Erratum: Mechanisms of extracellular vesicle-mediated immune evasion in melanoma
- Institute of Pharmaceutical Sciences, Swiss Federal Institute of Technology (ETH) Zurich, Zurich, Switzerland
Melanoma-derived extracellular vesicles (EVs) have been found to promote tumor growth and progression, and to predict patient responsiveness to immunotherapy. Consequently, EVs have been implicated in tumor immune evasion, and multiple studies reported immune-regulatory activities of melanoma EVs in vitro and in vivo. This review highlights mechanistic insights in EV-mediated regulation of various immune cell types, including effects on inflammatory, apoptotic, stress-sensing and immune checkpoint pathways as well as antigen-dependent responses. Additionally, current challenges in the field are discussed that need to be overcome to determine the clinical relevance of these various mechanisms and to develop corresponding therapeutic approaches to promote tumor immunity and immunotherapy responsiveness in melanoma patients in the future.
Introduction
Extracellular vesicles (EVs) are membrane-encapsulated, subcellular particles that are released by virtually every cell type and have been ascribed various biologic functions, ranging from waste disposal to molecular cell-to-cell communication (1). Traditionally, EVs have been classified based on the membrane of origin and/or the mode of EV generation, including exosomes derived from endosomal membranes, microvesicles derived from the plasma membrane, and apoptotic bodies (2). However, due to difficulties to specifically isolate and distinguish between these EV subsets, a classification based on measurable, physical parameters (e.g. size, density) has been proposed (3).
Melanoma is an aggressive cancer type prone to invade and metastasize, and melanoma-derived EVs have been implicated in progression (4). EV plasma levels in melanoma patients are increased compared to healthy individuals (5), and plasma EV signatures correlate with immunotherapy outcome (6–8), suggesting that melanoma-derived EVs might affect tumor immunity. Congruently, inhibition and activation of various immune cell types by melanoma EVs have been described (9). In this review, I focus specifically on mechanisms of EV-mediated immune regulation, such as innate immune-regulatory pathways, immune checkpoints, as well as transfer of tumor antigens and other immune-regulatory cargoes (Figure 1). Furthermore, current challenges in the field, including variability in experimental procedures, in vitro experiments failing to reflect natural EV biodistribution, and a lack of mechanistic in vivo studies are discussed.
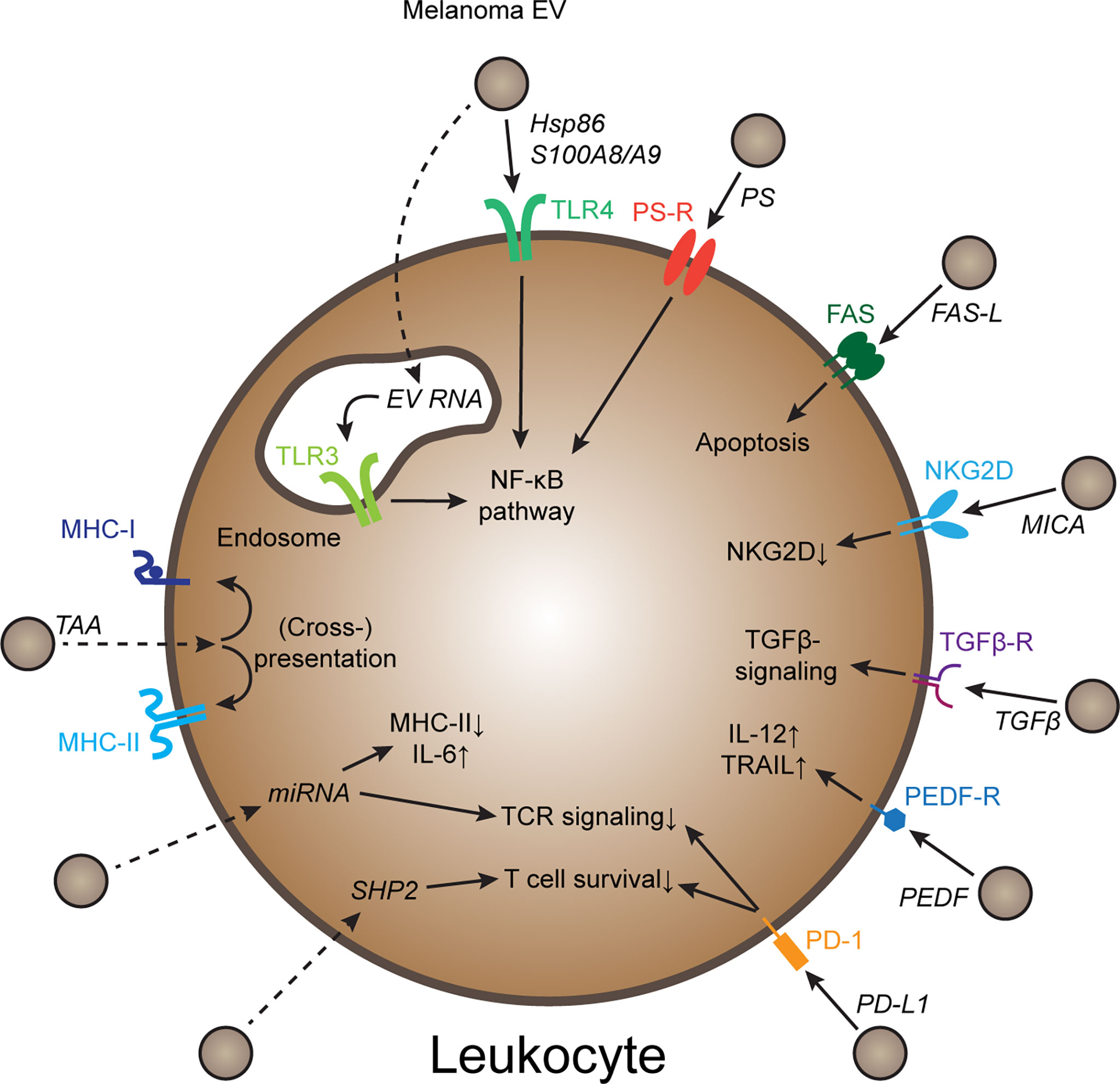
Figure 1 Mechanisms of melanoma EV-mediated immune cell regulation Schematic representation of several molecular mechanisms how melanoma-derived EVs can regulate immune cell behavior. Further explanations in the text. Dotted arrows indicate pathways that require EV uptake and release of EV cargo. PS, Phosphatidyl-serine; PS-R, PS-receptor; TAA, tumor-associated antigen; PEDF, pigment epithelium derived factor.
EV-mediated effects on the nuclear factor κ B (NF-κB) pathway
