.4.2011
56. Wang H, Yung MMH, Ngan HYS, Chan KKL, Chan DW. The impact of the tumor microenvironment on macrophage polarization in cancer metastatic progression. Int J Mol Sci (2021) 22(12). doi: 10.3390/ijms22126560
57. Hishinuma E, Shimada M, Matsukawa N, Saigusa D, Li B, Kudo K, et al. Wide-targeted metabolome analysis identifies potential biomarkers for prognosis prediction of epithelial ovarian cancer. Toxins (Basel) (2021) 13(7):461. doi: 10.3390/toxins13070461
58. Zhang M, He Y, Sun X, Li Q, Wang W, Zhao A, et al. A high M1/M2 ratio of tumor-associated macrophages is associated with extended survival in ovarian cancer patients. J Ovarian Res (2014) 7:19. doi: 10.1186/1757-2215-7-19
59. Winkler I, Wilczynska B, Bojarska-Junak A, Gogacz M, Adamiak A, Postawski K, et al. Regulatory T lymphocytes and transforming growth factor beta in epithelial ovarian tumors-prognostic significance. J Ovarian Res (2015) 8:39. doi: 10.1186/s13048-015-0164-0
60. Pompura SL, Wagner A, Kitz A, LaPerche J, Yosef N, Dominguez-Villar M, et al. Oleic acid restores suppressive defects in tissue-resident FOXP3 tregs from patients with multiple sclerosis. J Clin Invest (2021) 131(2):138519. doi: 10.1172/JCI138519
61. Zhang Z, Liu Q, Che Y, Yuan X, Dai L, Zeng B, et al. Antigen presentation by dendritic cells in tumors is disrupted by altered metabolism that involves pyruvate kinase M2 and its interaction with SOCS3. Cancer Res (2010) 70(1):89–98. doi: 10.1158/0008-5472.CAN-09-2970
62. Mabuchi S, Sasano T, Komura N. Targeting myeloid-derived suppressor cells in ovarian cancer. Cells (2021) 10(2):329. doi: 10.3390/cells10020329
63. Olkowicz M, Rosales-Solano H, Kulasingam V, Pawliszyn J. SPME-LC/MS-based serum metabolomic phenotyping for distinguishing ovarian cancer histologic subtypes: A pilot study. Sci Rep (2021) 11(1):22428. doi: 10.1038/s41598-021-00802-9
64. Dier U, Shin DH, Hemachandra LP, Uusitalo LM, Hempel N. Bioenergetic analysis of ovarian cancer cell lines: Profiling of histological subtypes and identification of a mitochondria-defective cell line. PloS One (2014) 9(5):e98479. doi: 10.1371/journal.pone.0098479
65. Wu S, Fukumoto T, Lin J, Nacarelli T, Wang Y, Ong D, et al. Targeting glutamine dependence through GLS1 inhibition suppresses ARID1A-inactivated clear cell ovarian carcinoma. Nat Cancer (2021) 2(2):189–200. doi: 10.1038/s43018-020-00160-x
66. Gaul DA, Mezencev R, Long TQ, Jones CM, Benigno BB, Gray A, et al. Highly-accurate metabolomic detection of early-stage ovarian cancer. Sci Rep (2015) 5:16351. doi: 10.1038/srep16351
67. Kumagai S, Koyama S, Itahashi K, Tanegashima T, Lin YT, Togashi Y, et al. Lactic acid promotes PD-1 expression in regulatory T cells in highly glycolytic tumor microenvironments. Cancer Cell (2022) 40(2):201–218e9. doi: 10.1016/j.ccell.2022.01.001
68. Bhattacharya R, Ray Chaudhuri S, Roy SS. FGF9-induced ovarian cancer cell invasion involves VEGF-A/VEGFR2 augmentation by virtue of ETS1 upregulation and metabolic reprogramming. J Cell Biochem (2018) 119(10):8174–89. doi: 10.1002/jcb.26820
69. Paolini L, Adam C, Beauvillain C, Preisser L, Blanchard S, Pignon P, et al. Lactic acidosis together with GM-CSF and m-CSF induces human macrophages toward an inflammatory protumor phenotype. Cancer Immunol Res (2020) 8(3):383–95. doi: 10.1158/2326-6066.CIR-18-0749
70. Bernacchioni C, Ghini V, Cencetti F, Japtok L, Donati C, Bruni P, et al. NMR metabolomics highlights sphingosine kinase-1 as a new molecular switch in the orchestration of aberrant metabolic phenotype in cancer cells. Mol Oncol (2017) 11(5):517–33. doi: 10.1002/1878-0261.12048
