Carbohydrate compositional trends throughout Holocene sediments of an alpine lake (Lake Cadagno)
- 1Institute of Biogeochemistry and Pollutant Dynamics, Swiss Federal Institute of Technology (ETH Zurich), Zurich, Switzerland
- 2Institute of Earth Surface Dynamics (IDYST), University of Lausanne, Lausanne, Switzerland
- 3Institute of Geological Sciences and Oeschger Centre for Climate Change Research, University of Bern, Bern, Switzerland
- 4School of Oceanography, Shanghai Jiao Tong University, Shanghai, China
- 5Geological Institute, Department of Earth Sciences, ETH Zurich, Zurich, Switzerland
- 6Department of Surface Waters–Research and Management, Swiss Federal Institute of Aquatic Science and Technology (EAWAG), Dübendorf, Switzerland
- 7Department of Surface Waters–Research and Management, Swiss Federal Institute of Aquatic Science and Technology (EAWAG), Kastanienbaum, Switzerland
- 8Marine Science Institute, University of Texas at Austin, Port Aransas, TX, United States
Carbohydrates are a ubiquitous constituent of organisms and contribute significantly to sedimentary organic carbon pools. Yet, the factors that control the degradation and long-term preservation of sedimentary carbohydrates are not well understood. Here, we investigate carbohydrate pool sizes and chemical compositions in high-altitude, meromictic Lake Cadagno (Switzerland) over a 13,500-year-old sedimentary succession that has recorded past changes from oxic to anoxic conditions and consists mostly of intercalations of lacustrine sediments and terrestrial-derived sediments. Analyses of the organic matter chemical composition by pyrolysis gas chromatography/mass spectrometry (Py-GC/MS) show that carbohydrates are selectively preserved over other organic matter constituents over time. The carbohydrate pyrolysis products levosugars (potentially cellulose-derived) and (alkyl)furans and furanones (potentially pectin-derived) dominate both lacustrine and terrestrially derived sediment layers, suggesting aquatic and terrestrial-derived sources of these compounds. Carbohydrate monomer analyses indicate galactose and glucose as dominant monomers and show no clear differences between aquatic and terrestrial organic matter. No clear impacts of past changes in redox conditions on carbohydrate compositions were observed. Our study shows that carbohydrates are a major contributor to sedimentary organic carbon burial in Lake Cadagno and indicates the effective preservation of both aquatic and terrestrial derived carbohydrates over millennia in lake sediments.
Introduction
Sub-aqueous sediments are Earth’s biggest organic carbon (OC) sink over geologic timescales (Hedges and Keil, 1995). Previous studies suggest that carbohydrates contribute 1%–22% of total OC in sediments (Burdige, 2007; Arnosti et al., 2021), with the highest percentages in surface sediments with high rates of OC sedimentation (Hamilton and Hedges, 1988; Wakeham et al., 1997; Jensen et al., 2005). These percentages are likely to be underestimations, given that a significant fraction of sedimentary OC is molecularly uncharacterizable (Hedges et al., 2000; Burdige, 2007). Contributions of carbohydrates to total OC either decrease with sediment depth, indicating selective degradation over bulk OC (Hamilton and Hedges, 1988; Wakeham et al., 1997; Wang et al., 1998) or show no depth-related trends (Miyajima et al., 2001; Kerhervé et al., 2002; Jensen et al., 2005), including preservation for >100,000 years (Wang et al., 1998; Burdige, 2007; Quijada et al., 2015).