The NF-κB pathway is a highly conserved signal transduction pathway triggered by danger signals and inflammatory cytokines and regulating inflammatory responses in virtually every cell type. In melanoma, NF-κB activation has been linked to tumor initiation, progression and inflammation affecting tumor immunity and cancer stemness (10–12). Interestingly, several studies suggested that melanoma-derived EVs can de-regulate NF-κB signaling in immune cells. For example, EVs derived from the mouse melanoma cell lines B16F1 and B16F10 could activate NF-κB in RAW264.7 and primary macrophages, altering the release of inflammatory cytokines and chemokines (13, 14). More recently, melanoma-derived EVs isolated from plasma of melanoma patients using CSPG4-binding antibodies were shown to activate NF-κB in autologous, peripheral CD8+ T cells, while pharmacological NF-κB inhibition could reverse EV-mediated suppression of T cell proliferation (15). In contrast, Luong et al. found that melanoma-derived EVs induce SOCS3, a negative regulator of the NF-κB pathway, in bone marrow-derived monocytes (16). However, this effect was observed after prolonged stimulation (4 days) of monocytes. Thus, SOCS3 upregulation might have been due to a negative feedback response towards increased NF-κB activation.
Exactly how melanoma EVs trigger NF-κB activation in immune cells is not completely understood, but several pathways have been implied. For instance, EVs derived from human melanoma cell lines have been found to inhibit dendritic cell (DC) maturation via S100A8 and S100A9 proteins, which signal via TLR4, among others (17). TLR4 also mediated melanoma EV-induced upregulation of PD-L1 in immature myeloid cells via EV surface-associated Hsp86 (18). Furthermore, mouse melanoma cell-derived EVs could trigger TLR3 in bone marrow-derived DCs (19). Another potential mechanism how melanoma-derived EVs could affect NF-κB is via phosphatidyl-serine (PS). PS is usually excluded from the outer face of the plasma membrane but can be exposed on the surface of apoptotic cells and EVs. Importantly, PS has broad immune-inhibitory and tolerogenic functions, in part via regulation of NF-κB (20). Blockade of EV PS ameliorated TGF-β1 induction by B16F10-derived EVs in peritoneal macrophages (21) as well as the inhibition of primary human T cells by EVs derived from a melanoma xenograft (22). In contrast, EV PS has been reported to bind CD300 in bone-marrow derived DCs, preventing their activation of TLR3 (19).
Melanoma EVs trigger apoptotic pathways
Melanoma-derived EVs might also impair tumor immunity by inducing apoptosis in immune effector cells. EVs isolated from human melanoma cell lines and from the serum of melanoma patients were shown to contain FasL and to induce apoptosis of patient-derived, melanoma-specific CD8+ T cells (23). In a follow-up study, melanoma EVs isolated from plasma of melanoma patients again consistently contained FasL as well as TRAIL, and FasL-blocking antibodies partially reduced EV-induced T cell apoptosis in vitro (15).
NK-activating receptors: NKG2D and NKp30
NKG2D is an activating receptor expressed by NK cells and subsets of CD8+ T cells recognizing stress signals such as MICA and MICB on the surface of target cells. Secreted MICA on the other hand impairs NK and CD8+ T cell responses, and a human melanoma cell line expressing the MICA allele *008 has been shown to release MICA in association with EVs (24). MICA (but not MICB) was also present in melanoma EVs isolated from plasma of melanoma patients and these EVs downregulated NKG2D in primary human NK cells, suggesting functional impairment (15).