71. Elia I, Rowe JH, Johnson S, Joshi S, Notarangelo G, Kurmi K, et al. Tumor cells dictate anti-tumor immune responses by altering pyruvate utilization and succinate signaling in CD8(+) T cells. Cell Metab (2022) 34(8):1137–1150e6. doi: 10.1016/j.cmet.2022.06.008
72. Israelsen WJ, Vander Heiden MG. Pyruvate kinase: Function, regulation and role in cancer. Semin Cell Dev Biol (2015) 43:43–51. doi: 10.1016/j.semcdb.2015.08.004
73. Chen M, Sheng XJ, Qin YY, Zhu S, Wu QX, Jia L, et al. TBC1D8 amplification drives tumorigenesis through metabolism reprogramming in ovarian cancer. Theranostics (2019) 9(3):676–90. doi: 10.7150/thno.30224
74. Li S, Ji X, Wang R, Miao Y. Follicle-stimulating hormone promoted pyruvate kinase isozyme type M2-induced glycolysis and proliferation of ovarian cancer cells. Arch Gynecol Obstet (2019) 299(5):1443–51. doi: 10.1007/s00404-019-05100-4
75. Chang CH, Curtis JD, Maggi LB Jr., Faubert B, Villarino AV, O'Sullivan D, et al. Posttranscriptional control of T cell effector function by aerobic glycolysis. Cell (2013) 153(6):1239–51. doi: 10.1016/j.cell.2013.05.016
76. Anwar S, Shamsi A, Mohammad T, Islam A, Hassan MI. Targeting pyruvate dehydrogenase kinase signaling in the development of effective cancer therapy. Biochim Biophys Acta Rev Cancer (2021) 1876(1):188568. doi: 10.1016/j.bbcan.2021.188568
77. Yao S, Shang W, Huang L, Xu R, Wu M, Wang F. The oncogenic and prognostic role of PDK1 in the progression and metastasis of ovarian cancer. J Cancer (2021) 12(3):630–43. doi: 10.7150/jca.47278
78. Zhang W, Su J, Xu H, Yu S, Liu Y, Zhang Y, et al. Dicumarol inhibits PDK1 and targets multiple malignant behaviors of ovarian cancer cells. PloS One (2017) 12(6):e0179672. doi: 10.1371/journal.pone.0179672
79. Zhou L, Liu L, Chai W, Zhao T, Jin X, Guo X, et al. Dichloroacetic acid upregulates apoptosis of ovarian cancer cells by regulating mitochondrial function. Onco Targets Ther (2019) 12:1729–39. doi: 10.2147/OTT.S194329
80. Wang JJ, Siu MK, Jiang YX, Leung TH, Chan DW, Cheng RR, et al. Aberrant upregulation of PDK1 in ovarian cancer cells impairs CD8(+) T cell function and survival through elevation of PD-L1. Oncoimmunology (2019) 8(11):e1659092. doi: 10.1080/2162402X.2019.1659092
81. Kitamura S, Yamaguchi K, Murakami R, Furutake Y, Higasa K, Abiko K, et al. PDK2 leads to cisplatin resistance through suppression of mitochondrial function in ovarian clear cell carcinoma. Cancer Sci (2021) 112(11):4627–40. doi: 10.1111/cas.15125
82. Weiss JM, Davies LC, Karwan M, Ileva L, Ozaki MK, Cheng RY, et al. Itaconic acid mediates crosstalk between macrophage metabolism and peritoneal tumors. J Clin Invest (2018) 128(9):3794–805. doi: 10.1172/JCI99169
83. Chen LL, Morcelle C, Cheng ZL, Chen X, Xu Y, Gao Y, et al. Itaconate inhibits TET DNA dioxygenases to dampen inflammatory responses. Nat Cell Biol (2022) 24(3):353–63. doi: 10.1038/s41556-022-00853-8
84. Sato M, Kawana K, Adachi K, Fujimoto A, Yoshida M, Nakamura H, et al. Detachment from the primary site and suspension in ascites as the initial step in metabolic reprogramming and metastasis to the omentum in ovarian cancer. Oncol Lett (2018) 15(1):1357–61. doi: 10.3892/ol.2017.7388
85. Ladanyi A, Mukherjee A, Kenny HA, Johnson A, Mitra AK, Sundaresan S, et al. Adipocyte-induced CD36 expression drives ovarian cancer progression and metastasis. Oncogene (2018) 37(17):2285–301. doi: 10.1038/s41388-017-0093-z
86. Schumann T, Adhikary T, Wortmann A, Finkernagel F, Lieber S, Schnitzer E, et al. Deregulation of PPARbeta/delta target genes in tumor-associated macrophages by fatty acid ligands in the ovarian cancer microenvironment. Oncotarget (2015) 6(15):13416–33. doi: 10.18632/oncotarget.3826