The preservation of sedimentary OC, including carbohydrates, is controlled by many factors. One important factor is oxygen (O2) exposure time (Hartnett et al., 1998; Zonneveld et al., 2010). This is because O2 is the highest energy-yielding biological electron acceptor (Canfield and Thamdrup, 2009) and in many cases supports high OC degradation rates (Middelburg et al., 1993). In addition, the chemical reactivity of OC strongly influences OC degradation rates. As reactive OC fractions are selectively removed with increasing time and sediment age, contributions of structural compounds and fragments of cell walls, which are more resistant to enzymatic hydrolysis, increase and lead to strong decrease in OC degradation rates (De Leeuw and Largeau, 1993; Hernes et al., 1996; Zhu et al., 2020). OC resistance to degradation can also increase as a result of abiotic complexation of diverse organic and inorganic components as “macromolecular matrices” (Keil and Mayer, 2014; LaRowe et al., 2020). In addition, physical shielding from enzyme attack by intact, degradation-resistant cell walls (Han et al., 2022) or through sorption and chemical bonds with minerals can enhance OC preservation (Keil and Mayer, 2014; Hemingway et al., 2019).
Carbohydrates are synthesized by organisms to store energy and provide structural support (Kennedy, 1988; Killops and Killops, 2013). Examples of energy storage carbohydrates are starch and glycogen (De Leeuw and Largeau, 1993), whereas structural carbohydrates include cellulose and chitin, the most abundant biopolymers in the terrestrial and aquatic realm, respectively (Gooday, 1990; Gupta, 2011; Wahlström et al., 2020). Owing to their function as intermediary energy reservoirs, energy storage carbohydrates are highly chemically reactive, whereas structural carbohydrates, which evolved to resist enzymatic attack, tend to be less chemically reactive. Nonetheless, knowledge on the controls on sedimentary carbohydrate cycling is still limited (Burdige, 2007). Experimental incubations with chemically pure carbohydrate monomers (Veuger et al., 2012), polymers (Boyer, 1994; Kanzog and Ramette, 2009), or polymer analogs (Wörner and Pester, 2019) indicate degradation of all carbohydrates, including structural polymers, over time scales of days to weeks, independent of redox conditions. These experimental results contrast with research indicating that, at least in certain marine sediments, carbohydrate contents and monomer compositions are nearly invariant over thousands of years (Miyajima et al., 2001; Quijada et al., 2015; Arnosti et al., 2021). A possible explanation is that chemically pure polymers used in experiments are more reactive than chemically complexed or physically shielded carbohydrates in marine sediments. Compared to marine sediments, even fewer studies have investigated the fate of carbohydrates in lake sediments (Handa and Mizuno, 1973; Ogier et al., 2001; Hayakawa, 2004) and to our knowledge only one study has investigated carbohydrate contents and compositions below the upper meter (Klok et al., 1984). Moreover, comparisons across studies are difficult due to the variety of carbohydrate extraction and quantification protocols (Zhu et al., 2020).
The chemical compositions of sedimentary carbohydrates can be characterized through analyses of elemental bonds and molecular structures (e.g., NMR, FT-ICR, pyrolysis) and by quantitative analyses of carbohydrate mono- and oligomer content (e.g., liquid- and gas chromatographic-based methods) (Bianchi and Canuel, 2011; Arnosti et al., 2021). Aqueous extractions involving chemical hydrolysis have been used to quantify total carbohydrate contents based on monosaccharide yields (Zhu et al., 2020) and to determine dominant carbohydrate sources based on monomer compositions and ratios of monomers (Benner and Kaiser, 2003; Carstens et al., 2012). In addition, pyrolysis-gas chromatography/mass spectrometry (Py-GC/MS) approaches have been used. Besides being a high-throughput method, Py-GC/MS has the benefit of providing insights into the contributions of all organic compound classes present in a sample (Tolu et al., 2017; 2015; Han et al., 2022), including chemically resistant compound classes that resist harsh chemical extraction methods (Zhu et al., 2020). In addition, Py-GC/MS provides information on the macromolecular structure of organic source compounds, e.g., polymer type, that is lost during the chemical hydrolysis to monomers (Dauwe and Middelburg, 1998; Tolu et al., 2015). Yet, Py-GC/MS is limited in quantifying OC compounds because in some cases the interpretation of pyrolysis products can be ambiguous, e.g., 2.5-diketopiperazines can be formed during pyrolysis of both protein and carbohydrates (Stankiewicz et al., 1998). Due to the different strengths the complementarity of information provided by each method, the combined analysis by chemical hydrolysis and Py-GC/MS is promising for the study of sedimentary carbohydrates.
