Soil Enzyme Activities and Their Relationships With Soil C, N, and P in Peatlands From Different Types of Permafrost Regions, Northeast China
- 1Heilongjiang Province Key Laboratory of Geographical Environment Monitoring and Spatial Information Service in Cold Regions, Harbin Normal University, Harbin, China
- 2Key Laboratory of Wetland Ecology and Environment, Northeast Institute of Geography and Agroecology, Chinese Academy of Sciences, Changchun, China
Peatland is a key component of terrestrial ecosystems in permafrost regions and have important effects on climate warming. Soil enzymes are involved in biogeochemical cycle of soil carbon (C), nitrogen (N) and phosphorus (P), which can be used as early sensitive indicators of soil nutrient changes caused by climate change. To predict the possible effects of permafrost degradation on soil enzymes in peatlands, ten peatlands from three types of permafrost regions along the permafrost degradation sequence (predominantly continuous permafrost region-predominantly continuous and island permafrost region-sparsely island permafrost region) in northeast China were selected to examine the activities of soil invertase, β-glucosidase, urease and acid phosphatase and their relationships with soil physicochemical properties. The results demonstrated that permafrost type had significant effect on soil enzyme activities. Soil enzyme activities in predominantly continuous and island permafrost region were significantly higher than those in sparsely island permafrost region and predominantly continuous permafrost region. The activities of four soil enzymes were higher in 0–15 cm than 15–30 cm soil layer. Soil enzymes activities were positively correlated with soil ammonia nitrogen (NH4+-N), soil moisture content (SMC), total phosphorus (TP) and total nitrogen (TN), but negatively correlated with soil nitrate nitrogen (NO3−-N). Soil inorganic nitrogen and moisture contents were the main factors affecting soil enzyme activities, with NH4+-N accounted for 41.6% of the variance, SMC 29.6%, and NO3−-N 11.0%. These results suggested that permafrost degradation may change soil enzyme activities by changing soil physicochemical properties. In this study, only 0–30 cm peat soil in permafrost regions was collected during the complete thawing period of permafrost active layer, further studies should be placed on the change of soil enzyme activities in active layer and permafrost layer during freezing and thawing process in the southernmost location of northeast China in the Eurasia permafrost body and boreal forest belt.
Introduction
Permafrost is both the product of solar radiation balance and the water-heat exchanges in lithosphere-soil-atmosphere system, which remains at or below 0°C for at least two continuous years (Hugelius et al., 2014; Yuan et al., 2020). It is one of the key components of terrestrial ecosystem in cold regions, covering approximately 25% of land area in the northern hemisphere (Yang et al., 2010). The carbon stored in northern permafrost regions is approximately 1,672 Pg, which is nearly twice the amount of carbon currently in atmosphere (Tarnocai et al., 2009). As a product of cold climate, permafrost is extremely sensitive to climate change, thus has become the hotspots of global carbon cycle (Serreze et al., 2000; Solomon et al., 2007). Numerous studies reported that climate warming has caused rapid permafrost degradation, including the deepening of active layer, northward movement of permafrost boundary and remarkable reduction of the total permafrost area in northern high-latitude regions (Jin et al., 2007; Hollesen et al., 2011; Wisser et al., 2011). In the past 60 years, the southern boundary of predominantly continuous permafrost, predominantly continuous and island permafrost, and sparsely island permafrost regions moved northward by 0–3.4°, 0–5.5° and 0.4–1.1°, respectively; The total area of permafrost in northeast China decreased from 4.8 × 105 km2 to 3.1 × 105 km2; In the Da Xing’anling Mountains, especially in the north of 50°N, permafrost degenerated from predominantly continuous permafrost region to discontinuous or sparsely island permafrost region; The areas of three types of permafrost regions decreased by 90.00, 43.40 and 23.80%, respectively; The mean annual surface temperature of Da Xing’anling Mountains and Xiao Xing’anling Mountains increased by 1.2–1.4°C (Zhang et al., 2021). The changes of soil hydrothermal dynamics caused by permafrost degradation will affect soil biogeochemical cycle process (Li et al., 2021). It has been generally regarded that sustained warming can promote the decomposition of soil organic matter stored in permafrost into atmosphere as greenhouse gases, which will further accelerate climate warming (Melillo et al., 2002; Schuur et al., 2015; Mu et al., 2017). Furthermore, permafrost degradation may increase freeze-thaw disasters and impact on ecosystem structure and function (Guo and Wang, 2017). Approximately one third of terrestrial carbon pool accumulates in peatlands of boreal permafrost, which is considered as an important CO2 sink (Gorham, 1991). Researches showed that the global temperature has decreased by about 1.5–2.5°C due to this function in the past ten thousand years (Holden, 2005). However, the anoxic, cool, and wet conditions of peatland also contribute it to a primary natural source of CH4 emission (Frolking et al., 2011). According to statistics, the annual CH4 emission from peatland accounts for 4–10% of the total global CH4 emission (Mikaloff Fletcher et al., 2004). Climate warming has significantly affected the carbon stability of peatlands in permafrost regions (Cong et al., 2020). Therefore, it is necessary to clarify the biochemical process of this unique ecosystem.
Soil enzymes play an important role in maintaining peatland ecosystem quality, functional diversity, and nutrient cycling (Tabatabai, 1994; Kandeler et al., 1999; Sinsabaugh et al., 2002). The response of soil enzymes to both natural and anthropogenic factors is rapider than other soil variables. Hence, the activities of soil enzymes have been considered as the early and sensitive indicators to evaluate soil quality under the scenario of future global climatic change (Ladd, 1985; Miller and Dick, 1995; Theriot et al., 2013). Previous studies have been published on soil enzyme activities and their relationship with soil physicochemical properties in permafrost regions. Song et al. (2019) demonstrated that soil invertase, β-glucosidase, urease and acid phosphatase are highly correlated with soil total carbon (TC), TN, SMC and dissolved organic carbon (DOC) contents in predominantly continuous permafrost region of northeast China. Research in Tibetan Plateau also indicated that soil invertase activity is significantly correlated with SMC and soil organic carbon content (Xu et al., 2018).
Current reports on soil enzymes are mostly limited to a single type of permafrost, which was not conducive to the comprehensive understanding of soil enzymes responding to future permafrost degradation. This study selected three types of permafrost regions (predominantly continuous permafrost region-predominantly continuous and island permafrost region-sparsely island permafrost region) to simulate permafrost degradation based on the method of space-time substitution. These areas have experienced warming of more than 1°C over the past century (Jin et al., 2019). The fragmentation degree in spatial distribution of the permafrost is becoming more and more serious. The objectives of this study were: 1) To clarify the variation of soil enzyme activities in different types of permafrost regions; 2) To determine the relationship between soil enzyme activities and soil physicochemical properties; 3) To predict the possible effects of permafrost degradation on soil enzyme activities in peatlands in permafrost regions. The detailed data of sampling sites are given in Table 1.
Materials and Methods
Site Description and Peat Sampling
The selected areas (Da and Xiao Xing’anling Mountains) are located in northeast China. It is regarded to be the southern margin of Eurasian permafrost region (Wei et al., 2011). The mean annual air temperature is −5.5°C and the annual precipitation is 400 mm for Daxing’anling Mountains and 0.4°C, 630 mm for Xiaoxing'anling Mountains (Wang et al., 2012). A total of 10 peatlands were carefully selected and investigated, with four squares in sparsely island permafrost region, three squares in predominantly continuous and island permafrost region, and three squares in predominantly continuous permafrost region in August 2018 (Figure 1), including shrub-carex-sphagnum peatlands, larch-shrub-sphagnum peatlands, and shrub-carex peatlands. Permafrost in this region is largely ecosystem-driven or -protected, the degradation of permafrost is a relatively slow process. Compared with the time scale of the response of permafrost to climate change, the current monitoring data are far from describing the degradation process. In view of this, from the spatial variation of permafrost characteristics to analogy the temporal evolution process, a research idea of space-time substitution is formed.
