Tree Species of Wet Tropical Forests Differ in Their Tissue Biochemistry and Effects on Soil Carbon Dynamics
- 1Department of Natural Resource Ecology and Management, Iowa State University, Ames, IA, United States
- 2IIHR-Hydroscience & Engineering, Department of Civil and Environmental Engineering, University of Iowa, Iowa City, IA, United States
- 3USDA-ARS, National Laboratory for Agriculture and the Environment, Ames, IA, United States
Given the hypothesized effects on soil organic matter (SOM) of polyphenols in plant tissues, differences among tree species in their biochemical composition could influence the turnover and accrual of SOM in various ways. The extent to which the biochemical composition of leaf and fine-root tissues differ among tropical tree species, and the effects on soil dynamics, are largely undocumented, however. We used cupric oxide analyses of plant tissues and soil in long-term, replicated, mono-dominant 15-year-old plantations at La Selva Biological Station, Costa Rica, to test for differences among six tree species. We related these results to companion studies in this experimental site to evaluate relationships between interspecific differences in tissue biochemistry and SOM dynamics. Newly senesced leaves and fine roots of the six species differed in their concentrations of three lignin-derived families of phenols, the cinnamyls, syringyls and vanillyls (p < 0.0001 for all tests). Cinnamyl and syringyl phenols in soil differed significantly among species (p = 0.0408, 0.0071, respectively), whereas vanillyl phenols did not (p = 0.83). The degree of decomposition of syringyl and vanillyl phenols in soil also differed (p = 0.0015, 0.0027, respectively), as evidenced by the ratio of carboxylic acid to aldehyde compounds, based on the concept that carboxylic acids are a common by-product of oxidative decomposition of lignin by microorganisms. In our study in a single site, i.e., the same soil type, climate, and growth form of vegetation, total phenols in soil ranged from 5 to 21 mg g–1 organic carbon (OC) across the 20 plots, and the endpoints were both broad-leaved evergreen species; even the means across species, 7–12 mg g–1 OC, covered half the range of values reported in another study across a broad latitudinal range of sites. This study’s tree species differed in traits that influenced at least four factors that explained their differential effects on soil organic carbon (SOC) pools: (1) Fine-root detrital inputs; (2) Fine-root syringyl concentrations; (3) Soil pH; and (4) Macroaggregate structure. This trait-based approach provides a process-based understanding of how trees species influence SOC dynamics, and the consequences for ecosystem properties, under land-use change that involves shifts in species composition.
Introduction
Humid tropical forests account for ∼19.6 million km2 of the Earth’s area (Asner et al., 2009) and contain some of the Earth’s largest soil organic carbon (SOC) stocks (Field et al., 1998; Jobbágy and Jackson, 2000). Development of these SOC stocks has been, and continues to be a function of climate, organisms, parent material, topography, time, and human factors (Jenny, 1941). Of all these factors, we focus on organisms, specifically tree species, because trees are the dominant growth form in tropical forests, which have been undergoing unprecedented rates of land-use change over the last few decades. These changes include fragmentation, degradation, regeneration of secondary forests following deforestation, and logging of remaining forests (Lewis et al., 2015). These changes generally involve shifts in tree species composition, yet it is not clear how these shifts influence SOC stocks.
Tree species can differ in a variety of traits that could influence the quantity and turnover of organic matter (OM) inputs to soil, that would in turn influence SOC stocks differentially as a result of shifts in species composition. These traits include the rate and chemistry of detrital inputs from litterfall and roots, the decomposition of these plant tissues in soil, and the fueling of microbial and fungal populations that influence SOC dynamics and stocks.
Evaluating plant traits that influence tissue decomposition, and tracing the flow of plant-based carbon (C) into soil and its stabilization there, has been a topic of much debate because so many factors can potentially contribute to the persistence of OM inputs to soil. In their review of multiple studies Schmidt et al. (2011) concluded that C residence time was only secondarily influenced by the molecular structure of detrital inputs; rather, the persistence of OM in soil was the result of complex interactions between the biota (including microbes and fungi) and abiotic factors such as climate, mineralogy, soil acidity, and water availability. In a litter-manipulation experiment, Ma et al. (2019) found that differences in temperature sensitivity of the active SOC pool were related to lignin content and the microbial community, whereas for the slow pool temperature sensitivity was related to environmental factors such as pH and soil C/N ratio.
To evaluate the relative impacts of the quantity and biochemistry of plant detrital inputs on SOC dynamics and stocks, we conducted studies in a field experiment in lowland Costa Rica that contains monocultures of six tropical tree species in four blocks, with species selected such that they differed in their root and litter attributes (Fisher, 1995). Established in 1988, this is one of the oldest replicated experiments containing broad-leaved evergreen tree species in the humid tropics. Because all tree species were planted in plots on the same soil type under similar environmental conditions and land-use history, this study focuses on the effects of the tree species on accrual of SOC after 15 years. Previous studies in this site allow for a rich database of traits to evaluate in relation to SOC stocks, including fine-root growth, litterfall, microbial studies, and various soil studies. For the study reported herein, we measured lignin-derived phenols (i.e., biomarkers that could be measured in plant tissues and soil), and evaluated SOC pools and their turnover times using a two-pool model. Given that lignin is a major constituent of forest litter, biomarkers such as cinnamyl, syringyl, and vanillyl phenols have been used to identify broad categories of vegetation in forest litter, e.g., angiosperms vs gymnosperms (Kögel, 1986), or pasture vs forest (Heim et al., 2010). The oxidative degradation of lignin with cupric oxide (CuO) has also been used to characterize the pattern of lignin decomposition (Kögel, 1986; Feng and Simpson, 2011). The acid-to-aldehyde (Ad/Al) ratio of syringyl and vanillyl units has been found to increase with the degree of lignin degradation (Kögel, 1986; Hedges et al., 1988; Opsahl and Benner, 1995; Otto and Simpson, 2006).
To compare and integrate findings about plant tissue biochemistry with SOC dynamics, long-term laboratory incubation-based methods can be used to estimate active and slow turnover pools of SOC and their residence times (Paul et al., 2001). This method has been used to compare SOC dynamics and C sequestration under different soil types and land uses and management (e.g., Six et al., 2002; McLauchlan et al., 2006), although to our knowledge laboratory incubations have not yet been coupled with biochemical analyses of tropical forest SOC.
We addressed four broad hypotheses in relation to differences in tropical tree species traits and their influence on SOC dynamics and stocks:
1. Species differ in the biochemistry of their newly senesced leaves and fine roots, and the differences persist in SOC. While differences between angiosperms and gymnosperms or grasses and trees are well-documented (Kögel, 1986; Heim et al., 2010), we are not aware of any other studies comparing broad-leaved evergreen tropical trees.
2. Species differ in the decomposability of their fine roots and soil, as indicated by the Ad/Al of their syringyl and vanillyl concentrations in soil. While this was true for studies comparing different growth forms and forest types (Guggenberger et al., 1995; Heim et al., 2010), it has not been tested among tropical tree species.
3. The size and turnover of active and passive SOC pools differ among tree species. In a previous study in this site, C mineralization rates differed among the species, as determined from 30-day laboratory incubations (Russell et al., 2007). However, we have not previously evaluated sizes of the active, slow and passive SOC pools and their turnover.
4. Differences among species in both the quantity and biochemistry of detrital inputs influence the dynamics and stocks of SOC. Results from previous studies in this site indicate that the tree species differ in the quantity and decay of fine-root inputs to soil (Valverde-Barrantes et al., 2007, 2009; Raich et al., 2009). However, we have not previously addressed the relative effects of the quantity and biochemistry of detrital inputs and their relationship with the sizes and turnover of SOC pools.
Materials and Methods
Study Site and Field Studies
Study Site
This study was conducted in a lowland Tropical Wet Forest, sensu Holdridge life zones (Hartshorn and Hammel, 1994), within experimental plots established in 1988 at La Selva Biological Station, Costa Rica. Mean annual precipitation (MAP) is 3,960 mm, with no month receiving < 100 mm; mean annual temperature (MAT) is 25.8°C (Sanford et al., 1994). The soil has most recently been classified as an Oxisol, a Mixed Haplic Haploperox (Kleber et al., 2007). The site is hilly, with elevations ranging from 51 to 84 m above mean sea level (Raich et al., 2007). Mean soil temperature (5 cm depth) was similar to air temperature (10 cm above forest floor), 24.4 and 24.1°C, respectively, based on hourly measurements over 24-h periods on >100 days from 2004 to 2010 in these plots (Raich and Valverde-Barrantes, 2017). Of these >2,600 soil temperature observations, 50% fell within a range of −1.3 to +2.0°C of air temperature.
The field experiment was established on a 12-ha site that had been mature wet tropical forest until deforestation in 1955. The site was then converted to cattle pasture dominated by the perennial grasses Panicum maximum L. and Melinis minutiflora Pal. and grazed until abandonment in 1987. In 1988 a randomized complete block experiment was established. The design contained twelve 0.25-ha (50 m × 50 m) plots in each of four blocks; each plot was divided into four quadrants to facilitate stratified random sampling within plots. Each of the 11 plots was planted with a single tree species, with a tree spacing of 3 × 3 m; the 12th plot was left unplanted as a control (Fisher, 1995). For details about site management, and early tree growth and soil properties, see González and Fisher (1994); Fisher (1995); and Haggar et al. (1997). To provide a reference for soil property measurements, we established four 50 m × 50 m plots in the adjacent mature forest, and four 14 m × 8 m plots in the adjacent abandoned pasture.