Activation of the innate pattern recognition receptor RIG-1 in human melanoma cell lines induced the release of EVs enriched for BAG6, a ligand for NKp30, another NK-activating receptor. In this case however, RIG-1-induced EVs activated the cytotoxicity of human NK cells in vitro and impaired primary tumor growth in a mouse model of melanoma (HCMel12) (25). This effect was not mediated by NKp30 though, since this receptor is not functional in mice (26). Instead, BAG6 promotes generation and cargo-loading of immune-stimulatory EVs released by stressed melanoma cells (27), thus explaining their immune-stimulatory function in mice lacking functional NKp30.
EV-associated cytokines
Transforming Growth Factor β (TGF-β) is a pleiotropic cytokine that can inhibit tumor immunity in melanoma (28). Whether or not TGF-β acts at least partially via EVs is controversial. On the one hand, TGF-β was associated with EVs derived from the human melanoma cell line A375, and melanoma EV-induced inhibition of DC maturation could be reversed using TGF-β-blocking antibodies (29). On the other hand, melanoma EVs isolated from patient plasma were not enriched for TGF-β compared to non-melanoma EVs derived from the same patients, while EV-mediated suppression of T cell proliferation in vitro could still be blocked by anti-TGF-β (15). Thus, further studies are necessary to establish whether (respectively how) TGF-β is associated with melanoma EV surfaces, or whether it is induced de novo in recipient cells of melanoma EVs and thereby inhibits immune responses (21).
Interestingly, EVs derived from non-metastatic melanoma cell lines and plasma of patients with non-metastatic melanoma have been found to activate patrolling monocytes, macrophages, and NK cells and thereby to suppress melanoma lung metastasis in vivo. This effect was mediated by pigment epithelium-derived factor (PEDF), a secreted cytokine that was found to be associated with melanoma EV surfaces (30).
Immune checkpoints: Do melanoma EVs inhibit T cells via PD-L1?
Immune checkpoints such as the CD80/86-CTLA and the PD-L1-PD-1 axes have recently gained much attention, since their therapeutic targeting elicits immune responses, particularly in melanoma that is characterized by a high mutation rate and occurrence of tumor (neo-) antigens. In melanoma, PD-L1 is expressed by immune, stromal, and tumor cells, enabling them to evade attacks by tumor antigen-specific CD8+ T cells in the tumor microenvironment. Importantly, several studies have shown that melanoma cells also release functional PD-L1 via EVs, potentially inhibiting T cells beyond the local microenvironment. Wei Guo’s team found surface PD-L1 on EVs isolated from several human and mouse melanoma cell lines, with higher levels in metastatic cell lines compared to non-metastatic ones (31). Furthermore, increased levels of PD-L1+ EVs could be detected in the plasma of patients with metastatic melanoma compared to healthy donors (31, 32). Functionally, EVs from melanoma cell lines could inhibit proliferation and cytotoxicity of CD8+ T cells in vitro in a PD-L1- (or PD-1-) dependent manner (15, 31, 32). Subsequently, this mechanism of EV-mediated T cell suppression was shown to depend on ICAM-1 on the EV surface (33). In the B16F10 model, melanoma EVs furthermore promoted primary tumor growth and systemically inhibited CD8+ T cell proliferation in vivo (31). Similar observations have been made using the B16F10 tail vein model of lung metastasis (32).
Somewhat contradictory to the above-mentioned studies, other groups failed to detect significant PD-L1 levels on melanoma-derived EVs. For instance, PD-L1 was neither detectable in melanoma cell line- and melanoma patient-derived EVs using mass spectrometry (34–36) nor was it enriched in melanoma-derived EVs compared to non-melanoma EVs isolated from the plasma of melanoma patients using a flow cytometry-based approach (15). Thus, the amount of PD-L1 in melanoma EVs might be low. Furthermore, PD-L1 blockade could not revert melanoma EV-mediated inhibition of cytokine production in patient-derived, NY-ESO-1-specific T cells unless it was combined with IL-10 blockade (37). Thus, the relevance of EV-associated PD-L1 compared to cellular PD-L1 for melanoma immunity and immunotherapy responsiveness is not entirely clear yet.
Horizontal transfer of immune-modulatory cargo
Apart from ligands on the EV surface that trigger immune-regulatory receptors upon contact with or uptake by their recipient cells, EVs carry complex payloads including cytosolic proteins and nucleic acids such as miRNA, which they can transfer from one cell to another. Especially EV-mediated transfer of miRNAs has been studied to considerable extent, and there are reports that in the case of melanoma, this phenomenon can contribute to tumor-associated immune inhibition. Huber et al. identified several miRNAs (miR100, miR125b, miR146a and miR155), that, when transferred by melanoma EVs to monocytes, induced the conversion of monocytes into myeloid-derived suppressor cells (MDSCs), resulting in the downregulation of MHC-II and concomitant upregulation of IL-6 and CCL2 (38). More recently, Vignard and colleagues identified another set of miRNAs in human melanoma cell line-derived EVs that could downregulate TCR responses and effector functions in CD8+ T cells in vitro (39).