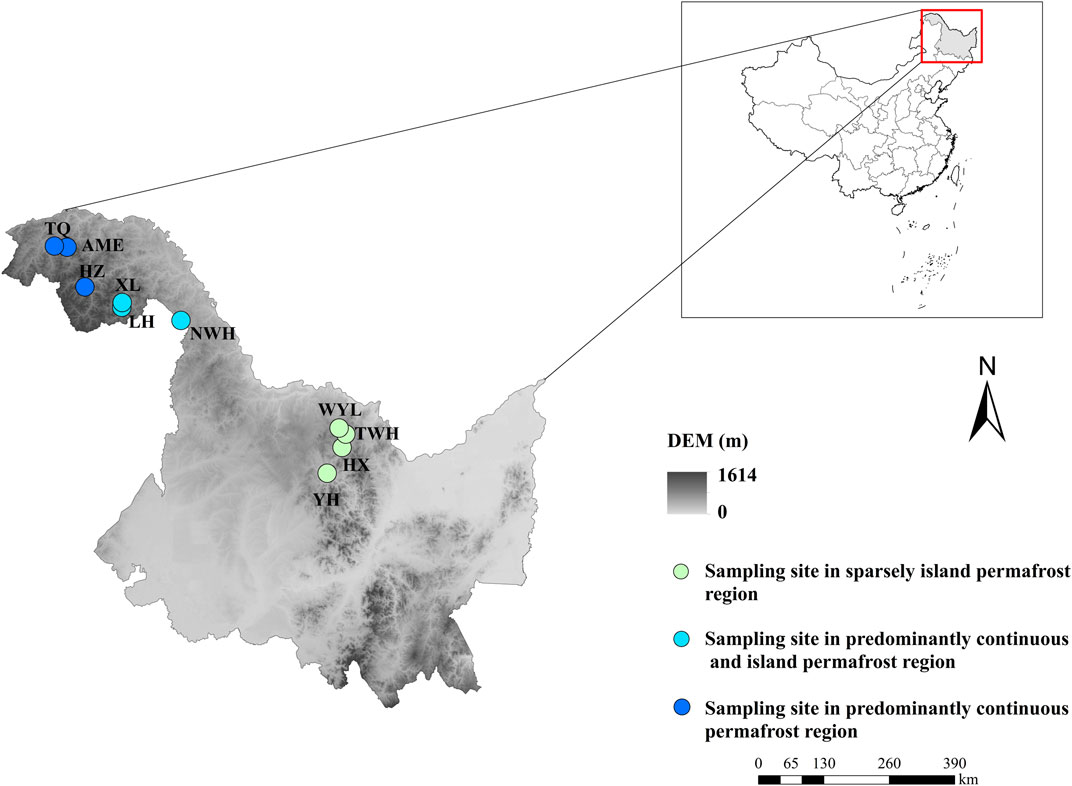
FIGURE 1. Spatial arrangement of sampling sites in northeast China. YH: Youhao; HX: Hongxing; TWH: Tangwanghe; WYL: Wuyiling; NWH: Nanwenghe; LH: Linhai; XL: Xinlin; HZ: Huzhong; AME: Amuer; TQ: Tuqiang.
Five soil cores of 0–15 cm and 15–30 cm were taken in each square using a soil core sampler after removing surface litter. Peat samples from the same soil layer were homogenized into composite samples and placed in zip-lock bags with headspaces removed and transported to the laboratory within 48–72 h. Following removal of visible plant and organic debris by hand picking, each fresh sample was sieved with a 4 mm mesh and divided into two subsamples. One subsample was stored at 4°C for determining soil enzyme activities, SMC, DOC, NH4+-N, and NO3−-N contents. The left subsample was air dried and then sieved through a 0.25 mm mesh to measure soil TC, TN, TP contents, and pH. The soil physicochemical properties and methods of analysis were previously reported in Song et al. (2020) and are analyzed here in conjunction with new soil enzymes data.
Soil Enzyme Assays
Table 2 shows the method of soil enzymes determination. To determine invertase activity, sucrose solution and pH 5.5 phosphate buffer were added to 2 g of fresh soil, and a few drops of toluene were added. The solution was shaken and incubated in a 37°C incubator for 24 h. Next, 3, 5-dinitrosalicylic acid was added to determine the glucose produced by a 508 nm spectrophotometry.
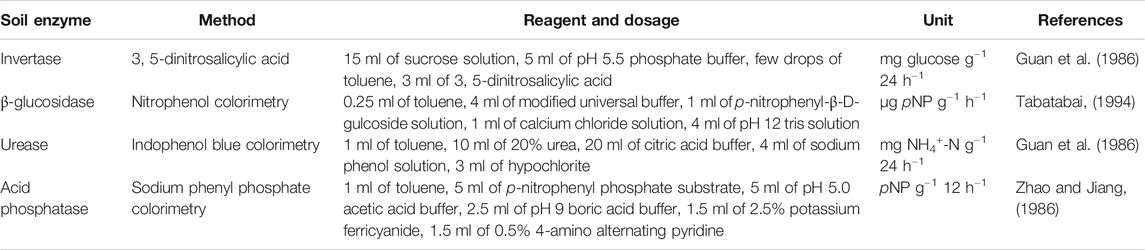
TABLE 2. Reagent and method details for soil invertase, β-glucosidase, urease, and acid phosphatase.
To determine β-glucosidase activity, 0.5 g of fresh soil was added to toluene and shaken for 15 min. Next, modified universal buffer and p-nitrophenyl-β-D-gulcoside solution were added, and the solution was incubated at 37°C for 1 h. Subsequently, calcium chloride solution and pH 12 tris solution were added, and the results were measured by colorimetry at 410 nm.
When urease was determined, 2 g of fresh soil was added to toluene, 20% urea, and citric acid buffer with a pH level of 6.7 then incubated at 37°C for 24 h. Then, sodium phenol and hypochlorite were added to determine the release of NH4+-N by colorimetry at 578 nm.
To determine acid phosphatase activity, 1 ml of toluene, 5 ml of p-nitrophenyl phosphate substrate, and 5 ml of pH 5.0 acetic acid buffer were added to 1 g of fresh soil and incubated at 37°C for 12 h. Next, 1 ml of the filtrate was taken and pH nine boric acid buffer, 2.5% potassium ferricyanide, and 0.5% 4-amino alternating pyridine were added. The produced pNP was determined colorimetrically at 570 nm.
Four replicates of each subsample for four enzymes were analyzed. Moreover, substrate-free and soil-free controls were added for each sample to account for nonenzymatic substrate hydrolysis.
Statistical Analysis
Data in the present study were presented as mean ± SE. The data were statistically analyzed via SPSS 16.0, with an accepted significance level of α = 0.05. Differences of soil enzymes activities among three permafrost regions were tested with One-way ANOVA. Two-way ANOVA was performed to analyze the effects of permafrost region type, soil layer and their interactions on soil enzyme activities. Applying Stepwise multiple linear regression analysis to derive optimal empirical models for predicting changes in soil enzyme activities. Redundancy analysis (RDA) was conducted using CANOCO software 5.0 to determine the relationship between soil enzyme activities and soil physicochemical properties. Figures were drawn by Origin 8 and CorelDRAW X4.