87. So J, Wang FQ, Navari J, Schreher J, Fishman DA. LPA-induced epithelial ovarian cancer (EOC) in vitro invasion and migration are mediated by VEGF receptor-2 (VEGF-R2). Gynecol Oncol (2005) 97(3):870–8. doi: 10.1016/j.ygyno.2005.03.004
88. Meng Y, Kang S, So J, Reierstad S, Fishman DA. Translocation of fas by LPA prevents ovarian cancer cells from anti-fas-induced apoptosis. Gynecol Oncol (2005) 96(2):462–9. doi: 10.1016/j.ygyno.2004.10.024
89. Reinartz S, Lieber S, Pesek J, Brandt DT, Asafova A, Finkernagel F, et al. Cell type-selective pathways and clinical associations of lysophosphatidic acid biosynthesis and signaling in the ovarian cancer microenvironment. Mol Oncol (2019) 13(2):185–201. doi: 10.1002/1878-0261.12396
90. Gupta VK, Jaiswara PK, Sonker P, Rawat SG, Tiwari RK, Kumar A. Lysophosphatidic acid promotes survival of T lymphoma cells by altering apoptosis and glucose metabolism. Apoptosis (2020) 25(1-2):135–50. doi: 10.1007/s10495-019-01585-1
91. Wang J, Yuen BH, Leung PC. Stimulation of progesterone and prostaglandin E2 production by lipoxygenase metabolites of arachidonic acid. FEBS Lett (1989) 244(1):154–8. doi: 10.1016/0014-5793(89)81182-2
92. Zhang X, Yan K, Deng L, Liang J, Liang H, Feng D, et al. Cyclooxygenase 2 promotes proliferation and invasion in ovarian cancer cells via the PGE2/NF-kappaB pathway. Cell Transplant (2019) 28(1_suppl):1S–13S. doi: 10.1177/0963689719890597
93. Obermajer N, Muthuswamy R, Odunsi K, Edwards RP, Kalinski P. PGE(2)-induced CXCL12 production and CXCR4 expression controls the accumulation of human MDSCs in ovarian cancer environment. Cancer Res (2011) 71(24):7463–70. doi: 10.1158/0008-5472.CAN-11-2449
94. Obermajer N, Muthuswamy R, Lesnock J, Edwards RP, Kalinski P. Positive feedback between PGE2 and COX2 redirects the differentiation of human dendritic cells toward stable myeloid-derived suppressor cells. Blood (2011) 118(20):5498–505. doi: 10.1182/blood-2011-07-365825
95. Chen X, Ying X, Wang X, Wu X, Zhu Q, Wang X. Exosomes derived from hypoxic epithelial ovarian cancer deliver microRNA-940 to induce macrophage M2 polarization. Oncol Rep (2017) 38(1):522–8. doi: 10.3892/or.2017.5697
96. Wise DR, Thompson CB. Glutamine addiction: A new therapeutic target in cancer. Trends Biochem Sci (2010) 35(8):427–33. doi: 10.1016/j.tibs.2010.05.003
97. Yang L, Moss T, Mangala LS, Marini J, Zhao H, Wahlig S, et al. Metabolic shifts toward glutamine regulate tumor growth, invasion and bioenergetics in ovarian cancer. Mol Syst Biol (2014) 10:728. doi: 10.1002/msb.20134892
98. Menga A, Favia M, Spera I, Vegliante MC, Gissi R, De Grassi A, et al. N-acetylaspartate release by glutaminolytic ovarian cancer cells sustains protumoral macrophages. EMBO Rep (2021) 22(9):e51981. doi: 10.15252/embr.202051981
99. Song M, Sandoval TA, Chae CS, Chopra S, Tan C, Rutkowski MR, et al. IRE1alpha-XBP1 controls T cell function in ovarian cancer by regulating mitochondrial activity. Nature (2018) 562(7727):423–8. doi: 10.1038/s41586-018-0597-x
100. MacPherson S, Keyes S, Kilgour MK, Smazynski J, Chan V, Sudderth J, et al. Clinically relevant T cell expansion media activate distinct metabolic programs uncoupled from cellular function. Mol Ther Methods Clin Dev (2022) 24:380–93. doi: 10.1016/j.omtm.2022.02.004
101. Czystowska-Kuzmicz M, Sosnowska A, Nowis D, Ramji K, Szajnik M, Chlebowska-Tuz J, et al. Small extracellular vesicles containing arginase-1 suppress T-cell responses and promote tumor growth in ovarian carcinoma. Nat Commun (2019) 10(1):3000. doi: 10.1038/s41467-019-10979-3
102. Garcia-Navas R, Gajate C, Mollinedo F. Neutrophils drive endoplasmic reticulum stress-mediated apoptosis in cancer cells through arginase-1 release. Sci Rep (2021) 11(1):12574. doi: 10.1038/s41598-021-91947-0