By 2003, only six of the 11 tree species planted had survived with complete canopy coverage, the six species in this study. We refer to these species by two or four-letter codes (in parentheses), based on the first letters of their genus and species: Hieronyma alchorneoides Allemao (Hial, Ha); Pentaclethra macroloba (Willd.) Ktze, (Pema, Pm); Pinus patula ssp. tecunumanii (Eguiluz & J.P. Perry) Styles (Pipa, Pp); Virola koschnyi Warb., (Viko, Vk); Vochysia ferruginea Mart. (Vofe, Vf); and Vochysia guatemalensis Donn. Sm (Vogu, Vg). All species are native to this region, except Pinus; Pentaclethra is the only nodulated legume. All of the broad-leaved species had arbuscular mycorrhizal associations (Kivlin and Hawkes, 2016b). When this study occurred, the trees had the following averages: stem densities, 200–500 stems ha–1; basal area, 15–40 m2 ha–1; tree diameters 22–37 cm; and heights, 19–33 m (Raich et al., 2007; Valverde-Barrantes et al., 2007).
Plant and Soil Sampling
Newly senesced, fallen leaves were collected from the ground in March 2004. The status of “newly senesced” was determined based on leaf color and integrity, as described by Raich et al. (2007). At least 20 leaves (fascicles in Pinus) were collected for each species in each plot and combined within a plot to yield n = 1 sample per plot. Fine roots (0–2 mm diameter) were sampled in June-July 2004 by carefully excavating and tracing roots to six individual, randomly selected trees within each plot. The six samples per plot were bulked to produce a single sample in each of the 24 plots. Root samples were washed before drying. All plant tissue samples were dried at 65°C, ground in a Wiley mill, and then pulverized to a fine powder using a LABCONCO Spex 8000 Mixer/Mill.
Fine root growth over this same period had been measured in a previously reported study (Valverde-Barrantes et al., 2007, 2009). To summarize, fine-root growth (0–15 cm depth) was measured using the ingrowth core method, using 20 cores per tree species. Fine-root growth was assumed to equal fine-root production because fine-root biomass did not vary significantly 2-year timeframe of that study.
Soil was sampled in the 0–15 cm layer, following the protocol of Russell et al. (2007) in June-July 2003. Within each quadrant of each plot, 20 punch-tube samples, 3.2-cm in diameter, were collected at randomly selected points and then bulked into a single sub-sample, for a total of four sub-samples per plot. For this study, the four sub-samples per plot were combined into a single sample per plot, for a total of 24 soil samples. These soil samples had been air-dried and roots were removed as the soil was passed through a 2-mm sieve. A well-mixed sub-sample was finely ground with mortar and pestle for subsequent analyses. Soil sub-samples were dried at 105°C to determine the air-dry to dry-weight conversion factor.
By 2008, it had become apparent that V. ferruginea was succumbing to a fungal disease. All of the tissue concentrations analyses we report here are for live tissues, and thus would not have been affected by the disease. However, we could not rule out the possibility that this disease could have caused premature fine-root die-off earlier than 2008, and could have thus created an artifact in the fine-root growth and soil data. Thus, we did not include V. ferruginea in any analyses involving soil or fine-root growth. This study was not designed to compare differences in land use, but for reference purposes, we used the same protocols to sample soil in the adjacent mature forest and abandoned pasture.
Laboratory Studies
Lignin-derived Phenols
Cupric Oxide extractable, lignin-derived phenols were measured by adapting the procedure of Goñi and Hedges (1992). Samples of 100 mg soil or 6 mg roots were oxidized with alkaline CuO and 2 M NaOH for 3 h in a stainless steel minibomb. Bombs were loaded into a specially designed carousel in a refitted Hewlett-Packard 5890A gas chromatograph (GC). The heating rate was 4.2°C/min for 30 min from a start temp of 27°C, and a final temp of 150°C was held for 150 min. Following heating, ethyl vanillin in a concentration of 240 ng μl–1 (25 μl for soils, 100 μl for roots) was added to each sample as the internal standard. The minibombs were closed again, shaken by hand, then centrifuged for 5 min at 3,500 rpm, and their supernatant transferred to test tubes. Three ml of 6 M HCl was added to each bomb to neutralize the NaOH and each test tube was vortexed. About 2.5 g NaCl was added to each test tube, then capped and mixed by hand. About 3 ml ether was added, then again capped and mixed by hand. Test tubes were shaken for 2 min to accumulate the phenols in the ether, then centrifuged for 2 min at 2,000 rpm. The ether layer containing the phenols was then carefully pipetted from the surface of each solution into a new test tube. Na2SO4 (∼2.5 g) was added to each tube to remove residual H2O from the ether solution. Tubes were shaken, and if necessary stored under refrigeration for overnight.
For derivitization and chromatography, samples were first filtered through quartz wool then concentrated by rotary evaporation to about 1 ml volume, then transferred to 4-ml vials, and dried to nearly air-dryness under a stream of Ar gas. The precipitate was then dissolved in 150 μl pyridine/50 μl methyl-3,4-dimethoxybenzoate (soils) or 300 μl pyridine/100 μl methyl-3,4-dimethoxybenzoate (roots). The methyl-3,4-dimethoxybenzoate served as a recovery standard a 50 μl aliquot of each solution was added to a new 4-ml vial and mixed with 50 μl of the derivitizing agent bis(trimethylsilyl)trifluoroacetamide and then placed in a heating block at 70°C for 30 min, after which the samples were loaded into the GC. Gas chromatography was carried out with a Hewlett-Packard 6890 GC equipped with a derivatized, non-packed injection liner, a HP-5 (5% crosslinked phenylmethyl siloxane) capillary column (30-m length, 0.32-mm column id., 0.25-μm film thickness) and a flame ionization detector. The following conditions were employed for phenol separation: injector temperature, 300°C; temperature ramp, 100°C ramped to 220°C at 4°C/min, 220°C ramped to 270°C at 25°C/min and held for 10.1 min; detector temperature 300°C. Results are reported in the conventional units of SOC enrichment, that is mg phenol g OC–1.
Soil C Pool Sizes and Turnover Rates
To determine sizes of active and slow pools and their respective turnover rates, we used long-term incubations of soil at 23°C, similar to field ambient temperature, coupled with a chemical approach, described by Paul et al. (2001). We incubated 16 field replicates of soil for each species, using sieved (2 mm), air-dried subsamples of 3 g, from each of the 4 quadrants × 4 blocks, as described in Russell et al. (2007). To summarize, the subsample was mixed with acid-washed sand (1:1 w/w) and adjusted to 60% water-filled pore space, using deionized water that contained 2 mL of inoculant (5 g field-moist soil, combined from all plots, homogenized with 50 mL distilled water). We measured CO2-C release rates periodically over 400 day (before CO2 concentrations reached 4%); the flask headspace was flushed through an infrared gas analyzer (LI-820, LI-COR Biosciences, Inc., Lincoln, NE, United States). The air supply was piped through a CO2-free water bath to keep the incubation-tube atmosphere hydrated. The mean lab temperature was very close to ambient field temperature, so we did not perform a temperature conversion of the data.
We determined the resistant fraction by refluxing 1-g soil subsamples in 6 M HCl at 115°C for 16 h on a controlled-temperature digestion block as described by Paul et al. (2001). The refluxed samples were then washed three times with deionized water, dried at 55°C and finely ground in a mortar and pestle. Resistant soil C was measured by dry combustion, using a Thermo-Finnigan EA Flash (Series 1112, EA Elantech, Lakewood, NJ, United States).
Data Calculations and Analyses
Soil Pool Parameter Calculations
We used curve analyses of CO2 evolved for each sample incubated over 400 days (n = 96) to determine the size and turnover rates of the active (Ca) and slow (Cs) SOC pools as in Paul et al. (2001). We did not have carbon dating for these soils, so we used a two-pool constrained model in the regression analysis:
where C(t) is the total C pool at time t, Ca and ka are the size and decay rate of the active pool. Cs is the size of the slow pool (SOC – Cr – Ca), where Cr is the size of the resistant pool as determined by acid hydrolysis and ks is the turnover of the slow pool. The mean residence times (MRTa and MRTs) were calculated as 1/k for each of the two soil pools. We used non-linear regression to calculate the parameters Ca, ka, and ks, using PROC NLIN in the Statistical Analysis System (SAS Institute Inc [SASI], 1999). A Marquardt fit was used with initial parameter settings of: ka = 0 to 0.3 by 0.05; ks = 0.001 to 0.005 by 0.001; and Ca = 100 to 2,000 by 100.
Analysis of Variance
The plot was the experimental unit; for variables with measurements at the quadrant level, the mean value for the plot was used in analyses. Soil data from V. ferruginea and the reference plots (mature forest and abandoned pasture) were not included in the analyses. To evaluate whether the tree species had effects on more than one response variable among soil phenols and SOC (pools and total), we first conducted a multivariate analysis of variance. Interactions among the response variables were significant, so we then tested response variables individually, using a generalized linear mixed effects model with both fixed and random effects in SAS (Littell et al., 1996). We tested for homogeneity of variances and normality of distributions. Variables that did not meet these assumptions included root and soil cinnamyl, and soil syringyl and vanillyl phenols, which met the assumptions when natural log transformed. Thus, analyses were conducted on the transformed data; we present the un-transformed values, however. Differences were considered to be significant at α ≤ 0.05. Pairwise comparisons for significant overall F-tests were performed using p-values adjusted by Tukey’s Honestly Significant Difference method.