Results
Soil Enzyme Activities in Different Types of Permafrost Regions
Soil Invertase Activity
Permafrost type had significant influence on soil invertase activity (p < 0.05) (Table 3). Soil invertase activity increased first and then decreased along the permafrost degradation sequence. Soil invertase in predominantly continuous and island permafrost region (0–15 cm: 270.51 ± 40.26 mg glucose g−1 24 h−1, 15–30 cm: 259.13 ± 36.99 mg glucose g−1 24 h−1) were significantly higher than those in sparsely island permafrost region (0–15 cm: 77.70 ± 5.00 mg glucose g−1 24 h−1, 15–30 cm: 59.69 ± 10.02 mg glucose g−1 24 h−1) and predominantly continuous permafrost region (0–15 cm: 47.72 ± 6.67 mg glucose g−1 24 h−1, 15–30 cm: 41.40 ± 7.93 mg glucose g−1 24 h−1) (p < 0.05) (Figure 2). No significant difference was observed between sparsely island permafrost region and predominantly continuous permafrost region (p > 0.05). The activity of soil invertase decreased with depths in three types of permafrost regions, but soil layer had no significant effect on invertase activity (p > 0.05). In depth, the largest difference in soil invertase was found in the sparsely island permafrost region (18.01 mg glucose g−1 24 h−1), while the minimum difference was in predominantly continuous permafrost region (6.32 mg glucose g−1 24 h−1). Table 3 showed that there was no obvious influence of the interaction between permafrost type and soil layer on invertase activity (p > 0.05).

TABLE 3. Two-way ANOVA on the effects of permafrost region type, soil layer and their interaction effects on soil invertase, β-glucosidase, urease, and acid phosphatase activity.
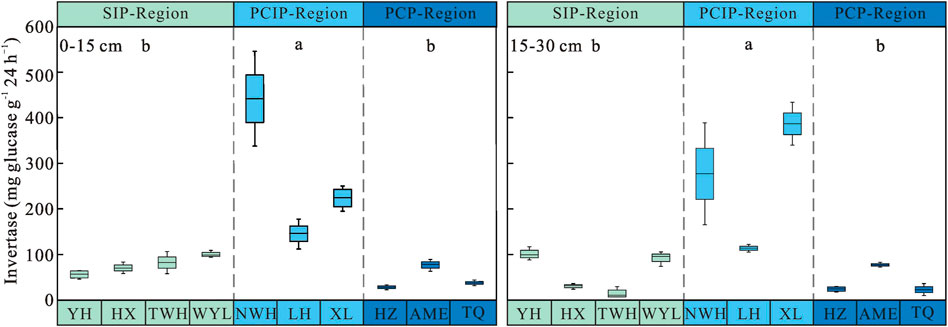
FIGURE 2. Soil invertase activity in peatlands from different types of permafrost regions. SIP-Region: sparsely island permafrost region; PCIP-Region: predominantly continuous and island permafrost region; PCP-Region: predominantly continuous permafrost region. Different lowercase letters in the figure indicate significant differences in the means between permafrost region types.
Soil β-glucosidase Activity
Soil β-glucosidase activity increased first and then decreased along the permafrost degradation sequence. The highest activity of soil β-glucosidase was observed in predominantly continuous and island permafrost region, and the activity of 0–15 cm and 15–30 cm soil layer was 1,273.31 ± 54.64 and 912.96 ± 49.38 µg pNP g−1 h−1, respectively, which was significantly higher than that in sparsely island permafrost region (0–15 cm: 566.52 ± 28.59 µg pNP g−1 h−1, 15–30 cm: 408.79 ± 44.14 µg pNP g−1 h−1) and predominantly continuous permafrost region (0–15 cm: 474.14 ± 31.86 µg pNP g−1 h−1, 15–30 cm: 428.18 ± 37.54 µg pNP g−1 h−1) (p < 0.05). No significant difference was found between sparsely island permafrost region and predominantly continuous permafrost region. As shown in Figure 3, soil β-glucosidase activity decreased with depth regardless of permafrost region type. Two-way ANOVA demonstrated that permafrost type (p < 0.001), soil layer (p < 0.001) and the interaction between permafrost region type and soil layer (p = 0.002) had significant effects on soil β-glucosidase activity. The influence intensity (F value) of each factor on soil β-glucosidase activity was in the order: permafrost type > soil layer > interaction (Table 3).
Soil Urease Activity
Soil urease activity showed decreasing trends from 0–15 cm to 15–30 cm soil layer in three permafrost regions (Figure 4). Along the permafrost degradation sequence, it increased first and then decreased in two soil layers. In 0–15 cm soil layer, the urease activity ranged from 1.69 ± 0.28 to 3.48 ± 0.19 mg NH4+-N g−1 24 h−1. The activity of urease in predominantly continuous and island permafrost region was significantly higher than other two permafrost regions (p < 0.05), no significant difference was observed between sparsely island permafrost region and predominantly continuous permafrost region (p > 0.05). In 15–30 cm soil layer, there were significant differences in soil urease activity among three different types of permafrost regions (p < 0.05). The urease activity in predominantly continuous and island permafrost region was the highest, which was 33.49 and 62.68% higher than that in predominantly continuous permafrost region and sparsely island permafrost region, respectively. As shown in Table 3, permafrost type (p < 0.001), soil layer (p < 0.001), and their interaction (p = 0.001) had significant effects on soil urease activity. The influence intensity (F value) of each factor on soil urease activity was in the order: soil layer > permafrost type > interaction.
Soil Acid Phosphatase Activity
Permafrost type (p < 0.001), soil layer (p < 0.001) and their interaction (p = 0.004) had significant effects on acid phosphatase activity (Table 3). Soil acid phosphatase activity declined with soil depths (Figure 5). Consistent with soil invertase, β-glucosidase and urease, soil acid phosphatase activity increased first and then decreased along the permafrost degradation sequence in 0–15 cm and 15–30 cm soil layers. For 0–15 cm, the highest acid phosphatase activity was observed in predominantly continuous and island permafrost region (36.88 ± 1.87 mg pNP g−1 12 h−1), which was significantly higher than that in sparsely island permafrost region (23.61 ± 1.03 mg pNP g−1 12 h−1) and predominantly continuous permafrost region (21.82 ± 1.21 mg pNP g−1 12 h−1) (p < 0.05). There was no significant difference between sparsely island permafrost region and predominantly continuous permafrost region (p > 0.05). For 15–30 cm, significant difference was found among three types of permafrost regions (p < 0.05). Acid phosphatase activity in predominantly continuous and island permafrost region was the highest (29.08 ± 1.33 mg pNP g−1 12 h−1), which was 1.53 times and 2.63 times of that in predominantly continuous permafrost region and sparsely island permafrost region, respectively.
Relationship Between Soil Enzyme Activities and Physicochemical Properties
Correlation Between Soil Enzymes Activities and Physicochemical Properties
RDA was used to ascertain the associations between soil enzyme activities and physicochemical properties (Figure 6). The first two components of RDA axes explained 94.08% of the variance of soil enzyme activities. Soil NH4+-N, SMC and NO3−-N were the most important variables, which could explain 41.6, 29.6 and 11.0% of the variation in four soil enzymes activities, respectively (Table 4). All of the four soil enzyme activities showed significantly positive correlation with soil NH4+-N, SMC, TP and TN (p < 0.01), while there were significantly negatively correlated between soil NO3−-N content and soil enzyme activities (p < 0.05). In addition, TC content was significantly positively correlated with soil β-glucosidase, urease, and acid phosphatase activity (p < 0.05) (Figure 6).