103. Xia H, Li S, Li X, Wang W, Bian Y, Wei S, et al. Autophagic adaptation to oxidative stress alters peritoneal residential macrophage survival and ovarian cancer metastasis. JCI Insight (2020) 5(18):141115. doi: 10.1172/jci.insight.141115
104. Lamas B, Vergnaud-Gauduchon J, Goncalves-Mendes N, Perche O, Rossary A, Vasson MP, et al. Altered functions of natural killer cells in response to l-arginine availability. Cell Immunol (2012) 280(2):182–90. doi: 10.1016/j.cellimm.2012.11.018
105. Caneba CA, Yang L, Baddour J, Curtis R, Win J, Hartig S, et al. Nitric oxide is a positive regulator of the warburg effect in ovarian cancer cells. Cell Death Dis (2014) 5:e1302. doi: 10.1038/cddis.2014.264
106. Obermajer N, Wong JL, Edwards RP, Chen K, Scott M, Khader S, et al. Induction and stability of human Th17 cells require endogenous NOS2 and cGMP-dependent NO signaling. J Exp Med (2013) 210(7):1433–445. doi: 10.1084/jem.20121277
107. Gao W, Huang M, Chen X, Chen J, Zou Z, Li L, et al. The role of s-nitrosylation of PFKM in regulation of glycolysis in ovarian cancer cells. Cell Death Dis (2021) 12(4):408. doi: 10.1038/s41419-021-03681-0
108. Sperner-Unterweger B, Neurauter G, Klieber M, Kurz K, Meraner V, Zeimet A, et al. Enhanced tryptophan degradation in patients with ovarian carcinoma correlates with several serum soluble immune activation markers. Immunobiology (2011) 216(3):296–301. doi: 10.1016/j.imbio.2010.07.010
109. Inaba T, Ino K, Kajiyama H, Yamamoto E, Shibata K, Nawa A, et al. Role of the immunosuppressive enzyme indoleamine 2,3-dioxygenase in the progression of ovarian carcinoma. Gynecol Oncol (2009) 115(2):185–92. doi: 10.1016/j.ygyno.2009.07.015
110. Nonaka H, Saga Y, Fujiwara H, Akimoto H, Yamada A, Kagawa S, et al. Indoleamine 2,3-dioxygenase promotes peritoneal dissemination of ovarian cancer through inhibition of natural killercell function and angiogenesis promotion. Int J Oncol (2011) 38(1):113–20. doi: 10.3892/ijo_00000830
111. Tanizaki Y, Kobayashi A, Toujima S, Shiro M, Mizoguchi M, Mabuchi Y, et al. Indoleamine 2,3-dioxygenase promotes peritoneal metastasis of ovarian cancer by inducing an immunosuppressive environment. Cancer Sci (2014) 105(8):966–73. doi: 10.1111/cas.12445
112. Liu J, Zhang H, Jia L, Sun H. Effects of treg cells and IDO on human epithelial ovarian cancer cells under hypoxic conditions. Mol Med Rep (2015) 11(3):1708–14. doi: 10.3892/mmr.2014.2893
113. Zhao Y, Tao F, Jiang J, Chen L, Du J, Cheng X, et al. Tryptophan 2, 3dioxygenase promotes proliferation, migration and invasion of ovarian cancer cells. Mol Med Rep (2021) 23(6):445. doi: 10.3892/mmr.2021.12084
114. Wang X, Zhao X, Zhao J, Yang T, Zhang F, Liu L. Serum metabolite signatures of epithelial ovarian cancer based on targeted metabolomics. Clin Chim Acta (2021) 518:59–69. doi: 10.1016/j.cca.2021.03.012
115. Wu J, Li G, Li L, Li D, Dong Z, Jiang P. Asparagine enhances LCK signalling to potentiate CD8(+) T-cell activation and anti-tumour responses. Nat Cell Biol (2021) 23(1):75–86. doi: 10.1038/s41556-020-00615-4
116. Morellato AE, Umansky C, Pontel LB. The toxic side of one-carbon metabolism and epigenetics. Redox Biol (2021) 40:101850. doi: 10.1016/j.redox.2020.101850
117. Ducker GS, Rabinowitz JD. One-carbon metabolism in health and disease. Cell Metab (2017) 25(1):27–42. doi: 10.1016/j.cmet.2016.08.009
118. Sugiura A, Andrejeva G, Voss K, Heintzman DR, Xu X, Madden MZ, et al. MTHFD2 is a metabolic checkpoint controlling effector and regulatory T cell fate and function. Immunity (2022) 55(1):65–81e9. doi: 10.1016/j.immuni.2021.10.011