Another potential immune-regulatory cargo of melanoma EVs is SHP2. Wu et al. reported that EVs derived from mouse B16F0 melanoma cells contained both SHP2 protein and mRNA, and could transfer it to primary CD8+ T cells resulting in reduced T cell viability upon stimulation. However, it remained unclear whether this effect was due to mRNA or protein transfer, and significant SHP2 induction required rather high concentrations of EVs in vitro (40).
EVs and tumor antigens
EVs contain a large variety of proteins derived from their donor cells, including potential tumor-associated antigens, which, if transferred to appropriate recipient cells, can be presented to the immune system. Indeed, more than two decades ago, it was shown that EVs derived from melanoma cell lines as well as malignant effusions of patients with metastatic melanoma contain classic melanoma antigens such as gp100 and Tyrp2 (41, 42). Subsequently, other studies confirmed these findings in human and mouse melanoma cell lines (23, 43). In addition, peptides derived from melanoma antigens might be transferred directly by EVs in association with MHC-I molecules (29, 44).
When transferred to immune-stimulatory antigen-presenting cells (APCs) such as mature DCs, melanoma EVs can induce tumor antigen presentation and T cell activation (41). However, if melanoma EVs deliver their cargo to tolerogenic APCs such as immature DCs, a state of “tumor tolerance” might ensue (45). Notably, melanoma EVs have been shown to inhibit DC maturation as discussed above (17, 29). Another tolergenic type of APC are lymphatic endothelial cells (LECs), particularly those lining lymph node (LN) sinuses. Previously, LN LECs have been shown to present self-antigens such as tyrosinase (which is also highly expressed in many melanomas) and inhibit T cells specific for it, possibly via PD-L1 which is constitutively expressed by LN LECs (46). We recently found that lymphatic PD-L1 can also impair tumor-specific T cell responses in melanoma-draining LNs (47), and that in the B16F10 melanoma model, EVs can transfer a model antigen from primary tumor cells to draining LN LECs, resulting in impaired CD8+ T cell responses (36). Thus, depending on the recipient cell type and state, melanoma EV-mediated transfer of tumor antigens can have either immune-stimulatory or tolerogenic effects.
Location, location, location…
The majority of the studies discussed above investigated mechanisms of EV-mediated immune regulation using highly reductionistic in vitro systems, typically co-culturing immune cells directly with EVs isolated from cell culture or plasma samples. Although these approaches are useful to explore the overall immune-regulatory capacity of EVs, they do not reflect the aspect of EV biodistribution in vivo, which is very important regarding the question which immune cell types (and in which functional state) are most likely affected by melanoma EVs in vivo.
Within a growing melanoma, malignant cells release EVs into the surrounding interstitial space, enabling them to interact with tumor-infiltrating leukocytes in a paracrine manner. Melanoma EVs are also present in the blood, implying that they may affect immune responses systemically. Additionally, there is considerable evidence that melanoma EVs can be taken up by tumor-associated lymphatic vessels and are transported to draining LNs, important sites not only for early melanoma metastasis but also for the initiation (and inhibition) of tumor-specific immune responses (48, 49). In fact, initial lymphatic vessels, in contrast to blood vessels, are very permeable for particles up to 100-200 nm in diameter due to a specific junctional organization (“Button-like junctions”) between adjacent LECs. Furthermore, interstitial fluid dynamics facilitate EV transport towards lymphatic vessels within or around the tumor site (49). Indeed, in a seminal study in 2011, Joshua Hood and colleagues found that after interstitial injection, fluorescently labeled B16F10-derived EVs specifically and efficiently homed to draining LNs where they accumulated (50). Later on, several other groups confirmed efficient transport of both injected EVs as well as EVs released endogenously from primary B16 melanomas, to draining LNs in mice (34, 36, 51, 52). Using EV-associated luciferase activity for quantification, one of these studies furthermore found that the relative accumulation of melanoma-derived EVs was much higher in draining LNs compared to any other tissue in the body, including the blood (51). Similarly, melanoma-derived EVs were enriched in lymph-rich wound exudate collected after lymphadenectomy in melanoma patients in comparison to plasma, and lymph-borne EVs were larger than those from plasma, suggesting qualitative (and potentially, functional) differences between them (34, 35, 53).
Prominent uptake of melanoma EVs in vivo was noted especially in macrophages in the tumor microenvironment and in tumor-draining LNs (36, 51, 52), and this uptake has been suggested to prevent tumor growth-promoting B cell responses (51). While none of these studies found a significant uptake of melanoma EVs by other immune cell types, including DCs, NK cells, and T cells, melanoma EVs might still interact with them and affect their phenotype or function via cellular receptors as discussed above. Another cell type with a strong capacity to take up EVs are LN-resident LECs, and this uptake appears to depend on EV-associated integrins (36, 52). Recent advances in single-cell RNA sequencing allowed the identification of LN LEC subsets in human and mice (54, 55), and it were particularly LECs lining the floor of the subcapsular sinus that took up melanoma EVs (36), consistent with EV transport to LNs via afferent lymphatic vessels. In conclusion, melanoma derived EVs appear to have profound effects on tumor-draining LNs, primarily interacting with macrophages and LECs and thereby regulating adaptive T- and B-cell responses indirectly.