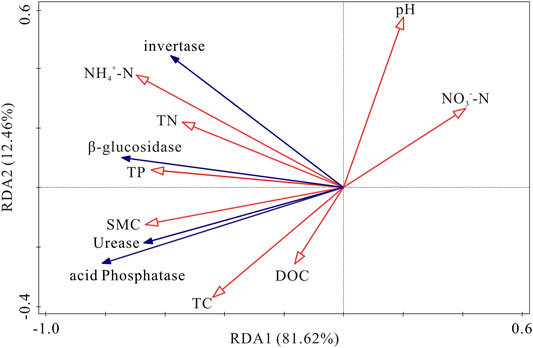
FIGURE 6. Redundancy analysis of soil enzymes activities and soil physicochemical properties. SMC: soil moisture content; TC: total carbon; DOC: dissolved organic carbon; TN: total nitrogen; NH4+-N: ammonia nitrogen; NO3--N: nitrate nitrogen; TP: total phosphorus.
Major Factors Affecting Soil Enzyme Activities
To remove multicollinearity, stepwise multiple linear regression analysis was used to quantitatively reveal the main soil physicochemical factors that controlled each soil enzyme activity variation in peatlands in permafrost regions (Table 5). The effects of different physicochemical properties on soil enzyme activities were quite different. According to regression equations, the influence factors of soil invertase were soil pH, NO3−-N, TN, SMC, NH4+-N and TC contents; The variation of β-glucosidase activity was explained by soil NO3−-N, SMC and NH4+-N contents; Soil pH, NO3−-N, TP and NH4+-N contents accounted for the variations of urease activity; Soil NO3−-N, TP, SMC, TC, and NH4+-N contents controlled the variance of acid phosphatase activity.

TABLE 5. Stepwise linear regression equation of soil enzyme activities and soil physicochemical properties.
Discussion
Variation in Soil Enzyme Activities From Different Permafrost Regions
As previous surveys on peatlands in northeast China (Wang et al., 2014; Song et al., 2018; Song et al., 2019), the activities of soil invertase, β-glucosidase, urease and acid phosphatase in this study were also much higher than those in alpine steppe ecosystem of the Qinghai Tibet Plateau (Wu et al., 2012; Xu et al., 2015) and forest permafrost region in Catalonia (Sardans and Peñuelas, 2005). Because of the anaerobic conditions and high productivity, amount of soil organic matter accumulated in peatlands (Dutta et al., 2006), which was beneficial for microorganisms to secrete more enzymes, further indicating the importance of peatlands in terrestrial carbon cycle. Consistent with our initial hypothesis, the permafrost region type had marked effect on soil enzyme activities, this probably due to the differences of freezing and thawing environment in three types of permafrost regions. Freezing and thawing process could affect the distribution of available substrates of soil enzymes (Wang et al., 2012). At the same time, the cracking caused by soil freezing-thawing cycle and the differential frost heave caused by soil hydrothermal gradient may lead to the mixing of different soil layers, and the soil substrates in the surface layer is churned down to the lower active layer and the upper permafrost (Bockheim et al., 1998; Ping et al., 2008). Microbial communities and enzymes were influenced by the supply and spatial distribution of nutrients (Allison, 2005; Bergstrom et al., 1998). Furthermore, changes in the distribution of permafrost have significantly changed the hydrology in cold regions (Walker et al., 2003; Zhang et al., 2013), this is mainly because the change of active layer depth changes soil water holding capacity, soil infiltration capacity and soil water conductivity, and redistribution of water in soil profile (Wang et al., 2009). In turn, these changes may lead to the change of vegetation cover and the distribution of soil substrates, thus affecting soil enzyme activities (Ishikawa et al., 2006; Yamazaki et al., 2006). The activities of four soil enzymes were all highest in predominantly continuous and island permafrost region, indicating that this region might have stronger soil mineralization rate of carbon, nitrogen and phosphorus and better capacity of nutrient supply (Qian et al., 2014). In addition, NH4+-N, SMC and NO3−-N were the most important factors affecting soil enzyme activities (Figure 6), implying that permafrost region type might affect soil enzyme activity by changing the content of soil moisture and inorganic nitrogen. The results of Song et al. (2017) demonstrated that permafrost degradation may influence soil nutrient availability, further change the activities of soil enzyme and its relationship with soil environmental conditions. The possible reason for the phenomenon that soil layer had strong impact on soil enzyme activity in this study was the variation in distribution of microorganism in profile. Our previous studies have pointed out that the abundance of soil microorganisms showed a downward trend with the increase of soil depth (Song et al., 2020).
Effects of Soil Physicochemical Properties on Soil Enzyme Activities
Relationship Between Soil Invertase Activity and Physicochemical Properties
Consistent with previous findings (Ciarkowska et al., 2014; Zhang et al., 2015; Feng et al., 2019), our results indicated that soil invertase activity was significantly enhanced with the increase of soil TN content. TN can increase the biomass of aboveground and underground roots and then promote the growth of rhizosphere microorganisms, thereby promoting soil invertase activity level (Li et al., 2018). Soil NH4+-N and NO3−-N are the main nitrogen forms absorbed by plants as well as the prerequisite for plant growth and development. Soil invertase was significantly positive associated with soil NH4+-N content, which was agreed with the results of Tan et al. (2014). The negative correlation between soil invertase activity and soil NO3−-N content might due to the opposite ionic charges of NH4+-N and NO3−-N, which have contrasting effects on litter decomposition, thus affecting soil invertase activity (Currey et al., 2010). These results further confirmed the critical role of soil invertase in soil nitrogen cycle in natural environment. Soil invertase activity reflects the pattern of soil organic carbon accumulation and decomposition (Paz-Ferreiro et al., 2011), which is generally used as an indicator of soil carbon cycle (Zhong et al., 2019). However, the correlation between soil invertase activity and TC content was not obvious in the present study. Similar results were found in non-cultivated land of Europe (Gianfreda et al., 2005). Variation in soil organic carbon fractions were found in different types of permafrost regions by Wang et al. (2012). The differences in sources of substrates availability and composition in different soil types might lead to different behavior of invertase (Katsalirou et al., 2010). The positive correlation between soil enzymes and water content reflected that invertase has the relatively strong ability to catalyze the hydrolysis of disaccharide to monosaccharide, which was a soluble soil carbohydrate and strongly affected by soil moisture content (Chendrayan et al., 1980; Li et al., 2018). In addition, low water content might inhibit soil invertase activity by limiting the growth and reproduction of soil microorganisms (Hooker and Stark, 2008).
Relationship Between Soil β-Glucosidase Activity and Physicochemical Properties
Soil β-glucosidase was involved in soil carbon cycle, which was closely related to the composition, transformation and circulation of soil organic matter (Eivazi and Tabatabai, 1990; Alvear et al., 2005; Bastida et al., 2006; Melero et al., 2009). However, stepwise regression analysis demonstrated that the main factor affecting β-glucosidase was available nitrogen content. Studies in the same region also reported that there was strongly correlation between β-glucosidase activity and inorganic nitrogen content (Song et al., 2019). Peatlands in permafrost regions in northeast China were generally nitrogen-poor ecosystem (Wang et al., 2012). β-glucosidase activity was significantly affected by N in such areas due to the deficiency of nitrogen, which would limit microbial activity and then decrease enzyme activity level (Sistla et al., 2012). In this way, the seemingly strange event above could be explained. Same as the mainstream researches (Sardans and Peñuelas, 2005; Pascual et al., 2007; Sardans et al., 2008), we found SMC was one of the influencing factors of soil β-glucosidase activity, which had a significant positive correlation with soil β-glucosidase. Soil moisture was the limiting factor of β-glucosidase activity because it could affect the osmotic of microbial cells and lead to cell death (Lamersdorf et al., 1998). In addition, soil water content has nonnegligible effects on the soil variables such as soil substrate, the diffusion of gases, soil pH, and temperature, indirectly regulating the activity of β-glucosidase (Schimel et al., 2007).