119. Cui X, Su H, Yang J, Wu X, Huo K, Jing X, et al. Up-regulation of MTHFD2 is associated with clinicopathological characteristics and poor survival in ovarian cancer, possibly by regulating MOB1A signaling. J Ovarian Res (2022) 15(1):23. doi: 10.1186/s13048-022-00954-w
120. Shang M, Yang H, Yang R, Chen T, Fu Y, Li Y, et al. The folate cycle enzyme MTHFD2 induces cancer immune evasion through PD-L1 up-regulation. Nat Commun (2021) 12(1):1940. doi: 10.1038/s41467-021-22173-5
121. Sinclair LV, Howden AJ, Brenes A, Spinelli L, Hukelmann JL, Macintyre AN, et al. Antigen receptor control of methionine metabolism in T cells. Elife (2019) 8:44210. doi: 10.7554/eLife.44210
122. Nunes SC, Ramos C, Santos I, Mendes C, Silva F, Vicente JB, et al. Cysteine boosts fitness under hypoxia-mimicked conditions in ovarian cancer by metabolic reprogramming. Front Cell Dev Biol (2021) 9:722412. doi: 10.3389/fcell.2021.722412
123. Gao T, Zhang X, Zhao J, Zhou F, Wang Y, Zhao Z, et al. SIK2 promotes reprogramming of glucose metabolism through PI3K/AKT/HIF-1alpha pathway and Drp1-mediated mitochondrial fission in ovarian cancer. Cancer Lett (2020) 469:89–101. doi: 10.1016/j.canlet.2019.10.029
124. Zhao J, Zhang X, Gao T, Wang S, Hou Y, Yuan P, et al. SIK2 enhances synthesis of fatty acid and cholesterol in ovarian cancer cells and tumor growth through PI3K/Akt signaling pathway. Cell Death Dis (2020) 11(1):25. doi: 10.1038/s41419-019-2221-x
125. Phillippi B, Singh M, Loftus T, Smith H, Muccioli M, Wright J, et al. Effect of laminin environments and tumor factors on the biology of myeloid dendritic cells. Immunobiology (2020) 225(1):151854. doi: 10.1016/j.imbio.2019.10.003
126. Zhang S, Wu M, Wang F. Immune regulation by CD8(+) treg cells: novel possibilities for anticancer immunotherapy. Cell Mol Immunol (2018) 15(9):805–7. doi: 10.1038/cmi.2018.170
127. Guo L, Zhou B, Liu Z, Xu Y, Lu H, Xia M, et al. Blockage of glutaminolysis enhances the sensitivity of ovarian cancer cells to PI3K/mTOR inhibition involvement of STAT3 signaling. Tumour Biol (2016) 37(8):11007–15. doi: 10.1007/s13277-016-4984-3
128. Wu M, Chen X, Lou J, Zhang S, Zhang X, Huang L, et al. TGF-beta1 contributes to CD8+ treg induction through p38 MAPK signaling in ovarian cancer microenvironment. Oncotarget (2016) 7(28):44534–44. doi: 10.18632/oncotarget.10003
129. Chen Y, Liu L, Xia L, Wu N, Wang Y, Li H, et al. TRPM7 silencing modulates glucose metabolic reprogramming to inhibit the growth of ovarian cancer by enhancing AMPK activation to promote HIF-1alpha degradation. J Exp Clin Cancer Res (2022) 41(1):44. doi: 10.1186/s13046-022-02252-1
130. Vallee A, Lecarpentier Y, Vallee JN. The key role of the WNT/beta-catenin pathway in metabolic reprogramming in cancers under normoxic conditions. Cancers (Basel) (2021) 13(21):5557. doi: 10.3390/cancers13215557
131. Liu X, Zuo X, Sun X, Tian X, Teng Y. Hexokinase 2 promotes cell proliferation and tumor formation through the wnt/beta-catenin pathway-mediated cyclin D1/c-myc upregulation in epithelial ovarian cancer. J Cancer (2022) 13(8):2559–69. doi: 10.7150/jca.71894
132. To SKY, Tang MKS, Tong Y, Zhang J, Chan KKL, Ip PPC, et al. A selective beta-Catenin-Metadherin/CEACAM1-CCL3 axis mediates metastatic heterogeneity upon tumor-macrophage interaction. Adv Sci (Weinh) (2022) 9(16):e2103230. doi: 10.1002/advs.202103230
133. Wall JA, Meza-Perez S, Scalise CB, Katre A, Londono AI, Turbitt WJ, et al. Manipulating the wnt/beta-catenin signaling pathway to promote anti-tumor immune infiltration into the TME to sensitize ovarian cancer to ICB therapy. Gynecol Oncol (2021) 160(1):285–94. doi: 10.1016/j.ygyno.2020.10.031
134. Alonezi S, Tusiimire J, Wallace J, Dufton MJ, Parkinson JA, Young LC, et al. Metabolomic profiling of the synergistic effects of melittin in combination with cisplatin on ovarian cancer cells. Metabolites (2017) 7(2):14. doi: 10.3390/metabo7020014