Discussion
Clearly, melanoma-derived EVs have broad immune-regulatory capacities, triggering innate and checkpoint receptors in immune cells, transferring immune-modulatory cargoes including miRNAs, and delivering melanoma antigens to APCs, all of which may ultimately affect adaptive, tumor-specific immunity and immunotherapy responsiveness (Figure 1). However, there are considerable discrepancies between individual studies, both regarding the reported mechanisms as well as the direction of EV-mediated immune regulation. These divergent results are at least in part due to differences in the starting material (cell lines, individual patient samples) and experimental approaches such as EV isolation methods (resulting in varying EV subtypes and co-contaminants), EV dosing and choice of controls (e.g. empty liposomes or normal melanocyte-derived EVs). While there are efforts in the fields to standardize methods and quality controls (3), it will be an important challenge for future EV functional studies to identify experimental set-ups and conditions that yield the most clinically relevant results.
One important although experimentally challenging aspect in this regard is to validate in vitro observations of EV-mediated immune cell regulation using appropriate in vivo systems, particularly those reflecting endogenous EV release from a growing tumor. Those systems most faithfully represent “natural” EV doses, biodistribution, access to different tissues and immune cells within, and interplay between varying immune and stromal cells in response to melanoma-derived EVs. Also, patterns of cellular uptake of EVs are strikingly different in vitro and in vivo. However, such systems require elaborate approaches to allow tracking and modulation of endogenously secreted EVs, for instance using genetic EV tags and methods to modify EV (subset) release and/or composition in vivo. In the long run, such functional studies will be key to develop novel therapeutic approaches, targeting the release, uptake or effector functions of melanoma EVs, which could ultimately enhance endogenous as well as immunotherapy-induced immune responses in melanoma patients, for instance in unresectable disease or in a neo-adjuvant setting.
Author contributions
LD wrote the manuscript and prepared the artwork. The author confirms being the sole contributor of this work and has approved it for publication.
Funding
LD has been supported by grants from ETH Zurich, Krebsliga Zurich, and the Vontobel Foundation. Open access funding was provided by ETH Zurich.
Conflict of interest
The author declares that the research was conducted in the absence of any commercial or financial relationships that could be construed as a potential conflict of interest.
Publisher’s note
All claims expressed in this article are solely those of the authors and do not necessarily represent those of their affiliated organizations, or those of the publisher, the editors and the reviewers. Any product that may be evaluated in this article, or claim that may be made by its manufacturer, is not guaranteed or endorsed by the publisher.
References
1. Couch Y, Buzas EI, Di Vizio D, Gho YS, Harrison P, Hill AF, et al. A brief history of nearly EV-erything - the rise and rise of extracellular vesicles. J Extracell Vesicles (2021) 10(14):e12144. doi: 10.1002/jev2.12144
2. Raposo G, Stoorvogel W. Extracellular vesicles: Exosomes, microvesicles, and friends. J Cell Biol (2013) 200(4):373–83. doi: 10.1083/jcb.201211138
3. Thery C, Witwer KW, Aikawa E, Alcaraz MJ, Anderson JD, Andriantsitohaina R, et al. Minimal information for studies of extracellular vesicles 2018 (MISEV2018): A position statement of the international society for extracellular vesicles and update of the MISEV2014 guidelines. J Extracell Vesicles (2018) 7(1):1535750. doi: 10.1080/20013078.2018.1535750
4. Lattmann E, Levesque MP. The role of extracellular vesicles in melanoma progression. Cancers (Basel) (2022) 14(13):3086. doi: 10.3390/cancers14133086
5. Logozzi M, De Milito A, Lugini L, Borghi M, Calabro L, Spada M, et al. High levels of exosomes expressing CD63 and caveolin-1 in plasma of melanoma patients. PLoS One (2009) 4(4):e5219. doi: 10.1371/journal.pone.0005219