Relationship Between Soil Urease Activity and Physicochemical Properties
Urease was involved in soil nitrogen cycle, which could promote the hydrolysis of urea and produce ammonia. In this study, soil urease activity exhibited a positive correlation with soil NH4+-N content. Similar results were observed by Li et al. (2018) and Tan et al. (2014). Typically, inorganic nitrogen supplied for the growth of plant, thus soil urease activity was used to be indicator for reflecting the transformation of nitrogen (Pan et al., 2013). In line with the results of Guo et al. (2019), there was a significant negative correlation between urease activity and NO3−-N content in this study, which mainly because NO3−-N could inhibit nitrification process, thereby affecting the activity of urease involved in nitrogen cycle (Li et al., 2018). Additionally, our results showed that soil TP content had a marked positive correlation with urease activity, which was in agreement with the consequence reported by Qian et al. (2014). Song et al. (2020) suggested that microbial abundance associated with N-cycle can be considered as important indicators of soil phosphorus status in peatlands in permafrost regions. Moreover, TP might indirectly change soil urease activity by affecting soil pH, the state of ionization and the availability of substrates (Singh and Nye, 1984; Dick et al., 1988; Zhang et al., 2014).
Relationship Between Soil Acid Phosphatase Activity and Physicochemical Properties
Soil phosphatase played a key role in the transformation of soil phosphorus, which could convert organic phosphate into plant-absorbable phosphorus. In our research regions, soil pH ranged from 4.44 to 4.76. Generally speaking, the role of acid phosphatase in the P-cycle was more concerned in acid environmental soil. In accord with previous studies, the observed positive correlations between acid phosphatase activity and soil TP (Gianfreda et al., 2005; Redel et al., 2008), SMC (Sardans and Peñuelas, 2005; Redel et al., 2008), TC (Xu et al., 2018) were found in current study. Soil enzymes were mainly produced by soil microorganisms, plant roots and litters (Xu et al., 2010). The multiplication of microbes depended on SMC, and the suitable condition of soil moisture favored soil enzyme production (Hooker and Stark, 2008). Although acid phosphatase is not directly involved in carbon turnover, soil TC content is still an important factor affecting soil acid phosphatase. Soil TC is a carrier of active nutrients, which provides suitable conditions for soil enzymes (Qi et al., 2009; Li et al., 2018). Soil microorganisms can obtain relatively scarce resources by optimizing the allocation of carbon, nitrogen and phosphorus in soil enzyme synthesis process (Sinsabaugh and Moorhead, 1994), indicating that if one of these becomes relatively limited, microbial communities might consume others to secrete enzymes required (Olander and Vitousek, 2000; Allison, 2005; Marklein and Spektor, 2012), thus led to the complex relationship between acid phosphatase and inorganic nitrogen in our study. This fully illustrated that the supply of substrate and the spatial distribution of nutrients would affect the growth of microorganisms and regulate the synthesis of soil acid phosphatase (Bergstrom et al., 1998; Allison, 2005; Gong et al., 2015).
Conclusions
Peat samples were collected from permafrost regions along a degenerate gradient in northeast China based on the method of space-time substitution to explore the potential response of soil enzymes activities to permafrost degradation and their relationships with soil C, N and P. The results showed that soil enzyme activities were significantly correlated with soil substrate, and NH4+-N, SMC and NO3−-N were the main factors affecting soil enzymes activities in peatlands in permafrost regions. Permafrost region types had significant effects on the soil enzyme activities, and the activities of four soil enzymes were all the highest in the predominantly continuous and island permafrost region, indicating that the degradation from predominantly continuous permafrost to sparsely island permafrost was accompanied by the increase of nutrient supply and element turnover. Therefore, soil enzymes could provide potential feedback for permafrost degradation under the background of global climate change in some sense. Our study demonstrated that permafrost degradation might affect soil enzyme by changing soil physicochemical properties and the soil enzyme could be used as an effective indicator for evaluating the function of peatlands in permafrost regions. In this study, only 0–30 cm soil was collected during the complete thawing period of permafrost active layer, however, the change of peat soil enzyme activity during freezing and thawing process are of great significance in the biogeochemical cycle of permafrost. Further researches are needed to assess the change of soil enzyme activity in active layer and permafrost layer under the background of permafrost degradation.
Data Availability Statement
The original contributions presented in the study are included in the article/Supplementary Material, further inquiries can be directed to the corresponding authors.
Author Contributions
CL: original draft preparation, data curation. YS: manuscript revised, methodology, funding acquisition. XD: investigation, methodology. XW: investigation. XM: investigation. GZ: methodology. SZ: methodology.
Funding
This work was supported by the National Natural Science Foundation of China (No. 41871090).
Conflict of Interest
The authors declare that the research was conducted in the absence of any commercial or financial relationships that could be construed as a potential conflict of interest.
The handling editor declared a shared affiliation with several of the authors XW, YS, XM at time of review.
References
Allison, S. D. (2005). Cheaters, Diffusion and Nutrients Constrain Decomposition by Microbial Enzymes in Spatially Structured Environments. Ecol. Lett. 8 (6), 626–635. doi:10.1111/j.1461-0248.2005.00756.x
Alvear, M., Rosas, A., Rouanet, J. L., and Borie, F. (2005). Effects of Three Soil Tillage Systems on Some Biological Activities in an Ultisol from Southern Chile. Soil Tillage Res. 82 (2), 195–202. doi:10.1016/j.still.2004.06.002
Bastida, F., Luis Moreno, J., Teresa Hernández, T., and García, C. (2006). Microbiological Degradation index of Soils in a Semiarid Climate. Soil Biol. Biochem. 38 (12), 3463–3473. doi:10.1016/j.soilbio.2006.06.001
Bergstrom, D. W., Monreal, C. M., and King, D. J. (1998). Sensitivity of Soil Enzyme Activities to Conservation Practices. Soil Sci. Soc. America J. 62 (5), 1286–1295. doi:10.2136/sssaj1998.03615995006200050020x
Bockheim, J. G., Walker, D. A., Everett, L. R., Nelson, F. E., and Shiklomanov, N. I. (1998). Soils and Cryoturbation in Moist Nonacidic and Acidic Tundra in the Kuparuk River Basin, Arctic Alaska, U.S.A. Arctic Alpine Res. 30 (2), 166–174. doi:10.2307/1552131
Chendrayan, K., Adhya, T. K., and Sethunathan, N. (1980). Dehydrogenase and Invertase Activities of Flooded Soils. Soil Biol. Biochem. 12 (3), 271–273. doi:10.1016/0038-0717(80)90073-5
Ciarkowska, K., Sołek-Podwika, K., and Wieczorek, J. (2014). Enzyme Activity as an Indicator of Soil-Rehabilitation Processes at a Zinc and lead Ore Mining and Processing Area. J. Environ. Manage. 132, 250–256. doi:10.1016/j.jenvman.2013.10.022
Cong, J., Gao, C., Han, D., Li, Y., and Wang, G. (2020). Stability of the Permafrost Peatlands Carbon Pool under Climate Change and Wildfires during the Last 150 Years in the Northern Great Khingan Mountains, China. Sci. Total Environ. 712, 136476. doi:10.1016/j.scitotenv.2019.136476
Currey, P. M., Johnson, D., Sheppard, L. J., Leith, I. D., Toberman, H., Van Der Wal, R., et al. (2010). Turnover of Labile and Recalcitrant Soil Carbon Differ in Response to Nitrate and Ammonium Deposition in an Ombrotrophic Peatland. Glob. Change Biol 16 (8), 2307–2321. doi:10.1111/j.1365-2486.2009.02082.x
Dick, R. P., Rasmussen, P. E., and Kerle, E. A. (1988). Influence of Long-Term Residue Management on Soil Enzyme Activities in Relation to Soil Chemical Properties of a Wheat-Fallow System. Biol. Fert Soils 6 (2), 159–164. doi:10.1007/BF00257667
Dutta, K., Schuur, E. A. G., Neff, J. C., and Zimov, S. A. (2006). Potential Carbon Release from Permafrost Soils of Northeastern Siberia. Glob. Change Biol 12 (12), 2336–2351. doi:10.1111/j.1365-2486.2006.01259.x
Eivazi, F., and Tabatabai, M. A. (1990). Factors Affecting Glucosidase and Galactosidase Activities in Soils. Soil Biol. Biochem. 22 (7), 891–897. doi:10.1016/0038-0717(90)90126-K
Feng, C., Ma, Y., Jin, X., Wang, Z., Ma, Y., Fu, S., et al. (2019). Soil Enzyme Activities Increase Following Restoration of Degraded Subtropical Forests. Geoderma 351, 180–187. doi:10.1016/j.geoderma.2019.05.006
Frolking, S., Talbot, J., Jones, M. C., Treat, C. C., Kauffman, J. B., Tuittila, E.-S., et al. (2011). Peatlands in the Earth's 21st century Climate System. Environ. Rev. 19 (NA), 371–396. doi:10.1139/a11-014
Gianfreda, L., Antonietta Rao, M., Piotrowska, A., Palumbo, G., and Colombo, C. (2005). Soil Enzyme Activities as Affected by Anthropogenic Alterations: Intensive Agricultural Practices and Organic Pollution. Sci. Total Environ. 341 (1-3), 265–279. doi:10.1016/j.scitotenv.2004.10.005
Gong, S., Zhang, T., Guo, R., Cao, H., Shi, L., Guo, J., et al. (2015). Response of Soil Enzyme Activity to Warming and Nitrogen Addition in a Meadow Steppe. Soil Res. 53 (3), 242–252. doi:10.1071/SR14140
Gorham, E. (1991). Northern Peatlands: Role in the Carbon Cycle and Probable Responses to Climatic Warming. Ecol. Appl. 1 (2), 182–195. doi:10.2307/1941811
Guan, S. Y., Zhang, D. S., and Zhang, Z. M. (1986). Soil Enzyme and its Research Methods. Beijing: Agricultural, 274–297.