Multiple Regression Models
We used a multiple regression model to evaluate the relative effects of the quantity, biochemistry and turnover of OM on one response variable, total SOC stocks. We used partial residual analysis to quantify the effect of a single explanatory variable on the response variable, total SOC stocks, after the effects of other possible explanatory variables in the model had been accounted for (Neter et al., 1996). With only n = 20 plots for this analysis, we were limited to only six explanatory variables in the model. There is a rich data set for this field experiment, so to select the six explanatory variables from the set of many possibilities, we used Pearson correlation analyses to evaluate the strength and significance of pair-wise comparisons of variables (Supplementary Table 1). Variables from other microbial and fungal studies in these plots were not included in the set of possible variables because previous studies had indicated a lack of difference among the tree species in microbial and fungal parameters (Kivlin and Hawkes, 2016a, b); also, microbial and fungal variables were not correlated with total SOC stocks (Russell et al., 2018). For plant tissue biochemistry, we evaluated fine roots but not leaves, given that fine roots had been more correlated with SOC stocks than were leaves in another study at La Selva (Russell et al., 2004). Based on the results of the correlational analyses, the six explanatory (=predictor) variables were included in a multiple regression model They were: (a) fine-root syringyls (mg 100 mg OC–1); (b) fine-root growth (Mg C ha–1 year–1); (c) acid/aldehyde of soil syringyls (mg 100 mg OC–1); (d) MRTs (year); (e) active SOC pool (mg kg–1); and (f) soil syringyls (mg 100 mg OC–1); all variables relate to the 0–15 cm soil layer.
Results
Phenols in Plant Tissues and Soils
Across the tree species in this study, mean total cinnamyl phenol concentrations (mg g OC–1) ranged from 1.5–3.4 in leaves, 0.8–3.7 in fine roots, and 0.9–1.7 in soil (Table 1). Mean total syringyl phenols (mg g OC–1) ranged from 0.3–6.7 in leaves, 1.8–13.4 in fine-roots, and 1.5–5.6 in soil. Mean total vanillyl phenols (mg g OC–1) ranged from 3.8–16.0 in leaves, 8.3–17.5 in fine roots, and 3.6–5.5 in soil. Thus, for cinnamyl and separately for vanillyl phenols, concentrations in leaves and fine roots were of similar magnitudes. In contrast, the average concentration across all plots of syringyl phenols in fine roots was double that of leaves, 7.8 and 3.8 mg g OC–1, respectively. Leaf and fine-root syringyl concentrations were significantly correlated (r = 0.62, p = 0.0098), as were vanillyl phenols (r = 0.79, p = 0.0003), but cinnamyl phenol concentrations were not significantly correlated (r = 0.18, p = 0.4936; Supplementary Table 1). The ratio of the phenolic compounds in fine roots-to-soil was calculated for each species. Across species, cinnamyl root concentrations ranged from 0.9 to 1.7 times that of soil, whereas the ratio was higher for syringyl phenols, 1.2 to 3.3 across species and for vanillyl phenols, 2.2 to 3.3.
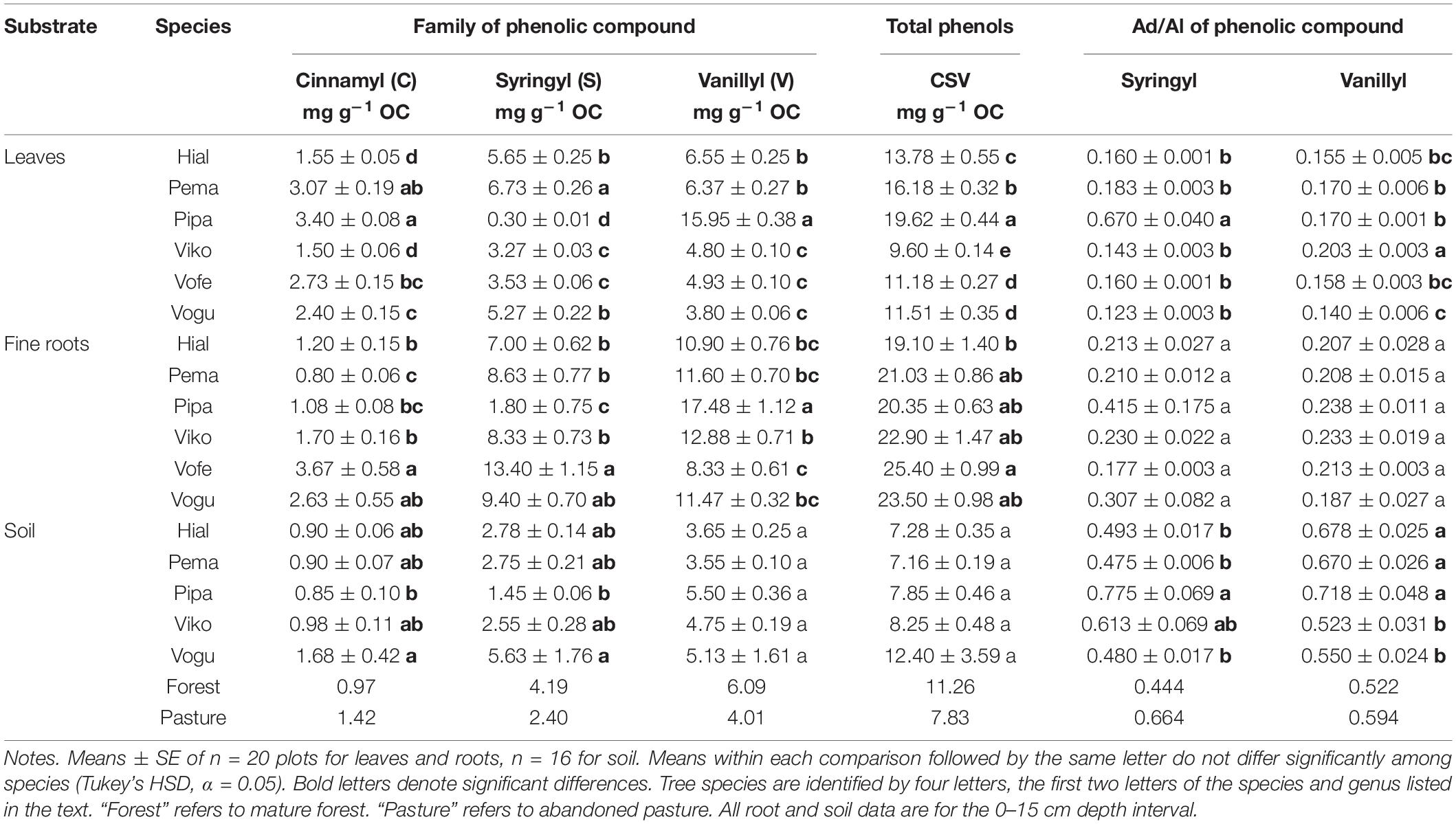
Table 1. Phenolic compounds and their Acid/aldehyde (Ad/Al) ratios in six tropical tree species in 15-year-old plantations.
Regarding comparisons among species, all three phenolic classes differed significantly among the tree species for both leaf and fine-root tissues (Tables 1, 2). For soil, species did not differ significantly in vanillyl phenol concentrations; for cinnamyl and syringyl phenols, the differences among species were significant, but not as strongly as for plant tissues. The sum of cinnamyl, syringyl, and vanillyl phenols (CSV) ranged from 5 to 21 mg g–1 OC across the 20 plots (published dataset), with a mean of 7–12 mg g–1 OC across species (Table 1). In comparison, the CSV sums for soil in the mature forest and abandoned pasture reference samples were 11 and 8 mg g–1 OC, respectively, with Vochysia most closely resembling mature forest values.
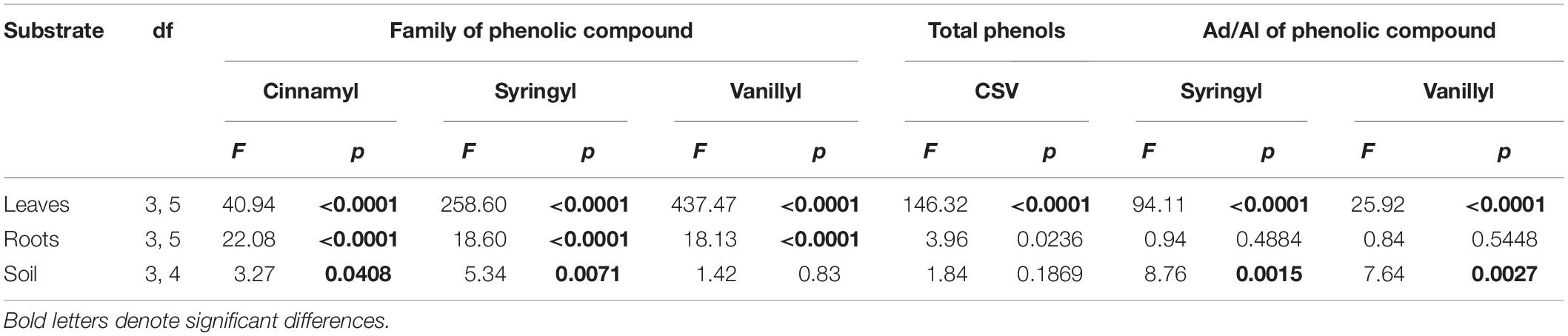
Table 2. Summary table of ANOVA results for families of phenolic compounds and their Acid/Aldehyde (Ad/Al) ratios in six tropical tree species.