6. Shi A, Kasumova GG, Michaud WA, Cintolo-Gonzalez J, Diaz-Martinez M, Ohmura J, et al. Plasma-derived extracellular vesicle analysis and deconvolution enable prediction and tracking of melanoma checkpoint blockade outcome. Sci Adv (2020) 6(46):eabb3461. doi: 10.1126/sciadv.abb3461
7. Porcelli L, Guida M, De Summa S, Di Fonte R, De Risi I, Garofoli M, et al. uPAR(+) extracellular vesicles: a robust biomarker of resistance to checkpoint inhibitor immunotherapy in metastatic melanoma patients. J Immunother Cancer (2021) 9(5):e002372. doi: 10.1136/jitc-2021-002372
8. Serrati S, Guida M, Di Fonte R, De Summa S, Strippoli S, Iacobazzi RM, et al. Circulating extracellular vesicles expressing PD1 and PD-L1 predict response and mediate resistance to checkpoint inhibitors immunotherapy in metastatic melanoma. Mol Cancer (2022) 21(1):20. doi: 10.1186/s12943-021-01490-9
9. Pretti MAM, Bernardes SS, da Cruz JGV, Boroni M, Possik PA. Extracellular vesicle-mediated crosstalk between melanoma and the immune system: Impact on tumor progression and therapy response. J Leukoc Biol (2020) 108(4):1101–15. doi: 10.1002/JLB.3MR0320-644R
10. Amiri KI, Richmond A. Role of nuclear factor-kappa b in melanoma. Cancer Metastasis Rev (2005) 24(2):301–13. doi: 10.1007/s10555-005-1579-7
11. Ueda Y, Richmond A. NF-kappaB activation in melanoma. Pigment Cell Res (2006) 19(2):112–24. doi: 10.1111/j.1600-0749.2006.00304.x
12. Lim MS, Wang JH, Power DG, Redmond HP. Inflammatory regulation of stem-like cells in melanoma. Melanoma Res (2017) 27(1):1–7. doi: 10.1097/CMR.0000000000000303
13. Marton A, Vizler C, Kusz E, Temesfoi V, Szathmary Z, Nagy K, et al. Melanoma cell-derived exosomes alter macrophage and dendritic cell functions in vitro. Immunol Lett (2012) 148(1):34–8. doi: 10.1016/j.imlet.2012.07.006
14. Bardi GT, Smith MA, Hood JL. Melanoma exosomes promote mixed M1 and M2 macrophage polarization. Cytokine (2018) 105:63–72. doi: 10.1016/j.cyto.2018.02.002
15. Sharma P, Diergaarde B, Ferrone S, Kirkwood JM, Whiteside TL. Melanoma cell-derived exosomes in plasma of melanoma patients suppress functions of immune effector cells. Sci Rep (2020) 10(1):92. doi: 10.1038/s41598-019-56542-4
16. Luong N, Lenz JA, Modiano JF, Olson JK. Extracellular vesicles secreted by tumor cells promote the generation of suppressive monocytes. Immunohorizons (2021) 5(8):647–58. doi: 10.4049/immunohorizons.2000017
17. Maus RLG, Jakub JW, Nevala WK, Christensen TA, Noble-Orcutt K, Sachs Z, et al. Human melanoma-derived extracellular vesicles regulate dendritic cell maturation. Front Immunol (2017) 8:358. doi: 10.3389/fimmu.2017.00358
18. Fleming V, Hu X, Weller C, Weber R, Groth C, Riester Z, et al. Melanoma extracellular vesicles generate immunosuppressive myeloid cells by upregulating PD-L1 via TLR4 signaling. Cancer Res (2019) 79(18):4715–28. doi: 10.1158/0008-5472.CAN-19-0053
19. Nakazawa Y, Nishiyama N, Koizumi H, Kanemaru K, Nakahashi-Oda C, Shibuya A. Tumor-derived extracellular vesicles regulate tumor-infiltrating regulatory T cells via the inhibitory immunoreceptor CD300a. Elife (2021) 10:e61999. doi: 10.7554/eLife.61999
20. Birge RB, Boeltz S, Kumar S, Carlson J, Wanderley J, Calianese D, et al. Phosphatidylserine is a global immunosuppressive signal in efferocytosis, infectious disease, and cancer. Cell Death Differ (2016) 23(6):962–78. doi: 10.1038/cdd.2016.11
21. Lima LG, Chammas R, Monteiro RQ, Moreira ME, Barcinski MA. Tumor-derived microvesicles modulate the establishment of metastatic melanoma in a phosphatidylserine-dependent manner. Cancer Lett (2009) 283(2):168–75. doi: 10.1016/j.canlet.2009.03.041
22. Bhatta M, Shenoy GN, Loyall JL, Gray BD, Bapardekar M, Conway A, et al. Novel phosphatidylserine-binding molecule enhances antitumor T-cell responses by targeting immunosuppressive exosomes in human tumor microenvironments. J Immunother Cancer (2021) 9(10):e003148. doi: 10.1136/jitc-2021-003148
23. Wieckowski EU, Visus C, Szajnik M, Szczepanski MJ, Storkus WJ, Whiteside TL. Tumor-derived microvesicles promote regulatory T cell expansion and induce apoptosis in tumor-reactive activated CD8+ T lymphocytes. J Immunol (2009) 183(6):3720–30. doi: 10.4049/jimmunol.0900970