Guo, C., Li, J., Li, H., and Li, Y. (2019). Influences of Stormwater Concentration Infiltration on Soil Nitrogen, Phosphorus, TOC and Their Relations with Enzyme Activity in Rain Garden. Chemosphere 233, 207–215. doi:10.1016/j.chemosphere.2019.05.236
Guo, D., and Wang, H. (2017). Permafrost Degradation and Associated Ground Settlement Estimation under 2 °C Global Warming. Clim. Dyn. 49 (7), 2569–2583. doi:10.1007/s00382-016-3469-9
Holden, J. (2005). Peatland Hydrology and Carbon Release: Why Small-Scale Process Matters. Phil. Trans. R. Soc. A. 363 (1837), 2891–2913. doi:10.1098/rsta.2005.1671
Hollesen, J., Elberling, B., and Jansson, P. E. (2011). Future Active Layer Dynamics and Carbon Dioxide Production from Thawing Permafrost Layers in Northeast Greenland. Glob. Change Biol 17 (2), 911–926. doi:10.1111/j.1365-2486.2010.02256.x
Hooker, T. D., and Stark, J. M. (2008). Soil C and N Cycling in Three Semiarid Vegetation Types: Response to an In Situ Pulse of Plant Detritus. Soil Biol. Biochem. 40 (10), 2678–2685. doi:10.1016/j.soilbio.2008.07.015
Hugelius, G., Strauss, J., Zubrzycki, S., Harden, J. W., Schuur, E. A. G., Ping, C.-L., et al. (2014). Estimated Stocks of Circumpolar Permafrost Carbon with Quantified Uncertainty Ranges and Identified Data Gaps. Biogeosciences 11 (23), 6573–6593. doi:10.5194/bg-11-6573-2014
Ishikawa, M., Zhang, Y., Kadota, T., and Ohata, T. (2006). Hydrothermal Regimes of the Dry Active Layer. Water Resour. Res. 42 (4), W04401. doi:10.1029/2005WR004200110
Jin, H., Jin, X., He, R., Luo, D., Chang, X., Wang, S., et al. (2019). Evolution of Permafrost in China during the Last 20 Ka. Sci. China Earth Sci. 62 (8), 1207–1223. doi:10.1007/s11430-018-9272-0
Jin, H., Yu, Q., Lü, L., Guo, D., He, R., Yu, S., et al. (2007). Degradation of Permafrost in the Xing'anling Mountains, Northeastern China. Permafrost Periglac. Process. 18 (3), 245–258. doi:10.1002/ppp.589
Kandeler, E., Stemmer, M., and Klimanek, E.-M. (1999). Response of Soil Microbial Biomass, Urease and Xylanase within Particle Size Fractions to Long-Term Soil Management. Soil Biol. Biochem. 31 (2), 261–273. doi:10.1016/S0038-0717(98)00115-1
Katsalirou, E., Deng, S., Nofziger, D. L., and Gerakis, A. (2010). Long-term Management Effects on Organic C and N Pools and Activities of C-Transforming Enzymes in Prairie Soils. Eur. J. Soil Biol. 46 (5), 335–341. doi:10.1016/j.ejsobi.2010.06.004
Ladd, J. N. (1985). Soil Enzymes Soil Organic Matter and Biological Activity. Dordrecht: Springer, 175–221. doi:10.1007/978-94-009-5105-1_6
Lamersdorf, N. P., Beier, C., Blanck, K., Bredemeier, M., Cummins, T., Farrell, E. P., et al. (1998). Effect of Drought Experiments Using Roof Installations on Acidification/nitrification of Soils. For. Ecol. Manage. 101 (1-3), 95–109. doi:10.1016/S0378-1127(97)00128-X
Li, J., Tong, X., Awasthi, M. K., Wu, F., Ha, S., Ma, J., et al. (2018). Dynamics of Soil Microbial Biomass and Enzyme Activities along a Chronosequence of Desertified Land Revegetation. Ecol. Eng. 111, 22–30. doi:10.1016/j.ecoleng.2017.11.006
Li, X.-Y., Jin, H.-J., Wang, H.-W., Marchenko, S. S., Shan, W., Luo, D.-L., et al. (2021). Influences of forest Fires on the Permafrost Environment: A Review. Adv. Clim. Change Res. 12 (1), 48–65. doi:10.1016/j.accre.2021.01.001Marklein
Marklein, A. R., and Spektor, V. (2012). Nitrogen Inputs Accelerate Phosphorus Cycling Rates across a Wide Variety of Terrestrial Ecosystems. New Phytol. 193 (3), 696–704. doi:10.1111/j.1469-8137.2011.03967.xMelero
Melero, S., Li, X.-Y., Jia, N., and Moreno, F. (2009). Conservation Tillage: Short-And Long-Term Effects on Soil Carbon Fractions and Enzymatic Activities under Mediterranean Conditions. Adv. Clim. Change Res. 104 (2), 292–298. doi:10.1016/j.still.2009.04.001
Melillo, J. M., Steudler, P. A., Aber, J. D., Newkirk, K., Lux, H., Bowles, F. P., et al. (2002). Soil Warming and Carbon-Cycle Feedbacks to the Climate System. Science 298 (5601), 2173–2176. doi:10.1126/science.1074153
Mikaloff Fletcher, S. E., Tans, P. P., Bruhwiler, L. M., Miller, J. B., and Heimann, M. (2004). CH4sources Estimated from Atmospheric Observations of CH4and its13C/12C Isotopic Ratios: 1. Inverse Modeling of Source Processes. Glob. Biogeochem. Cycles 18 (4), a–n. doi:10.1029/2004GB002223
Miller, M., and Dick, R. P. (1995). Thermal Stability and Activities of Soil Enzymes as Influenced by Crop Rotations. Soil Biol. Biochem. 27 (9), 1161–1166. doi:10.1016/0038-0717(95)00045-G
Mu, C., Zhang, T., Zhao, Q., Su, H., Wang, S., Cao, B., et al. (2017). Permafrost Affects Carbon Exchange and its Response to Experimental Warming on the Northern Qinghai-Tibetan Plateau. Agric. For. Meteorology 247, 252–259. doi:10.1016/j.agrformet.2017.08.009
Olander, L. P., and Vitousek, P. M. (2000). Regulation of Soil Phosphatase and Chitinase Activityby N and P Availability. Biogeochemistry 49 (2), 175–191. doi:10.1023/A:1006316117817