135. Yamawaki K, Mori Y, Sakai H, Kanda Y, Shiokawa D, Ueda H, et al. Integrative analyses of gene expression and chemosensitivity of patient-derived ovarian cancer spheroids link G6PD-driven redox metabolism to cisplatin chemoresistance. Cancer Lett (2021) 521:29–38. doi: 10.1016/j.canlet.2021.08.018
136. Lu J, Li Y, Li YA, Wang L, Zeng AR, Ma XL, et al. In vivo detection of dysregulated choline metabolism in paclitaxel-resistant ovarian cancers with proton magnetic resonance spectroscopy. J Transl Med (2022) 20(1):92. doi: 10.1186/s12967-022-03292-z
137. Odunsi K, Qian F, Lugade AA, Yu H, Geller MA, Fling SP, et al. Metabolic adaptation of ovarian tumors in patients treated with an IDO1 inhibitor constrains antitumor immune responses. Sci Transl Med (2022) 14(636):eabg8402. doi: 10.1126/scitranslmed.abg8402
138. Shi Q, Shen Q, Liu Y, Shi Y, Huang W, Wang X, et al. Increased glucose metabolism in TAMs fuels O-GlcNAcylation of lysosomal cathepsin b to promote cancer metastasis and chemoresistance. Cancer Cell (2022), S1535-6108(22)00376-2. doi: 10.1016/j.ccell.2022.08.012
139. Alharbi M, Lai A, Sharma S, Kalita-de Croft P, Godbole N, Campos A, et al. Extracellular vesicle transmission of chemoresistance to ovarian cancer cells is associated with hypoxia-induced expression of glycolytic pathway proteins, and prediction of epithelial ovarian cancer disease recurrence. Cancers (Basel) (2021) 13(14):3388. doi: 10.3390/cancers13143388
140. Purwaha P, Lorenzi PL, Silva LP, Hawke DH, Weinstein JN. Targeted metabolomic analysis of amino acid response to l-asparaginase in adherent cells. Metabolomics (2014) 10(5):909–19. doi: 10.1007/s11306-014-0634-1
141. Wang L, Li Y, Wang J. Effect of glutamine on the immune function of paclitaxel intervention in ovarian cancer mice. Cell Mol Biol (Noisy-le-grand) (2020) 66(2):193–7. doi: 10.14715/cmb/2020.66.2.30
142. Priebe A, Tan L, Wahl H, Kueck A, He G, Kwok R, et al. Glucose deprivation activates AMPK and induces cell death through modulation of akt in ovarian cancer cells. Gynecol Oncol (2011) 122(2):389–95. doi: 10.1016/j.ygyno.2011.04.024
143. El-Mais N, Fakhoury I, Abdellatef S, Abi-Habib R, El-Sibai M. Human recombinant arginase I [HuArgI (Co)-PEG5000]-induced arginine depletion inhibits ovarian cancer cell adhesion and migration through autophagy-mediated inhibition of RhoA. J Ovarian Res (2021) 14(1):13. doi: 10.1186/s13048-021-00767-3
144. Cheng H, Ma R, Wang S, Wang Y, Li Y, Tang Z, et al. Preliminary safety and potential effect of 6B11-OCIK adoptive cell therapy against platinum-resistant recurrent or refractory ovarian cancer. Front Immunol (2021) 12:707468. doi: 10.3389/fimmu.2021.707468
145. Amobi-McCloud A, Muthuswamy R, Battaglia S, Yu H, Liu T, Wang J, et al. IDO1 expression in ovarian cancer induces PD-1 in T cells via aryl hydrocarbon receptor activation. Front Immunol (2021) 12:678999. doi: 10.3389/fimmu.2021.678999
146. Jung KH, LoRusso P, Burris H, Gordon M, Bang YJ, Hellmann MD, et al. Phase I study of the indoleamine 2,3-dioxygenase 1 (IDO1) inhibitor navoximod (GDC-0919) administered with PD-L1 inhibitor (Atezolizumab) in advanced solid tumors. Clin Cancer Res (2019) 25(11):3220–8. doi: 10.1158/1078-0432.CCR-18-2740
147. Wang JJ, Siu MK, Jiang YX, Leung TH, Chan DW, Wang HG, et al. A combination of glutaminase inhibitor 968 and PD-L1 blockade boosts the immune response against ovarian cancer. Biomolecules (2021) 11(12):1749. doi: 10.3390/biom11121749