The Ad/Al of both syringyl and vanillyl phenols in soil differed significantly among species (Tables 1, 2), with that of Pinus higher than Hieronyma, Pentaclethra and Vochysia for syringyl phenols and Hieronyma, Pentacletha and Pinus greater than that of Virola and Vochysia for vanillyl phenols. In the mature forest, the Ad/Al for syringyl phenols in soil was lower than that of the abandoned pasture, with Pinus and Virola more closely resembling that of the abandoned pasture, and the other three species closer to the mature forest values. There was not a significant relationship of the Ad/Al of syringyl phenols in soil with either root or soil syringyl concentrations (Figure 1 and Supplementary Table 1). Acid/aldehyde ratios of roots and soil were not significantly correlated, for either syringyl or vanillyl phenols; nor was the soil Ad/Al correlated with total SOC for either biochemical family (Figures 1, 2 and Supplementary Table 1).
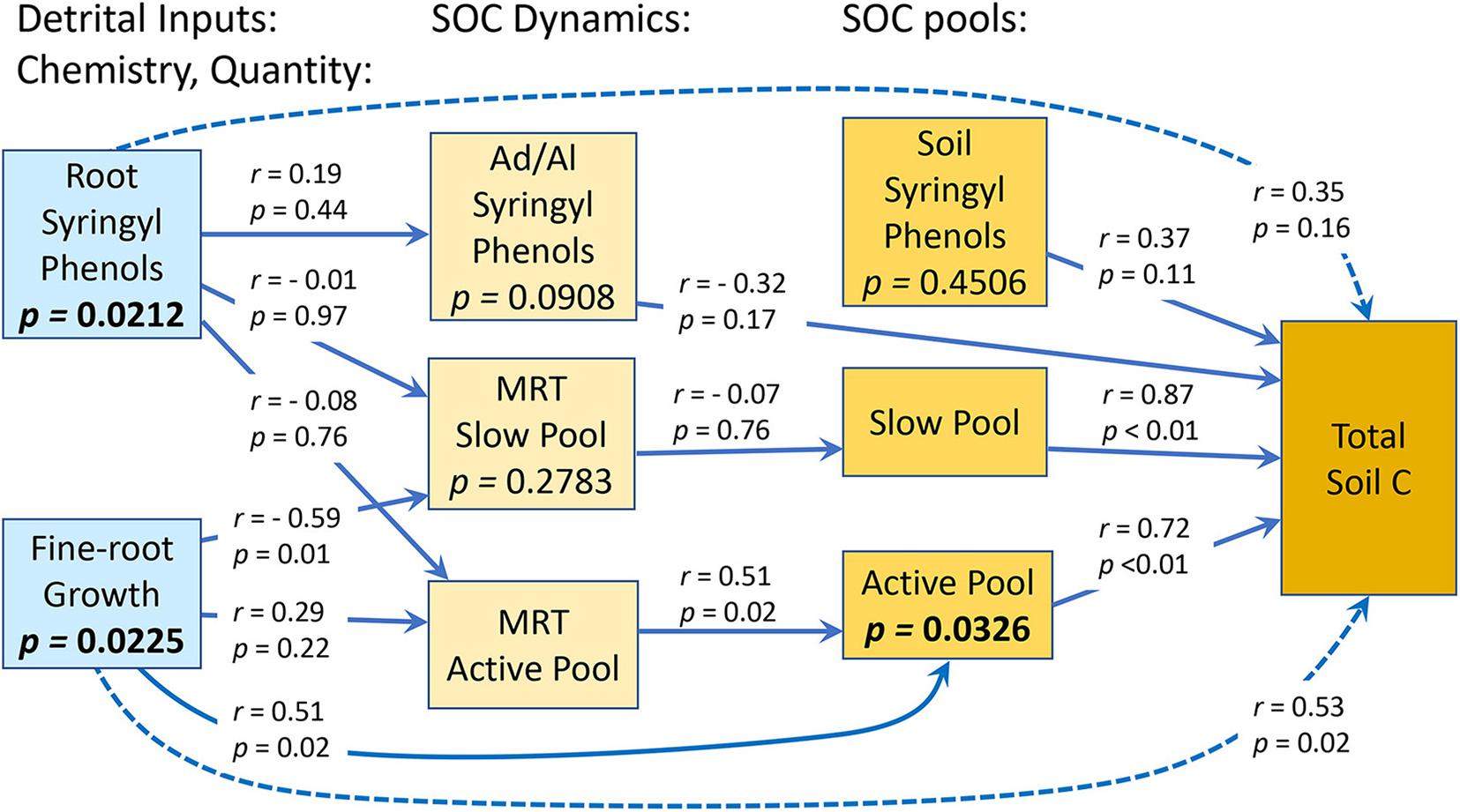
Figure 1. Relationships between quantity and chemistry of detrital inputs to soil, and SOC dynamics and pools in an experiment containing five tropical tree species. Results of correlations are shown on arrows connecting the pair of variables in the analysis. P-values in boxes are from partial residual analyses. N = 20 plots.
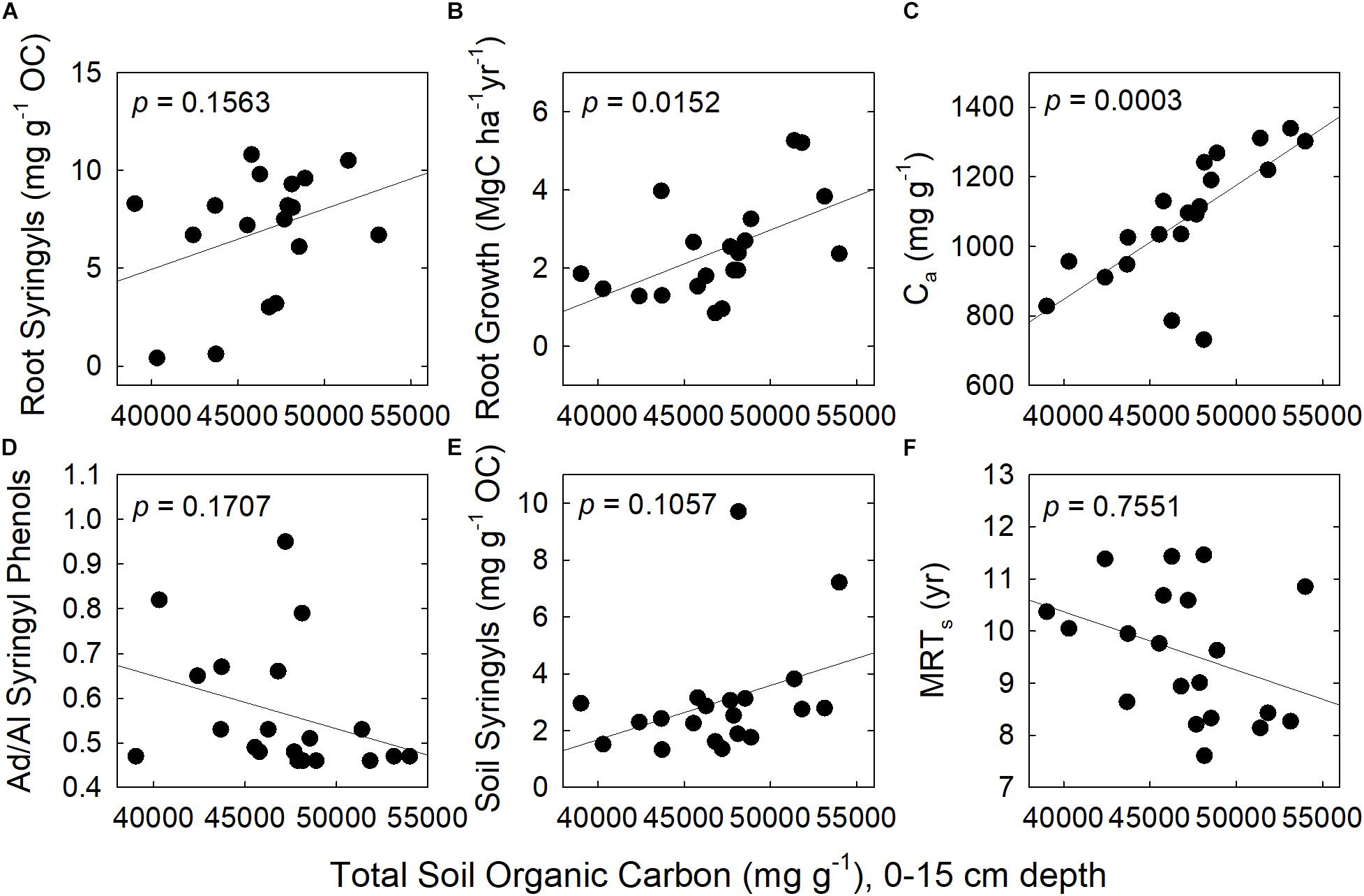
Figure 2. Correlations between total SOC and six explanatory variables. They are: (A) Root syringyl phenols; (B) Fine-root growth; (C) Size of the active soil pool, Ca; (D) Acid/Aldehyde of Syringyl phenols in soil; (E) Soil syringyl phenols; and (F) Mean Residence Time of the slow pool, MRTs. N = 20 plots.