Pan, C., Liu, C., Zhao, H., and Wang, Y. (2013). Changes of Soil Physico-Chemical Properties and Enzyme Activities in Relation to Grassland Salinization. Eur. J. Soil Biol. 55, 13–19. doi:10.1016/j.ejsobi.2012.09.009
Pascual, I., Antolín, M., García, C., Polo, A., and Sanchezdiaz, M. (2007). Effect of Water Deficit on Microbial Characteristics in Soil Amended with Sewage Sludge or Inorganic Fertilizer under Laboratory Conditions. Bioresour. Tech. 98 (1), 29–37. doi:10.1016/j.biortech.2005.11.026
Paz-Ferreiro, J., Trasar-Cepeda, C., Leirós, M. d. C., Seoane, S., and Gil-Sotres, F. (2011). Intra-annual Variation in Biochemical Properties and the Biochemical Equilibrium of Different Grassland Soils under Contrasting Management and Climate. Biol. Fertil. Soils 47 (6), 633–645. doi:10.1007/s00374-011-0570-4
Ping, C.-L., Michaelson, G. J., Jorgenson, M. T., Kimble, J. M., Epstein, H., Romanovsky, V. E., et al. (2008). High Stocks of Soil Organic Carbon in the North American Arctic Region. Nat. Geosci 1 (9), 615–619. doi:10.1038/ngeo284
Qi, Y., Darilek, J. L., Huang, B., Zhao, Y., Sun, W., and Gu, Z. (2009). Evaluating Soil Quality Indices in an Agricultural Region of Jiangsu Province, China. Geoderma 149 (3-4), 325–334. doi:10.1016/j.geoderma.2008.12.015
Qian, X., Gu, J., Sun, W., Li, Y.-D., Fu, Q.-X., Wang, X.-J., et al. (2014). Changes in the Soil Nutrient Levels, Enzyme Activities, Microbial Community Function, and Structure during Apple Orchard Maturation. Appl. Soil Ecol. 77, 18–25. doi:10.1016/j.apsoil.2014.01.003
Redel, Y., Rubio, R., Godoy, R., and Borie, F. (2008). Phosphorus Fractions and Phosphatase Activity in an Andisol under Different forest Ecosystems. Geoderma 145 (3-4), 216–221. doi:10.1016/j.geoderma.2008.03.007
Sardans, J., and Peñuelas, J. (2005). Drought Decreases Soil Enzyme Activity in a Mediterranean Quercus ilex L. forest. Soil Biol. Biochem. 37 (3), 455–461. doi:10.1016/j.soilbio.2004.08.004
Sardans, J., Peñuelas, J., and Estiarte, M. (2008). Changes in Soil Enzymes Related to C and N Cycle and in Soil C and N Content under Prolonged Warming and Drought in a Mediterranean Shrubland. Appl. Soil Ecol. 39 (2), 223–235. doi:10.1016/j.apsoil.2007.12.011
Schimel, J., Balser, T. C., and Wallenstein, M. (2007). Microbial Stress-Response Physiology and its Implications for Ecosystem Function. Ecology 88 (6), 1386–1394. doi:10.1890/06-0219
Schuur, E. A. G., McGuire, A. D., Schädel, C., Grosse, G., Harden, J. W., Hayes, D. J., et al. (2015). Climate Change and the Permafrost Carbon Feedback. Nature 520 (7546), 171–179. doi:10.1038/nature14338
Serreze, M. C., Walsh, J. E., Chapin III, F. S., Osterkamp, T., Dyurgerov, M., Romanovsky, V., et al. (2000). Observational Evidence of Recent Change in the Northern High-Latitude Environment. Clim. Change 46 (1), 159–207. doi:10.1023/A:1005504031923
Singh, R., and Nye, P. H. (1984). The Effect of Soil pH and High Urea Concentrations on Urease Activity in Soil. J. Soil Sci. 35 (4), 519–527. doi:10.1111/j.1365-2389.1984.tb00609.x
Sinsabaugh, R. L., Carreiro, M. M., and Repert, D. A. (2002). Allocation of Extracellular Enzymatic Activity in Relation to Litter Composition, N Deposition, and Mass Loss. Biogeochemistry 60 (1), 1–24. doi:10.1023/A:1016541114786
Sinsabaugh, R. L., and Moorhead, D. L. (1994). Resource Allocation to Extracellular Enzyme Production: a Model for Nitrogen and Phosphorus Control of Litter Decomposition. Soil Biol. Biochem. 26 (10), 1305–1311. doi:10.1016/0038-0717(94)90211-9
Sistla, S. A., Asao, S., and Schimel, J. P. (2012). Detecting Microbial N-Limitation in Tussock Tundra Soil: Implications for Arctic Soil Organic Carbon Cycling. Soil Biol. Biochem. 55, 78–84. doi:10.1016/j.soilbio.2012.06.010
Solomon, S., Manning, M., Marquis, M., and Qin, D. H. (2007). Climate Change 2007: The Physical Science Basis. Cambridge: Cambridge University Press. doi:10.1016/S0925-7721(01)00003-7
Song, Y., Liu, C., Wang, X., Ma, X., Jiang, L., Zhu, J., et al. (2020). Microbial Abundance as an Indicator of Soil Carbon and Nitrogen Nutrient in Permafrost Peatlands. Ecol. Indicators 115, 106362. doi:10.1016/j.ecolind.2020.106362
Song, Y., Song, C., Hou, A., Ren, J., Wang, X., Cui, Q., et al. (2018). Effects of Temperature and Root Additions on Soil Carbon and Nitrogen Mineralization in a Predominantly Permafrost Peatland. Catena 165, 381–389. doi:10.1016/j.catena.2018.02.026
Song, Y., Song, C., Shi, F., Wang, M., Ren, J., Wang, X., et al. (2019). Linking Plant Community Composition with the Soil C Pool, N Availability and Enzyme Activity in Boreal Peatlands of Northeast China. Appl. Soil Ecol. 140, 144–154. doi:10.1016/j.apsoil.2019.04.019
Song, Y. Y., Song, C. C., Wang, J. Y., Wang, X. W., and Meng, H. N. (2017). Variation of Soil Enzyme Activities in Wetlands from Permafrost to Seasonally Frozen Soil Region in Northeast China. Fresen. Environ. Bull. 26 (4), 2869–2878.