Here we investigate the long-term controls on sedimentary carbohydrate preservation based on carbohydrate pool sizes and compositions across a 13,500-year-old continuous sedimentary succession (across the geological epoch—Holocene) in meromictic alpine Lake Cadagno (Wirth et al., 2013; Berg et al., 2022a). We hypothesize that past fluctuations in water column O2 concentrations, carbohydrate inputs, and carbohydrate sources have influenced sedimentary carbohydrate contents and composition through time. We integrate data on (1) water column redox history based on high-resolution scanning X-Ray-Fluorescence (XRF) sedimentary element data (Br, S, Mo, Fe, Mn, Al, Si, K, and Zr), (2) OC and carbohydrate macromolecule sources and compositions based on Py-GC/MS (Tolu et al., 2015) and (3) carbohydrate pool sizes and monomeric compositions extracted by sequential chemical hydrolysis followed by gas chromatographic analyses (Zhu et al., 2020). These data are synthesized with published organic carbon, nitrogen, and paleolimnological data (Camacho et al., 2001; Del Don et al., 2001; Wirth et al., 2013; Berg et al., 2022a) to better understand the drivers of sedimentary carbohydrate cycling throughout the history of Lake Cadagno. Our results show that—rather than monomer compositions or past water column redox conditions—the macromolecular structure and/or chemical matrices of the original OM sources in overlying water or surrounding land are the primary determinants of long-term carbohydrate preservation in sediments of Lake Cadagno.
Materials and methods
Study site
Lake Cadagno is a meromictic alpine lake located at an altitude of 1921 m above sea level in Ticino, southern Switzerland (Figure 1). The lake has a maximal water depth of 21 m, a surface area of 0.26 km2, and a small catchment area of only ∼2.4 km2 (Niemann et al., 2012). Today’s lake emerged following the last glacial period as indicated by uninterrupted lacustrine sedimentation since 13.5 kyr before the present (BP). Initially characterized by a well-oxygenated water column, Lake Cadagno has since evolved through a brief hypoxic stage with high dissolved manganese concentrations (“transition period”, ∼10.9–12.9 kyr BP) to the persisting meromictic sulfidic conditions (“euxinic period”, ∼10.9 kyr BP to today; Berg et al., 2022a; Wirth et al., 2013). Parallel to this evolution, Lake Cadagno sediments have changed from organic-poor, oxic glacial clays to organic-rich, anoxic sediments. The transition from fully mixed, oxygenated waters to stratified, permanently anoxic bottom waters was driven by the inflow of saline sulfidic subaquatic springs since the end of the last glacial stage (Del Don et al., 2001; Wirth et al., 2013). The presence of anoxic sulfidic conditions, together with anoxygenic photosynthesis, has made Lake Cadagno a famous analog for oceanic conditions on the early Earth (Dahl et al., 2010; Wirth et al., 2013; Xiong et al., 2019).
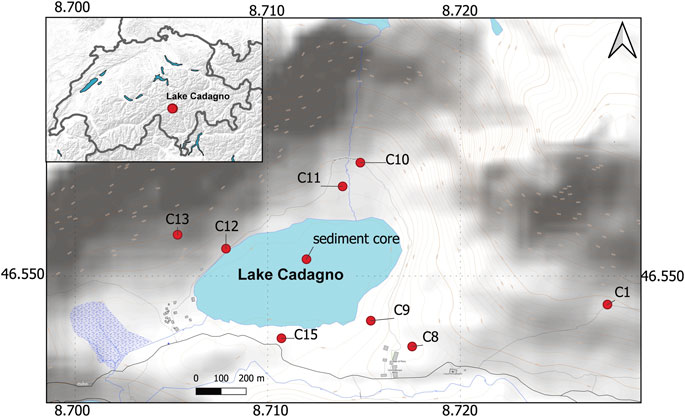
FIGURE 1. Map of Lake Cadagno study area in Southern Switzerland. The red dots indicate the location of lake sediments (sediment core) and soil (C1–C15) samples.