SOC Pools and Turnover and Fine-Root Growth
The mean size of the Ca (active pool) ranged from 880 to 1,281 mg kg–1 across species; the Cs (slow pool) was 25 to 32 times larger, ranging from 27,000 to 32,000 mg kg–1 (Table 3). Both the active and slow pools were significantly larger in Vochysia and Hieronyma. The Ca pool was smallest in Virola and the Cs pool smallest in Pinus and Pentaclethra. The tree species differed significantly in the turnover of the slow pool, reported here as the mean residence time (MRTs): it was longest in Virola and shortest in Hieronyma (Table 3). In contrast, the MRTa was similar across species. Fine-root growth differed significantly among the species, ranging from 1.2 to 3.9 Mg C ha–1 year–1 in Pinus and Hieronyma, respectively (Table 3; Valverde-Barrantes et al., 2007, 2009).
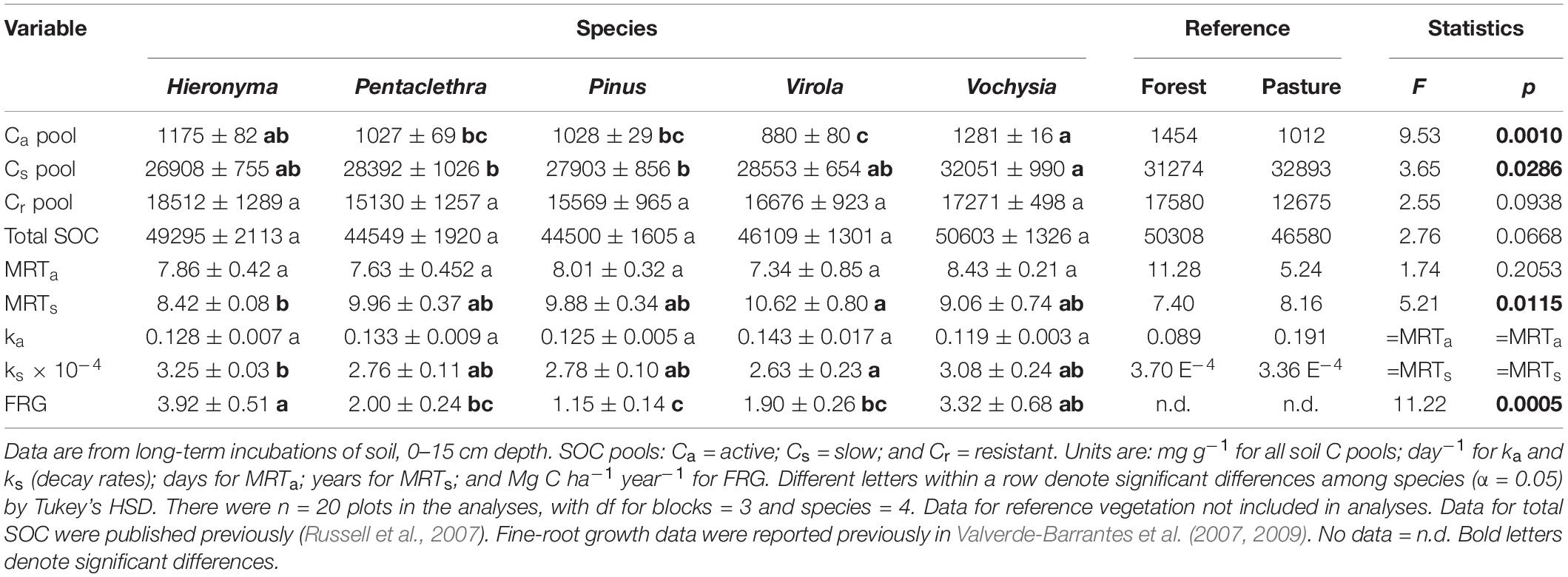
Table 3. Soil organic carbon (SOC) pools and their turnover and fine-root growth (FRG) in five tropical tree species.
Correlation Analysis Results
Across species, fine-root growth was significantly correlated with the Ca, MRTs, and total SOC (Figures 1, 2 and Supplementary Table 1). In contrast, root syringyl phenols were not significantly correlated with these measures of SOC turnover, or with the Ad/Al of soil syringyl phenols or total SOC stocks. Within the total SOC, the relationship between the pool size and its turnover differed with the pool: Ca and MRTa were significantly correlated, but Cs and MRTs were not (Supplementary Table 1).
Partial Regression Results
Based on the results of the correlation analyses, six explanatory variables were included in the multiple regression model to analyze their effects on total SOC stocks: (a) Fine-root growth (FRG); (b) Fine-root syringyls (RtSyr); (c) Acid/Aldehyde ratio of Soil Syringyls (AdAlSyr); (d) mean residence time of the slow pool (MRTs); (e) size of the active SOC pool (Ca); and (f) Soil Syringyls (SoSyr). Of those six variables, fine-root growth, fine-root syringyls and the size of the active SOC pool were significant after the other variables have been taken into account (Table 4). Comparisons of various multiple regression models indicated that for the simplest models (only one variable), the size of the active pool and fine-root growth explained the most variability (Table 5). However, in 2-variable models, the addition of root syringyls explained substantially more of the variability, without over-fitting the model. The addition of Ad/Al soil syringyls in a 3-variable model had a similar effect. Beyond that, addition of the other variables had diminishing explanatory effects.
Discussion
Effects of Tropical Tree Species on Phenolic Biochemistry
Lignin signatures based on CuO analysis have been used to distinguish between vegetation types (Hetherington and Anderson, 1998), effects of land-use change (Guggenberger et al., 1994), and invasions of a new growth form, as in shrub encroachment in grasslands in Mongolia (Zhou et al., 2018). Most studies of plant phenolic contents in relation to SOC dynamics take place in the temperate zone or higher latitudes. Moreover, studies of plant tissues usually concern aboveground plant components, despite that root-derived C dominates SOC stocks in comparison with aboveground detrital inputs, as reviewed in Schmidt et al. (2011).
Comparison of a cropping rotation, grassland, mixed deciduous forest and spruce forest in a pre-Alpine region, found that the sum of lignin-derived soil phenols, CSV, ranged from 11 to 23 g/kg (Guggenberger et al., 1994). Across 18 sites that encompassed a broad range of latitudes and soil types from Saskatchewan (Canada) to Texas (United States), in which MAT ranged from 0.9 to 23.4°C and MAP from 300 to 1,308 mm, CSV phenols in soil ranged from 9 to 26 mg g–1 OC (Amelung et al., 1999). In our study in a single site, i.e., the same soil type, climate, and growth form of vegetation, CSV phenols in soil ranged from 5 to 21 mg g–1 OC across the 20 plots; even the means across species, 7–12 mg g–1 OC (Table 1), covered half the range found in the broad latitudinal study by Amelung et al. (1999). The broad range found in our study was not driven by different growth forms either: values for the only gymnosperm species in the experiment fell in the middle of the range, with the endpoints of the range both represented by broad-leaved evergreen tropical tree species.
In a study of 18 sites across a range of biomes, from taiga and tundra to temperate forest and tropical forest, Vancampenhout et al. (2009) concluded that tropical soils were low in lignin. However, two of three forests in that study were dry forests. Low soil lignin content could be explained by lower detrital inputs in the drier forests, given the results of another study in which net primary productivity (NPP) in tropical forests declined with MAP for sites with MAP less than ∼2,500 mm (Schuur, 2003). It is also possible that lignin degraded faster in the drier forests, given the finding that lignin was subject to accelerated degradation via photodegradation in a semi-arid environment because lignin effectively absorbed light over a wide range of wavelengths (Austin and Ballaré, 2010). Thus, the lower lignin contents in tropical dry forests could be the result of an abiotic factor that increases lignin degradation.
SOC Pools and Their Turnover
In our study, the Ad/Al ratio of both syringyl and vanillyl phenols in soil differed significantly among species. For syringyl phenols, this ratio decreased significantly as fine-root growth increased (r = −0.51, p = 0.0210). This suggests that in a species such as Vochysia, which had a low Ad/Al ratio, the soil pool of syringyl phenols was being replenished relatively more with inputs of fine-root detritus. Hieronyma, however, also had relatively high fine-root growth, but a relatively higher Ad/Al ratio, suggesting that its fine-root detritus decomposed relatively faster. Thus, while the Ad/Al ratio provides a measure of the state of decomposition of OM within the soil, when comparing different treatments (e.g., land use, species, and history), the interpretation of these data requires information about the quantity of detrital inputs to the soil pool in each of the treatments.