148. Fucikova J, Hensler M, Kasikova L, Lanickova T, Pasulka J, Rakova J, et al. An autologous dendritic cell vaccine promotes anticancer immunity in patients with ovarian cancer with low mutational burden and cold tumors. Clin Cancer Res (2022) 28(14):3053–65. doi: 10.1158/1078-0432.CCR-21-4413
149. Rob L, Cibula D, Knapp P, Mallmann P, Klat J, Minar L, et al. Safety and efficacy of dendritic cell-based immunotherapy DCVAC/OvCa added to first-line chemotherapy (carboplatin plus paclitaxel) for epithelial ovarian cancer: A phase 2, open-label, multicenter, randomized trial. J Immunother Cancer (2022) 10(1):e003190. doi: 10.1136/jitc-2021-003190
150. Block MS, Dietz AB, Gustafson MP, Kalli KR, Erskine CL, Youssef B, et al. Th17-inducing autologous dendritic cell vaccination promotes antigen-specific cellular and humoral immunity in ovarian cancer patients. Nat Commun (2020) 11(1):5173. doi: 10.1038/s41467-020-18962-z
151. Scherwitzl I, Opp S, Hurtado AM, Pampeno C, Loomis C, Kannan K, et al. Sindbis virus with anti-OX40 overcomes the immunosuppressive tumor microenvironment of low-immunogenic tumors. Mol Ther Oncolytics (2020) 17:431–47. doi: 10.1016/j.omto.2020.04.012
152. Yu J, Wei Z, Li Q, Wan F, Chao Z, Zhang X, et al. Advanced cancer starvation therapy by simultaneous deprivation of lactate and glucose using a MOF nanoplatform. Adv Sci (Weinh) (2021) 8(19):e2101467. doi: 10.1002/advs.202101467
153. Prentice RL, Thomson CA, Caan B, Hubbell FA, Anderson GL, Beresford SA, et al. Low-fat dietary pattern and cancer incidence in the women's health initiative dietary modification randomized controlled trial. J Natl Cancer Inst (2007) 99(20):1534–43. doi: 10.1093/jnci/djm159
154. Zhang M, Lee AH, Binns CW. Reproductive and dietary risk factors for epithelial ovarian cancer in China. Gynecol Oncol (2004) 92(1):320–6. doi: 10.1016/j.ygyno.2003.10.025
155. Cozen W, Peters R, Reichardt JK, Ng W, Felix JC, Wan P, et al. Galactose-1-phosphate uridyl transferase (GALT) genotype and phenotype, galactose consumption, and the risk of borderline and invasive ovarian cancer (United states). Cancer Causes Control (2002) 13(2):113–20. doi: 10.1023/a:1014384027523
156. Fan J, Shang D, Han B, Song J, Chen H, Yang JM. Adoptive cell transfer: Is it a promising immunotherapy for colorectal cancer? Theranostics (2018) 8(20):5784–800. doi: 10.7150/thno.29035
157. Geller MA, Cooley S, Judson PL, Ghebre R, Carson LF, Argenta PA, et al. A phase II study of allogeneic natural killer cell therapy to treat patients with recurrent ovarian and breast cancer. Cytotherapy (2011) 13(1):98–107. doi: 10.3109/14653249.2010.515582
158. Hoogstad-van Evert J, Bekkers R, Ottevanger N, Schaap N, Hobo W, Jansen JH, et al. Intraperitoneal infusion of ex vivo-cultured allogeneic NK cells in recurrent ovarian carcinoma patients (a phase I study). Med (Baltimore) (2019) 98(5):e14290. doi: 10.1097/MD.0000000000014290
159. Lu YC, Shi YY, Luo ZY, Guo XM, Jiang MS, Li X, et al. Reactivation of dysfunctional dendritic cells by a stress-relieving nanosystem resets anti-tumor immune landscape. Nano Today (2022) 43:101416. doi: 10.1016/j.nantod.2022.101416
160. Benesova K, Kraus FV, Carvalho RA, Lorenz H, Horth CH, Gunther J, et al. Distinct immune-effector and metabolic profile of CD8(+) T cells in patients with autoimmune polyarthritis induced by therapy with immune checkpoint inhibitors. Ann Rheumatic Dis (2022) 43:101416. doi: 10.1136/ard-2022-222451
161. Liu Y, Yu Y, Yang S, Zeng B, Zhang Z, Jiao G, et al. Regulation of arginase I activity and expression by both PD-1 and CTLA-4 on the myeloid-derived suppressor cells. Cancer Immunol Immunother (2009) 58(5):687–97. doi: 10.1007/s00262-008-0591-5