24. Ashiru O, Boutet P, Fernandez-Messina L, Aguera-Gonzalez S, Skepper JN, Vales-Gomez M, et al. Natural killer cell cytotoxicity is suppressed by exposure to the human NKG2D ligand MICA*008 that is shed by tumor cells in exosomes. Cancer Res (2010) 70(2):481–9. doi: 10.1158/0008-5472.CAN-09-1688
25. Dassler-Plenker J, Reiners KS, van den Boorn JG, Hansen HP, Putschli B, Barnert S, et al. RIG-I activation induces the release of extracellular vesicles with antitumor activity. Oncoimmunology (2016) 5(10):e1219827. doi: 10.1080/2162402X.2016.1219827
26. Hollyoake M, Campbell RD, Aguado B. NKp30 (NCR3) is a pseudogene in 12 inbred and wild mouse strains, but an expressed gene in mus caroli. Mol Biol Evol (2005) 22(8):1661–72. doi: 10.1093/molbev/msi162
27. Schuldner M, Dorsam B, Shatnyeva O, Reiners KS, Kubarenko A, Hansen HP, et al. Exosome-dependent immune surveillance at the metastatic niche requires BAG6 and CBP/p300-dependent acetylation of p53. Theranostics (2019) 9(21):6047–62. doi: 10.7150/thno.36378
28. Busse A, Keilholz U. Role of TGF-beta in melanoma. Curr Pharm Biotechnol (2011) 12(12):2165–75. doi: 10.2174/138920111798808437
29. Duchler M, Czernek L, Peczek L, Cypryk W, Sztiller-Sikorska M, Czyz M. Melanoma-derived extracellular vesicles bear the potential for the induction of antigen-specific tolerance. Cells (2019) 8(7):665. doi: 10.3390/cells8070665
30. Plebanek MP, Angeloni NL, Vinokour E, Li J, Henkin A, Martinez-Marin D, et al. Pre-metastatic cancer exosomes induce immune surveillance by patrolling monocytes at the metastatic niche. Nat Commun (2017) 8(1):1319. doi: 10.1038/s41467-017-01433-3
31. Chen G, Huang AC, Zhang W, Zhang G, Wu M, Xu W, et al. Exosomal PD-L1 contributes to immunosuppression and is associated with anti-PD-1 response. Nature (2018) 560(7718):382–6. doi: 10.1038/s41586-018-0392-8
32. Chen J, Song Y, Miao F, Chen G, Zhu Y, Wu N, et al. PDL1-positive exosomes suppress antitumor immunity by inducing tumor-specific CD8(+) T cell exhaustion during metastasis. Cancer Sci (2021) 112(9):3437–54. doi: 10.1111/cas.15033
33. Zhang W, Zhong W, Wang B, Yang J, Yang J, Yu Z, et al. ICAM-1-mediated adhesion is a prerequisite for exosome-induced T cell suppression. Dev Cell (2022) 57(3):329–43.e327. doi: 10.1016/j.devcel.2022.01.002
34. Broggi MAS, Maillat L, Clement CC, Bordry N, Corthesy P, Auger A, et al. Tumor-associated factors are enriched in lymphatic exudate compared to plasma in metastatic melanoma patients. J Exp Med (2019) 216(5):1091–107. doi: 10.1084/jem.20181618
35. Garcia-Silva S, Benito-Martin A, Sanchez-Redondo S, Hernandez-Barranco A, Ximenez-Embun P, Nogues L, et al. Use of extracellular vesicles from lymphatic drainage as surrogate markers of melanoma progression and BRAF (V600E) mutation. J Exp Med (2019) 216(5):1061–70. doi: 10.1084/jem.20181522
36. Leary N, Walser S, He Y, Cousin N, Pereira P, Gallo A, et al. Melanoma-derived extracellular vesicles mediate lymphatic remodelling and impair tumour immunity in draining lymph nodes. J Extracell Vesicles (2022) 11(2):e12197. doi: 10.1002/jev2.12197
37. Shu S, Matsuzaki J, Want MY, Conway A, Benjamin-Davalos S, Allen CL, et al. An immunosuppressive effect of melanoma-derived exosomes on NY-ESO-1 antigen-specific human CD8(+) T cells is dependent on IL-10 and independent of BRAF(V600E) mutation in melanoma cell lines. Immunol Invest (2020) 49(7):744–57. doi: 10.1080/08820139.2020.1803353
38. Huber V, Vallacchi V, Fleming V, Hu X, Cova A, Dugo M, et al. Tumor-derived microRNAs induce myeloid suppressor cells and predict immunotherapy resistance in melanoma. J Clin Invest (2018) 128(12):5505–16. doi: 10.1172/JCI98060
39. Vignard V, Labbe M, Marec N, Andre-Gregoire G, Jouand N, Fonteneau JF, et al. MicroRNAs in tumor exosomes drive immune escape in melanoma. Cancer Immunol Res (2020) 8(2):255–67. doi: 10.1158/2326-6066.CIR-19-0522
40. Wu Y, Deng W, McGinley EC, Klinke DJ 2nd. Melanoma exosomes deliver a complex biological payload that upregulates PTPN11 to suppress T lymphocyte function. Pigment Cell Melanoma Res (2017) 30(2):203–18. doi: 10.1111/pcmr.12564