Tabatabai, M. A. (1994). Soil Enzymes. Methods Soil Anal. Part 2 Microbiol. Biochem. Properties 5, 775–833. doi:10.1016/j.ecolind.2020.106362
Tan, B., Wu, F.-z., Yang, W.-q., and He, X.-h. (2014). Snow Removal Alters Soil Microbial Biomass and Enzyme Activity in a Tibetan alpine forest. Appl. Soil Ecol. 76, 34–41. doi:10.1016/j.apsoil.2013.11.015
Tarnocai, C., Canadell, J. G., Schuur, E. A. G., Kuhry, P., Mazhitova, G., and Zimov, S. (2009). Soil Organic Carbon Pools in the Northern Circumpolar Permafrost Region. Glob. Biogeochem. Cycles 23 (2), a–n. doi:10.1029/2008GB003327
Theriot, J. M., Conkle, J. L., Reza Pezeshki, S., DeLaune, R. D., and White, J. R. (2013). Will Hydrologic Restoration of Mississippi River Riparian Wetlands Improve Their Critical Biogeochemical Functions? Ecol. Eng. 60, 192–198. doi:10.1016/j.ecoleng.2013.07.021
Walker, D. A., Jia, G. J., Epstein, H. E., Raynolds, M. K., Chapin III, F. S., Copass, C., et al. (2003). Vegetation-soil-thaw-depth Relationships along a Low-Arctic Bioclimate Gradient, Alaska: Synthesis of Information from the ATLAS Studies. Permafrost Periglac. Process. 14 (2), 103–123. doi:10.1002/ppp.452
Wang, G., Hu, H., and Li, T. (2009). The Influence of Freeze-Thaw Cycles of Active Soil Layer on Surface Runoff in a Permafrost Watershed. J. Hydrol. 375 (3-4), 438–449. doi:10.1016/j.jhydrol.2009.06.046
Wang, J., Song, C., Hou, A., Miao, Y., Yang, G., and Zhang, J. (2014). Effects of Freezing-Thawing Cycle on Peatland Active Organic Carbon Fractions and Enzyme Activities in the Da Xing'anling Mountains, Northeast China. Environ. Earth Sci. 72 (6), 1853–1860. doi:10.1007/s12665-014-3094-z
Wang, J., Song, C., Wang, X., and Song, Y. (2012). Changes in Labile Soil Organic Carbon Fractions in Wetland Ecosystems along a Latitudinal Gradient in Northeast China. Catena 96, 83–89. doi:10.1016/j.catena.2012.03.009
Wei, Z., Jin, H., Zhang, J., Yu, S., Han, X., Ji, Y., et al. (2011). Prediction of Permafrost Changes in Northeastern China under a Changing Climate. Sci. China Earth Sci. 54 (6), 924–935. doi:10.1007/s11430-010-4109-6
Wisser, D., Marchenko, S., Talbot, J., Treat, C., and Frolking, S. (2011). Soil Temperature Response to 21st century Global Warming: the Role of and Some Implications for Peat Carbon in Thawing Permafrost Soils in North America. Earth Syst. Dynam. 2 (1), 121–138. doi:10.5194/esd-2-121-2011
Wu, X. D., Zhao, L., Fang, H. B., Chen, J., Pang, Q. Q., Wang, Z. W., et al. (2012). Soil Enzyme Activities in Permafrost Regions of the Western Qinghai-Tibetan Plateau. Soil Sci. Soc. America J. 76 (4), 1280–1289. doi:10.2136/sssaj2011.0400
Xu, H., Liu, G., Wu, X., Smoak, J. M., Mu, C., Ma, X., et al. (2018). Soil Enzyme Response to Permafrost Collapse in the Northern Qinghai-Tibetan Plateau. Ecol. Indicators 85 (15), 585–593. doi:10.1016/j.ecolind.2017.11.013
Xu, Z.-f., Hu, R., Xiong, P., Wan, C., Cao, G., and Liu, Q. (2010). Initial Soil Responses to Experimental Warming in Two Contrasting forest Ecosystems, Eastern Tibetan Plateau, China: Nutrient Availabilities, Microbial Properties and Enzyme Activities. Appl. Soil Ecol. 46 (2), 291–299. doi:10.1016/j.apsoil.2010.07.005
Xu, Z., Yu, G., Zhang, X., Ge, J., He, N., Wang, Q., et al. (2015). The Variations in Soil Microbial Communities, Enzyme Activities and Their Relationships with Soil Organic Matter Decomposition along the Northern Slope of Changbai Mountain. Appl. Soil Ecol. 86, 19–29. doi:10.1016/j.apsoil.2014.09.015
Yamazaki, Y., Kubota, J., Ohata, T., Vuglinsky, V., and Mizuyama, T. (2006). Seasonal Changes in Runoff Characteristics on a Permafrost Watershed in the Southern Mountainous Region of Eastern Siberia. Hydrol. Process. 20 (3), 453–467. doi:10.1002/hyp.5914
Yang, M., Nelson, F. E., Shiklomanov, N. I., Guo, D., and Wan, G. (2010). Permafrost Degradation and its Environmental Effects on the Tibetan Plateau: A Review of Recent Research. Earth-Science Rev. 103 (1-2), 31–44. doi:10.1016/j.earscirev.2010.07.002
Yuan, L., Zhao, L., Li, R., Hu, G., Du, E., Qiao, Y., et al. (2020). Spatiotemporal Characteristics of Hydrothermal Processes of the Active Layer on the central and Northern Qinghai-Tibet Plateau. Sci. Total Environ. 712, 136392. doi:10.1016/j.scitotenv.2019.136392
Zhang, T., Wan, S., Kang, Y., and Feng, H. (2014). Urease Activity and its Relationships to Soil Physiochemical Properties in a Highly saline-sodic Soil. J. Soil Sci. Plant Nutr. 14 (2), 304–315. doi:10.4067/S0718-95162014005000025
Zhang, X., He, J., Zhang, J., Polyakov, I., Gerdes, R., Inoue, J., et al. (2013). Enhanced Poleward Moisture Transport and Amplified Northern High-Latitude Wetting Trend. Nat. Clim Change 3 (1), 47–51. doi:10.1038/NCLIMATE1631
Zhang, Y. L., Chen, L. J., Chen, X. H., Tan, M. L., Duan, Z. H., Wu, Z. J., et al. (2015). Response of Soil Enzyme Activity to Long-Term Restoration of Desertified Land. Catena 133, 64–70. doi:10.1016/j.catena.2015.04.012
Zhang, Z.-Q., Wu, Q.-B., Hou, M.-T., Tai, B.-W., and An, Y.-K. (2021). Permafrost Change in Northeast China in the 1950s-2010s. Adv. Clim. Change Res. 12 (1), 18–28. doi:10.1016/j.accre.2021.01.006
Zhao, L. P., and Jiang, Y. (1986). Measure Method of Soil Phosphatase. Chin. J. Soil Sci. 17 (3), 138–141. (in Chinese).
Keywords: permafrost degradation, permafrost region, peatlands, soil enzyme activity, physicochemical properties, soil substrate, soil biogeochemical cycle
Citation: Liu C, Song Y, Dong X, Wang X, Ma X, Zhao G and Zang S (2021) Soil Enzyme Activities and Their Relationships With Soil C, N, and P in Peatlands From Different Types of Permafrost Regions, Northeast China. Front. Environ. Sci. 9:670769. doi: 10.3389/fenvs.2021.670769
Received: 22 February 2021; Accepted: 20 April 2021;
Published: 20 May 2021.
Edited by:
Lu-Jun Li, Northeast Institute of Geography and Agroecology (CAS), ChinaReviewed by:
Shengyun Chen, Northwest Institute of Eco-Environment and Resources (CAS), ChinaWei Shan, Northeast Forestry University, China
Copyright © 2021 Liu, Song, Dong, Wang, Ma, Zhao and Zang. This is an open-access article distributed under the terms of the Creative Commons Attribution License (CC BY). The use, distribution or reproduction in other forums is permitted, provided the original author(s) and the copyright owner(s) are credited and that the original publication in this journal is cited, in accordance with accepted academic practice. No use, distribution or reproduction is permitted which does not comply with these terms.
*Correspondence: Yanyu Song, songyanyu@iga.ac.cn; Shuying Zang, zsy6311@hrbnu.edu.cn