162. Awuah SG, Zheng YR, Bruno PM, Hemann MT, Lippard SJ. A Pt(IV) pro-drug preferentially targets indoleamine-2,3-dioxygenase, providing enhanced ovarian cancer immuno-chemotherapy. J Am Chem Soc (2015) 137(47):14854–7. doi: 10.1021/jacs.5b10182
163. Kuznicki ML, Bennett C, Yao M, Joehlin-Price A, Rose PG, Mahdi H. Predictors of response to immune checkpoint inhibition in a real world gynecologic cancer population. Gynecol Oncol Rep (2020) 34:100671. doi: 10.1016/j.gore.2020.100671
164. Sadik A, Somarribas Patterson LF, Ozturk S, Mohapatra SR, Panitz V, Secker PF, et al. IL4I1 is a metabolic immune checkpoint that activates the AHR and promotes tumor progression. Cell (2020) 182(5):1252–1270e34. doi: 10.1016/j.cell.2020.07.038
165. Wallace-Povirk A, Rubinsak L, Malysa A, Dzinic SH, Ravindra M, Schneider M, et al. Targeted therapy of pyrrolo[2,3-d]pyrimidine antifolates in a syngeneic mouse model of high grade serous ovarian cancer and the impact on the tumor microenvironment. Sci Rep (2022) 12(1):11346. doi: 10.1038/s41598-022-14788-5
166. Hou Z, Gattoc L, O'Connor C, Yang S, Wallace-Povirk A, George C, et al. Dual targeting of epithelial ovarian cancer Via folate receptor alpha and the proton-coupled folate transporter with 6-substituted Pyrrolo[2,3-d]pyrimidine antifolates. Mol Cancer Ther (2017) 16(5):819–30. doi: 10.1158/1535-7163.MCT-16-0444
167. Li L, Liu S, Han D, Tang B, Ma J. Delivery and biosafety of oncolytic virotherapy. Front Oncol (2020) 10:475. doi: 10.3389/fonc.2020.00475
168. Hulin-Curtis SL, Davies JA, Nestic D, Bates EA, Baker AT, Cunliffe TG, et al. Identification of folate receptor alpha (FRalpha) binding oligopeptides and their evaluation for targeted virotherapy applications. Cancer Gene Ther (2020) 27(10-11):785–98. doi: 10.1038/s41417-019-0156-0
169. Sun J, Yan C, Xu D, Zhang Z, Li K, Li X, et al. Immuno-genomic characterisation of high-grade serous ovarian cancer reveals immune evasion mechanisms and identifies an immunological subtype with a favourable prognosis and improved therapeutic efficacy. Br J Cancer (2022) 126(11):1570–80. doi: 10.1038/s41416-021-01692-4
170. Sun L, Zhang H, Gao P. Metabolic reprogramming and epigenetic modifications on the path to cancer. Protein Cell (2022) 13(12):877–919. doi: 10.1007/s13238-021-00846-7
171. Sun T, Liu Z, Yang Q. The role of ubiquitination and deubiquitination in cancer metabolism. Mol Cancer (2020) 19(1):146. doi: 10.1186/s12943-020-01262-x
172. Zhao E, Maj T, Kryczek I, Li W, Wu K, Zhao L, et al. Cancer mediates effector T cell dysfunction by targeting microRNAs and EZH2 via glycolysis restriction. Nat Immunol (2016) 17(1):95–103. doi: 10.1038/ni.3313
Keywords: ovarian cancer, tumor immune microenvironment, metabolic reprogramming, metabolism, immunotherapy
Citation: Lin Y, Zhou X, Ni Y, Zhao X and Liang X (2022) Metabolic reprogramming of the tumor immune microenvironment in ovarian cancer: A novel orientation for immunotherapy. Front. Immunol. 13:1030831. doi: 10.3389/fimmu.2022.1030831
Received: 29 August 2022; Accepted: 29 September 2022;
Published: 14 October 2022.
Edited by:
Xuyu Gu, Southeast University, ChinaReviewed by:
Jie Tang, The University of Queensland, AustraliaYiqing Zhao, Case Western Reserve University, United States
Copyright © 2022 Lin, Zhou, Ni, Zhao and Liang. This is an open-access article distributed under the terms of the Creative Commons Attribution License (CC BY). The use, distribution or reproduction in other forums is permitted, provided the original author(s) and the copyright owner(s) are credited and that the original publication in this journal is cited, in accordance with accepted academic practice. No use, distribution or reproduction is permitted which does not comply with these terms.
*Correspondence: Xiao Liang, xiaoliang9101@163.com