The size of the active pool (0-15 cm), averaged across all species in our site, 1,078 mg kg–1 (Table 3) was nearly double that reported for an agricultural soil (0-20 cm) at Kellogg Biological Station in Michigan, 570 mg kg–1 (Paul et al., 2001). The slow, resistant and total SOC pools in our site were > 5-, 3-, and 4-fold greater, respectively, than those reported for the Michigan site. The MRT in that site for the active and slow SOC pools were 20 days and 12 years under laboratory temperatures, respectively, compared with 8 days and 10 years for MRTa and MRTs, respectively, averaged across species in this study (Table 3). When the Michigan data were converted to field temperatures, however, the MRTa was 60 days and MRTs was 37 years. For the tropical soils in this study, the laboratory and field incubation temperatures were nearly the same; thus these tropical soils were more dynamic and had larger SOC pools.
Tree Species Effects on Accrual and Persistence of SOC
Given that lignin is generally considered to be recalcitrant, owing to its complex chemical structure that protects litter from microbial attack (Swift et al., 1979), variation in lignin content among species is expected to translate into species-specific variation in the decomposability of their tissues. Indeed, in multiple studies of decomposition of aboveground plant tissues, higher lignin content has been highly correlated with slower decomposition of detritus (Melillo et al., 1982; Berg et al., 2000; Cornwell et al., 2008). Lignins were also expected to degrade slowly in soil, but the evidence regarding lignin’s recalcitrance within soil has been varied. A review by Vancampenhout et al. (2009) indicated a lack of persistence of lignins in soil. In a comparison of lignin biomarkers in a pasture and rainforest soil in subtropical Australia, Heim et al. (2010) found that only small portions of rainforest-derived lignin persisted 100 years after conversion to pasture, indicating that lignin biomarkers didn’t provide a proxy for stable SOC. In tropical soils, higher temperature is thought to increase decomposition of lignin, and thus explain their low lignin contents. As indicated above, however, the soils in our study site had lignin contents on par with temperate-zone sites. Mineral-organic associations can stabilize a large fraction of SOC (Mikutta et al., 2009), however, and this could provide a mechanism for stabilizing lignin-derived phenols in our study site’s Oxisol. In addition, given the high rates of NPP (Russell et al., 2010), detrital inputs were replenishing soil lignin stocks in this site. This study was not designed to distinguish between these two mechanisms of lignin retention and accrual in soil, however.
Many studies have demonstrated that leguminous trees increase SOC stocks, as reviewed by Mayer et al. (2020). Given our results that the only nodulated legume species in the study, Pentaclethra, had low SOC stocks (Table 3), we suggest that the generalization about tree species with N-fixing root associates may well hold for sites that are relatively limited in available N, but not necessarily in tropical sites where N is relatively less limiting for microbial processes (Camenzind et al., 2018). Our data also suggest that lower inputs from roots was an important factor that explained differences among tree species in the accrual of SOC stocks (Figure 1). In biomarker studies in temperate zone forests, roots have also been shown to be more important contributors to SOC than aboveground detritus (Crow et al., 2009; Spielvogel et al., 2014). Root activities in soil may afford them physical protection through mycorrhiza and interactions with metal ion (Rasse et al., 2005). By allocating C to microbes, roots may also prime microbial processes, and thus speed up decomposition of older SOC and thus, availability of nutrients for plant uptake (Fontaine et al., 2007; Kuzyakov, 2010), thereby stimulating plant growth and the detrital inputs to soil.
Tree species identity, i.e., species-specific effects, have been shown to influence SOC stocks across a range of experiments (Vesterdal and Raulund-Rasmussen, 1998; Hansson et al., 2011; Osei et al., 2021). Nevertheless, in their evaluation of a large set of factors that could provide indicators of SOC storage, Wiesmeier et al. (2019) concluded that there was no consensus among previous studies regarding the effects of tree species on SOC storage. Some studies distinguish between broad taxonomic categories of tree species, and then find differences in SOC storage between the categories, e.g., gymnosperms and broad-leaved species, without differences within a category (Mayer et al., 2020). In this study, however, we found differences among the four broadleaved evergreen tropical species in their pools of SOC and turnover times.
Kleber et al. (2011), in their comparisons of three different soil types, found that the turnover rate of SOC was not determined solely by the thermodynamic stability of the detrital inputs, such that chemical “recalcitrance” of SOC was not a good predictor of its turnover. Schmidt et al. (2011) emphasized that although compound chemistry is a driver of decomposition, its relative importance must be considered within the context of other environmental factors. Lehmann and Kleber (2015) found that prediction of SOC turnover required an understanding of the interactions between the substrate, microbes, and abiotic drivers; as such, OM quality was a poor predictor of OM persistence. In our study, there was not a significant correlation between total SOC stocks and the Ad/Al ratio of soil, for either syringyl or vanillyl phenols (Supplementary Table 1). These ratios can represent the state of oxidation of these phenolic compounds, and thus indicate the intensity of microbially driven oxidation or the length of time the phenols have been in soil, rather than intrinsic phenol stability. That interpretation is consistent with the results from these experimental plots that microbial composition did not differ among tree species (Kivlin and Hawkes, 2016a, b).
In contrast with phenol Ad/Al ratios in soil, fine-root syringyl concentrations did explain differences in total SOC stocks across species in our study, after other variables were taken into account in the partial residual analysis (Table 4 and Figures 1, 2). This effect of biochemistry on SOC stocks was likely promoted in part by high NPP, and accentuated by the short-term recalcitrance of syringyl phenols; these two factors together would cause syringyl input rates to exceed their degradation rates. Fine-root growth was significantly correlated with fine-root syringyl phenol content (r = 0.71, p = 0.03, and Supplementary Table 1), indicating the importance of input rates. However, of the three families of root phenols in this study, only the syringyl phenols, the “woodiest” of the three, were also identified as a significant factor; this indicates a role of their specific chemical nature or location within roots, in addition to quantity of inputs alone. In contrast, the more degradable cinnamic phenols had much lower accumulations in soil (Table 1), further suggesting that biochemistry played a role in soil organic matter dynamics.
Given the experimental design of our study, however, abiotic factors, e.g., MAT, MAP, parent material, time of soil development, relief, and previous land-use history were similar across plots. Certainly, individual species can influence microclimate and microbial communities that could influence SOC dynamics. Given that the tree species in this experiment had similar canopy coverage and height, microclimate differences were not expected to differ significantly. Other results from this study site indicated that soil bacteria richness, composition and soil fungal abundance, richness, and composition did not differ among the tree species (Kivlin and Hawkes, 2016a, b). Based on this and other studies in this field experiment, we suggest that at least four other factors explained the differences among the tree species in SOC pools and dynamics. (1) Differences among the species in fine-root productivity (Valverde-Barrantes et al., 2007) and decay rate (Raich et al., 2009) resulted in differential inputs of detritus to the soil pool. (2) Differences in fine-root syringyl phenols explained some of the variation in total SOC pools across species (Tables 1, 4). (3) The species differed in their effects on soil pH, which in turn influenced the availability of cations that are limiting in this Oxisol, and hence the quantity and chemistry of detrital inputs to soil (Russell et al., 2017). As a result of Vochysia’s trait of accumulating aluminum, this species de-acidified the soil. We hypothesized that increasing the soil pH above the point of zero charge in this soil with variable-charge clays dispersed soil organo-mineral colloids, thereby liberating cations available for plant uptake. In contrast, as a result of its N-fixing association, Pentaclethra reduced soil pH below the point of zero charge, which was hypothesized to reduce cation availability for uptake. (4) The species also differed in their effects on macroaggregate structure (Russell et al., 2018), which would have differentially influenced the protection of OM in soil.
Given the rates and extent of land-use change in humid tropical forests over the last several decades (Asner et al., 2009) that have generally resulted in loss of biodiversity, the need is ever more urgent to understand the effects of tree species on soil C. Our results from these studies indicate that, as Schmidt et al. (2011) suggested, the explanation for differences among the tree species in their effects on SOC stocks and dynamics is complicated and involves a mix of factors. Nevertheless, developing a framework for evaluating the effects of species advances our conceptual framework of carbon cycling (Russell et al., 2010), and offers managers an important tool, selection of tree species, for forest regeneration projects or establishment of plantation. Our study illustrates that a trait-based approach can provide a process-based understanding of how trees can influence SOC dynamics at a finer-tuned taxonomic level than the Division, e.g., Angiosperms vs. Gymnosperms, and the consequences for ecosystem properties such as SOC stocks.
Conclusion
We compared tropical tree species in terms of the quantity of detrital inputs and tissue biochemistry and evaluated the effects of these differences on SOC pools and turnover. The study was conducted in a 15-year-old field experiment containing six tree species, including one gymnosperm and one nodulated legume, grown in monocultures in four blocks. Even within the category of broad-leaved evergreen tree species, the species differed in their concentrations of phenols in tissues and soil. In this single site with the same soil type, MAT and MAP, the means of total phenols across the study species encompassed half the range of values found in another study that covered sites across a broad latitudinal range (Canada to Texas). We used partial residual analysis to quantify the effect of a single explanatory variable on SOC stocks; results indicated that differences among species in syringyl phenols in fine roots, and in the quantity of detrital inputs from fine roots explained differences among species in SOC stocks. Previous studies in this site indicated that the tree species also influenced SOC accumulation differentially as a result of species-specific effects on soil pH and macroaggregate structure. Together, these results highlight the complexity of tree species effects on soil, and the need for a trait-based approach in the selection of tree species when ameliorating degraded sites after land-use change.