41. Wolfers J, Lozier A, Raposo G, Regnault A, Thery C, Masurier C, et al. Tumor-derived exosomes are a source of shared tumor rejection antigens for CTL cross-priming. Nat Med (2001) 7(3):297–303. doi: 10.1038/85438
42. Andre F, Schartz NE, Movassagh M, Flament C, Pautier P, Morice P, et al. Malignant effusions and immunogenic tumour-derived exosomes. Lancet (2002) 360(9329):295–305. doi: 10.1016/S0140-6736(02)09552-1
43. Peinado H, Aleckovic M, Lavotshkin S, Matei I, Costa-Silva B, Moreno-Bueno G, et al. Melanoma exosomes educate bone marrow progenitor cells toward a pro-metastatic phenotype through MET. Nat Med (2012) 18(6):883–91. doi: 10.1038/nm.2753
44. Kumar P, Boyne C, Brown S, Qureshi A, Thorpe P, Synowsky SA, et al. Tumour-associated antigenic peptides are present in the HLA class I ligandome of cancer cell line derived extracellular vesicles. Immunology (2022) 166(2):249–64. doi: 10.1111/imm.13471
45. Hood JL. Melanoma exosomes enable tumor tolerance in lymph nodes. Med Hypotheses (2016) 90:11–3. doi: 10.1016/j.mehy.2016.02.018
46. Tewalt EF, Cohen JN, Rouhani SJ, Guidi CJ, Qiao H, Fahl SP, et al. Lymphatic endothelial cells induce tolerance via PD-L1 and lack of costimulation leading to high-level PD-1 expression on CD8 T cells. Blood (2012) 120(24):4772–82. doi: 10.1182/blood-2012-04-427013
47. Cousin N, Cap S, Dihr M, Tacconi C, Detmar M, Dieterich LC. Lymphatic PD-L1 expression restricts tumor-specific CD8+ T cell responses. Cancer Res (2021) 81(15):4133–44. doi: 10.1158/0008-5472.CAN-21-0633
48. du Bois H, Heim TA, Lund AW. Tumor-draining lymph nodes: At the crossroads of metastasis and immunity. Sci Immunol (2021) 6(63):eabg3551. doi: 10.1126/sciimmunol.abg3551
49. Dieterich LC, Tacconi C, Ducoli L, Detmar M. Lymphatic vessels in cancer. Physiol Rev (2022) 102(4):1837–79. doi: 10.1152/physrev.00039.2021
50. Hood JL, San RS, Wickline SA. Exosomes released by melanoma cells prepare sentinel lymph nodes for tumor metastasis. Cancer Res (2011) 71(11):3792–801. doi: 10.1158/0008-5472.CAN-10-4455
51. Pucci F, Garris C, Lai CP, Newton A, Pfirschke C, Engblom C, et al. SCS macrophages suppress melanoma by restricting tumor-derived vesicle-b cell interactions. Science (2016) 352(6282):242–6. doi: 10.1126/science.aaf1328
52. Garcia-Silva S, Benito-Martin A, Nogues L, Hernandez-Barranco A, Mazariegos MS, Santos V, et al. Melanoma-derived small extracellular vesicles induce lymphangiogenesis and metastasis through an NGFR-dependent mechanism. Nat Cancer (2021) 2(12):1387–405. doi: 10.1038/s43018-021-00272-y
53. Maus RLG, Jakub JW, Hieken TJ, Nevala WK, Christensen TA, Sutor SL, et al. Identification of novel, immune-mediating extracellular vesicles in human lymphatic effluent draining primary cutaneous melanoma. Oncoimmunology (2019) 8(12):e1667742. doi: 10.1080/2162402X.2019.1667742
54. Takeda A, Hollmen M, Dermadi D, Pan J, Brulois KF, Kaukonen R, et al. Single-cell survey of human lymphatics unveils marked endothelial cell heterogeneity and mechanisms of homing for neutrophils. Immunity (2019) 51 (3) 561–72.e565. doi: 10.1016/j.immuni.2019.06.027
Keywords: exosome, immune checkpoint, immunotherapy, melanoma, lymph node, metastasis, tumor immunity, extracellular vesicle (EV)
Citation: Dieterich LC (2022) Mechanisms of extracellular vesicle-mediated immune evasion in melanoma. Front. Immunol. 13:1002551. doi: 10.3389/fimmu.2022.1002551
Received: 25 July 2022; Accepted: 09 August 2022;
Published: 23 August 2022.
Edited by:
Susana García-Silva, Spanish National Cancer Research Center (CNIO), SpainReviewed by:
Theresa L. Whiteside, University of Pittsburgh, United StatesCopyright © 2022 Dieterich. This is an open-access article distributed under the terms of the Creative Commons Attribution License (CC BY). The use, distribution or reproduction in other forums is permitted, provided the original author(s) and the copyright owner(s) are credited and that the original publication in this journal is cited, in accordance with accepted academic practice. No use, distribution or reproduction is permitted which does not comply with these terms.
*Correspondence: Lothar C. Dieterich, Lothar.dieterich@pharma.ethz.ch