Data Availability Statement
The original contributions presented in the study are included in the article/Supplementary Material. The datasets for this study are available: doi: 10.5061/dryad.fbg79cntt.
Author Contributions
AR and DO conceptualized the study and acquired funding. RM collected the data under supervision of AR and DO. AR analyzed the data, designed and ran the model, and prepared the figures and tables. All authors contributed to interpretation of the findings. AR and RM wrote the original draft, and all authors participated in reviewing and editing the manuscript.
Funding
Funding was provided by United States National Science Foundation grants DEB-0236502 and a Research Experience for Undergraduates supplement on this grant, DEB-0413682 and IOS-0703561.
Conflict of Interest
The authors declare that the research was conducted in the absence of any commercial or financial relationships that could be construed as a potential conflict of interest.
Acknowledgments
We are grateful to Ricardo Bedoya and the ECOS staff for assistance in the field. We thank Jennifer Boeckmann and Nick Bessman for assistance with the laboratory phenols study and Matt Dornbush for assistance with the long-term soil incubations. We thank Philip Dixon and Haoyan Hu for statistical advice.
Supplementary Material
The Supplementary Material for this article can be found online at: https://www.frontiersin.org/articles/10.3389/ffgc.2021.674213/full#supplementary-material
References
Amelung, W., Flach, K. W., and Zech, W. (1999). Lignin in particle-size fractions of native grassland soils as influenced by climate. Soil Sci. Soc. Am. J. 63, 1222–1228. doi: 10.2136/sssaj1999.6351222x
Asner, G. P., Rudel, T. K., Aide, T. M., Defries, R., and Emerson, R. (2009). A contemporary assessment of change in humid tropical forests. Conserv. Biol. 23, 1386–1395. doi: 10.1111/j.1523-1739.2009.01333.x
Austin, A. T., and Ballaré, C. L. (2010). Dual role of lignin in plant litter decomposition in terrestrial ecosystems. Proc. Natl. Acad. Sci. 107, 4618–4622. doi: 10.1073/pnas.0909396107
Berg, B., Johansson, M. B., and Meentemeyer, V. (2000). Litter decomposition in a transect of Norway spruce forests: substrate quality and climate control. Can. J. For. Res. 30, 1136–1147. doi: 10.1139/x00-044
Camenzind, T., Hättenschwiler, S., Treseder, K. K., Lehmann, A., and Rillig, M. C. (2018). Nutrient limitation of soil microbial processes in tropical forests. Ecol. Monogr. 88, 4–21. doi: 10.1002/ecm.1279
Cornwell, W. K., Cornelissen, J. H., Amatangelo, K., Dorrepaal, E., Eviner, V. T., Godoy, O., et al. (2008). Plant species traits are the predominant control on litter decomposition rates within biomes worldwide. Ecol. Lett. 11, 1065–1071. doi: 10.1111/j.1461-0248.2008.01219.x
Crow, S. E., Lajtha, K., Filley, T. R., Swanston, C. W., Bowden, R. D., and Caldwell, B. A. (2009). Sources of plant-derived carbon and stability of organic matter in soil: implications for global change. Glob. Change Biol. 15, 2003–2019. doi: 10.1111/j.1365-2486.2009.01850.x
Feng, X., and Simpson, M. J. (2011). Molecular-level methods for monitoring soil organic matter responses to global climate change. J. Environ. Monitor. 13, 1246–1254. doi: 10.1039/c0em00752h
Field, C. B., Behrenfeld, M. J., Randerson, J. T., and Falkowski, P. (1998). Primary production of the biosphere: integrating terrestrial and oceanic components. Science 281, 237–240. doi: 10.1126/science.281.5374.237
Fisher, R. F. (1995). Amelioration of degraded rain forest soils by plantations of native trees. Soil Sci. Soc. Am. J. 59, 544–549. doi: 10.2136/sssaj1995.03615995005900020039x
Fontaine, S., Barot, S., Barré, P., Bdioui, N., Mary, B., and Rumpel, C. (2007). Stability of organic carbon in deep soil layers controlled by fresh carbon supply. Nature 450, 277–280. doi: 10.1038/nature06275
Goñi, M. A., and Hedges, J. I. (1992). Lignin dimers: structures, distribution, and potential geochemical applications. Geochim. Cosmochim. Acta 56, 4025–4043. doi: 10.1016/0016-7037(92)90014-A
González, J. E., and Fisher, R. F. (1994). Growth of native forest species planted on abandoned pasture land in Costa Rica. For. Ecol. Manag. 70, 159–167. doi: 10.1016/0378-1127(94)90083-3
Guggenberger, G., Christensen, B. T., and Zech, W. (1994). Land-use effects on the composition of organic matter in particle-size separates of soil: I. Eur. J. Soil Sci. 45, 449–458. doi: 10.1111/j.1365-2389.1994.tb00530.x
Guggenberger, G., Zech, W., and Thomas, R. J. (1995). Lignin and carbohydrate alteration in particle-size separates of an oxisol under tropical pastures following native savanna. Soil Biol. Biochem. 27, 1629–1638. doi: 10.1016/0038-0717(95)00080-X
Haggar, J., Wightman, K., and Fisher, R. (1997). The potential of plantations to foster woody regeneration within a deforested landscape in lowland Costa Rica. For. Ecol. Manag. 99, 55–64. doi: 10.1016/S0378-1127(97)00194-1
Hansson, K., Olsson, B. A., Olsson, M., Johansson, U., and Kleja, D. B. (2011). Differences in soil properties in adjacent stands of Scots pine, Norway spruce and silver birch in SW Sweden. For. Ecol. Manag. 262, 522–530. doi: 10.1016/j.foreco.2011.04.021
Hartshorn, G. S., and Hammel, B. E. (1994). “Vegetation types and floristic patterns,” in La Selva: Ecology and Natural History of a Neotropical Rain Forest, eds L. A. McDade, K. S. Bawa, H. A. Hespenheide, and G. S. Hartshorn (Chicago, IL: University of Chicago Press), 73–89.
Hedges, J. I., Blanchette, R. A., Weliky, K., and Devol, A. H. (1988). Effects of fungal degradation on the CuO oxidation products of lignin: a controlled laboratory study. Geochim. Cosmochim. Acta 52, 2717–2726. doi: 10.1016/0016-7037(88)90040-3
Heim, A., Hofmann, A., and Schmidt, M. W. (2010). Forest-derived lignin biomarkers in an Australian oxisol decrease substantially after 90 years of pasture. Org. Geochem. 41, 1219–1224. doi: 10.1016/j.orggeochem.2010.07.005
Hetherington, S. L., and Anderson, J. M. (1998). Lignin signatures show the effects of changes in heather and bracken cover on the composition of organic matter in a moorland soil profile. Oecologia 117, 194–200. doi: 10.1007/s004420050648
SAS Institute Inc [SASI] (1999). SAS/STAT User’s Guide. Version 8. Cary, North Carolina, USA: SAS Institute.
Jenny, H. (1941). Factors of Soil Formation: A System of Quantitative Pedology. New York: McGraw-Hill.
Jobbágy, E. G., and Jackson, R. B. (2000). The vertical distribution of soil organic carbon and its relation to climate and vegetation. Ecol. Appl. 10, 423–436. doi: 10.1890/1051-07612000010[0423:TVDOSO]2.0.CO;2
Kivlin, S. N., and Hawkes, C. V. (2016a). Temporal and spatial variation of soil bacteria richness, composition, and function in a neotropical rainforest. PLoS One 11:e0159131. doi: 10.1371/journal.pone.0159131
Kivlin, S. N., and Hawkes, C. V. (2016b). Tree species, spatial heterogeneity, and seasonality drive soil fungal abundance, richness, and composition in Neotropical rainforests. Environ. Microbiol. 18, 4662–4673. doi: 10.1111/1462-2920.13342
Kleber, M., Nico, P. S., Plante, A., Filley, T., Kramer, M., Swanston, C., et al. (2011). Old and stable soil organic matter is not necessarily chemically recalcitrant: implications for modeling concepts and temperature sensitivity. Glob. Change Biol. 17, 1097–1107. doi: 10.1111/j.1365-2486.2010.02278.x
Kleber, M., Schwendenmann, L., Veldkamp, E., Rößner, J., and Jahn, R. (2007). Halloysite versus gibbsite: silicon cycling as a pedogenetic process in two lowland neotropical rain forest soils of La Selva, Costa Rica. Geoderma 138, 1–11. doi: 10.1016/j.geoderma.2006.10.004
Kögel, I. (1986). Estimation and decomposition pattern of the lignin component in forest humus layers. Soil Biol. Biochem. 18, 589–594. doi: 10.1016/0038-0717(86)90080-5
Kuzyakov, Y. (2010). Priming effects: interactions between living and dead organic matter. Soil Biol. Biochem. 42, 1363–1371. doi: 10.1016/j.soilbio.2010.04.003
Lehmann, J., and Kleber, M. (2015). The contentious nature of soil organic matter. Nature 528, 60–68. doi: 10.1038/nature16069
Lewis, S. L., Edwards, D. P., and Galbraith, D. (2015). Increasing human dominance of tropical forests. Science 349, 827–832. doi: 10.1126/science.aaa9932
Littell, R. C., Milliken, G. A., Stroup, W. W., Wolfinger, R. D., and Schabenberger, O. (1996). SAS System for Mixed Models. U.S.A.: SAS Publishing.
Ma, Y., McCormick, M. K., Szlavecz, K., and Filley, T. R. (2019). Controls on soil organic carbon stability and temperature sensitivity with increased aboveground litter input in deciduous forests of different forest ages. Soil Biol. Biochem. 134, 90–99. doi: 10.1016/j.soilbio.2019.03.020
Mayer, M., Prescott, C. E., Abaker, W. E., Augusto, L., Cécillon, L., Ferreira, G. W., et al. (2020). Influence of forest management activities on soil organic carbon stocks: a knowledge synthesis. For. Ecol. Manag. 466:118127. doi: 10.1016/j.foreco.2020.118127
McLauchlan, K. K., Hobbie, S. E., and Post, W. M. (2006). Conversion from agriculture to grassland builds soil organic matter on decadal timescales. Ecol. Appl. 16, 143–153. doi: 10.1890/04-1650
Melillo, J. M., Aber, J. D., and Muratore, J. F. (1982). Nitrogen and lignin control of hardwood leaf litter decomposition dynamics. Ecology 63, 621–626. doi: 10.2307/1936780
Mikutta, R., Schaumann, G. E., Gildemeister, D., Bonneville, S., Kramer, M. G., Chorover, J., et al. (2009). Biogeochemistry of mineral–organic associations across a long-term mineralogical soil gradient (0.3–4100 kyr), Hawaiian Islands. Geochim. Cosmochim. Acta 73, 2034–2060. doi: 10.1016/j.gca.2008.12.028
Neter, J., Kutner, M. H., Nachtsheim, C. J., and Wasserman, W. (1996). Applied Linear Statistical Models. Volume 4. Chicago: Irwin Chicago.
Opsahl, S., and Benner, R. (1995). Early diagenesis of vascular plant tissues: lignin and cutin decomposition and biogeochemical implications. Geochim. Cosmochim. Acta 59, 4889–4904. doi: 10.1016/0016-7037(95)00348-7
Osei, R., Titeux, H., Bielak, K., Bravo, F., Collet, C., Cools, C., et al. (2021). Tree species identity drives soil organic carbon storage more than species mixing in major two-species mixtures (pine, oak, beech) in Europe. For. Ecol. Manag. 481:118752. doi: 10.1016/j.foreco.2020.118752
Otto, A., and Simpson, M. J. (2006). Evaluation of CuO oxidation parameters for determining the source and stage of lignin degradation in soil. Biogeochemistry 80, 121–142. doi: 10.1007/s10533-006-9014-x
Paul, E. A., Morris, S. J., and Bohm, S. (2001). “The determination of soil C pool sizes and turnover rates: biophysical fractionation and tracers,” in Assessment Methods for Soil Carbon, eds R. Lal, J. M. Kimble, R. F. Follett, and B. A. Stewart (Boca Raton, FL: Lewis Publ.), 193–206. doi: 10.1201/9781482278644-24
Raich, J. W., Russell, A. E., and Bedoya-Arrieta, R. (2007). Lignin and enhanced litter turnover in tree plantations of lowland Costa Rica. For. Ecol. Manag. 239, 128–135. doi: 10.1016/j.foreco.2006.11.016
Raich, J. W., Russell, A. E., and Valverde-Barrantes, O. (2009). Fine root decay rates vary widely among lowland tropical tree species. Oecologia 161, 325–330. doi: 10.1007/s00442-009-1379-9
Raich, J. W., and Valverde-Barrantes, O. J. (2017). Soil CO2 Flux, Moisture, Temperature, and Litterfall, La Selva, Costa Rica, 2003-2010. ORNL DAAC, Oak Ridge, Tennessee, USA. doi: 10.3334/ORNLDAAC/1373
Rasse, D. P., Rumpel, C., and Dignac, M. F. (2005). Is soil carbon mostly root carbon? Mechanisms for a specific stabilisation. Plant Soil 269, 341–356. doi: 10.1007/s11104-004-0907-y
Russell, A. E., Cambardella, C. A., Ewel, J. J., and Parkin, T. B. (2004). Species, rotation, and life-form diversity effects on soil carbon in experimental tropical ecosystems. Ecol. Appl. 14, 47–60. doi: 10.1890/02-5299
Russell, A. E., Hall, S. J., and Raich, J. W. (2017). Tropical tree species traits drive soil cation dynamics via effects on pH: a proposed conceptual framework. Ecol. Monogr. 87, 685–701. doi: 10.1002/ecm.1274
Russell, A. E., Kivlin, S. N., and Hawkes, C. V. (2018). Tropical tree species effects on soil pH and biotic factors and the consequences for macroaggregate dynamics. Forests 9:184. doi: 10.3390/f9040184
Russell, A. E., Raich, J. W., Arrieta, R. B., Valverde-Barrantes, O., and González, E. (2010). Impacts of individual tree species on carbon dynamics in a moist tropical forest environment. Ecol. Appl. 20, 1087–1100. doi: 10.1890/09-0635.1
Russell, A. E., Raich, J. W., Valverde-Barrantes, O. J., and Fisher, R. F. (2007). Tree species effects on soil properties in experimental plantations in tropical moist forest. Soil Sci. Soc. Am. J. 71, 1389–1397. doi: 10.2136/sssaj2006.0069
Sanford, R. L., Paaby, P., Luvall, J. C., and Phillips, E. (1994). “Climate, geomorphology, and aquatic systems,” in La Selva: Ecology and Natural History of a Neotropical Rain Forest, eds L. A. McDade, K. S. Bawa, H. A. Hespenheide, and G. S. Hartshorn (Chicago, IL: University of Chicago Press), 19–33.
Schmidt, M. W., Torn, M. S., Abiven, S., Dittmar, T., Guggenberger, G., Janssens, I. A., et al. (2011). Persistence of soil organic matter as an ecosystem property. Nature 478, 49–56. doi: 10.1038/nature10386
Schuur, E. A. (2003). Productivity and global climate revisited: the sensitivity of tropical forest growth to precipitation. Ecology 84, 1165–1170.
Six, J., Callewaert, P., Lenders, S., De Gryze, S., Morris, S. J., Gregorich, E. G., et al. (2002). Measuring and understanding carbon storage in afforested soils by physical fractionation. Soil Sci. Soc. Am. J. 66, 1981–1987. doi: 10.2136/sssaj2002.1981
Spielvogel, S., Prietzel, J., Leide, J., Riedel, M., Zemke, J., and Kögel-Knabner, I. (2014). Distribution of cutin and suberin biomarkers under forest trees with different root systems. Plant Soil 381, 95–110. doi: 10.1007/s11104-014-2103-z
Swift, M. J., Heal, O. W., Anderson, J. M., and Anderson, J. M. (1979). Decomposition in Terrestrial Ecosystems. U. S. A.: University of California Press.
Valverde-Barrantes, O. J., Raich, J. W., and Russell, A. E. (2007). Fine-root mass, growth and nitrogen content for six tropical tree species. Plant Soil 290, 357–370. doi: 10.1007/s11104-006-9168-2
Valverde-Barrantes, O. J., Raich, J. W., and Russell, A. E. (2009). Erratum to Fine-root mass, growth and nitrogen content for six tropical tree species. Plant Soil 320, 333–334. doi: 10.1007/s11104-009-9996-y
Vancampenhout, K., Wouters, K., De Vos, B., Buurman, P., Swennen, R., and Deckers, J. (2009). Differences in chemical composition of soil organic matter in natural ecosystems from different climatic regions–A pyrolysis–GC/MS study. Soil Biol. Biochem. 41, 568–579. doi: 10.1016/j.soilbio.2008.12.023
Vesterdal, L., and Raulund-Rasmussen, K. (1998). Forest floor chemistry under seven tree species along a soil fertility gradient. Can. J. For. Res. 28, 1636–1647. doi: 10.1139/x98-140
Wiesmeier, M., Urbanski, L., Hobley, E., Lang, B., von Lützow, M., Marin-Spiotta, E., et al. (2019). Soil organic carbon storage as a key function of soils-A review of drivers and indicators at various scales. Geoderma 333, 149–162. doi: 10.1016/j.geoderma.2018.07.026
Keywords: fine root growth, phenolic compounds, soil carbon (C) storage, tropical tree species, soil organic carbon pools
Citation: Russell AE, Marek RF and Olk DC (2021) Tree Species of Wet Tropical Forests Differ in Their Tissue Biochemistry and Effects on Soil Carbon Dynamics. Front. For. Glob. Change 4:674213. doi: 10.3389/ffgc.2021.674213
Received: 28 February 2021; Accepted: 13 April 2021;
Published: 05 May 2021.
Edited by:
Laurent Augusto, INRA Centre Bordeaux-Aquitaine, FranceReviewed by:
Xiao-Tao Lu, Institute of Applied Ecology, Chinese Academy of Sciences (CAS), ChinaAntra Boca, Latvia University of Agriculture, Latvia
Copyright © 2021 Russell, Marek and Olk. This is an open-access article distributed under the terms of the Creative Commons Attribution License (CC BY). The use, distribution or reproduction in other forums is permitted, provided the original author(s) and the copyright owner(s) are credited and that the original publication in this journal is cited, in accordance with accepted academic practice. No use, distribution or reproduction is permitted which does not comply with these terms.
*Correspondence: Ann E. Russell, arussell@iastate.edu