Besides OC produced by anoxygenic phototrophs in and below the chemocline (Tonolla et al., 2017; Saini et al., 2022) and by phyto- and zooplankton in the oxic epilimnion (Camacho et al., 2001), Lake Cadagno receives input of terrestrial vegetation and soil-derived OC from surrounding land. In addition, two types of density-driven events deposits (“mass movement deposits” and “flood deposits”) periodically transfer large volumes of sediments to deeper parts of the lake and can transport oxygen to the otherwise anoxic part of the lake (Wirth et al., 2013). Mass movement deposits, which can reach thicknesses of 70 cm, derive externally from steep slopes on the north side of the lake or consist of re-located sedimentary deposits from shallow parts of the lake. Mass movement deposits contain terrestrial sediments with varying contributions of lacustrine sediments and often have graded bedding, with coarse sands at the base and more silt and clay near the top of the layer. By contrast, flood deposits from snowmelt and avalanches, are thinner, are more uniformly dominated by sand and silt, and almost exclusively contain terrestrial OC (Niemann et al., 2012; Wirth et al., 2013). The frequency of these “event deposits” has varied through time and was lowest during the Holocene Thermal Maximum (∼9—5 kyr BP; Niemann et al., 2012; Figure 2). Flood deposits were most frequent between 5—3 kyr BP, while mass movements dominated between 8—5 kyr BP and within the last 2 kyr BP (Wirth et al., 2013).
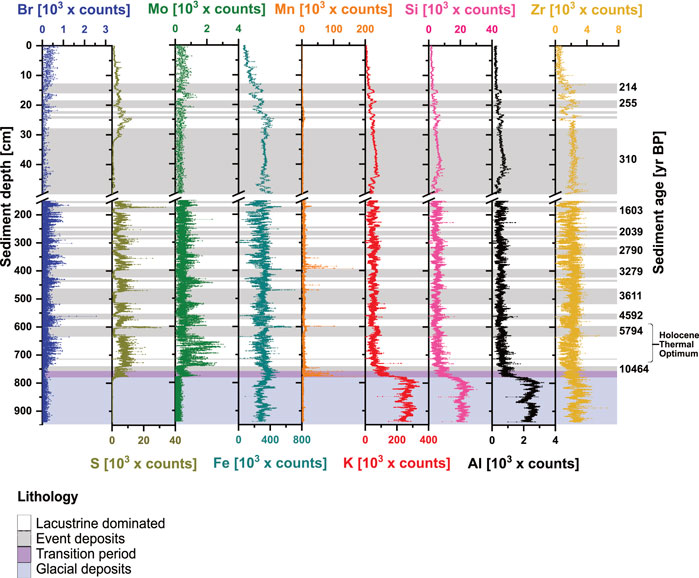
FIGURE 2. Trends in XRF-counts of nine major elements in relation to sediment depth [cm] and sediment age [yr BP].
Sediment and soil samples
Three overlapping up to 9-m-long piston cores from the deepest part of Lake Cadagno (Figure 1) were recovered in 2019 using a Uwitec-Niederreiter coring platform (HELVETIA, Uwitec, AT), as described in Berg et al. (2022a). The uppermost part of the succession, including the sediment-water interface, was recovered using a 90-mm diameter UWITEC gravity corer. One piston core succession was used for sedimentological observations, including XRF-scanning, while a second core was used for porewater sampling using Rhizons (0.2 μm pore size, Rhizosphere). A third piston core was used to collect sediment samples for measurements of solid phases. In addition, in 2020, 15 soil samples were collected from the lake surroundings (Figure 1), including a long water stream (C1, C8, and C9), from the shallower lake shore (C15), and from the steeper western (C12 and C13) and northern (C10 and C11) valley flanks. Samples for analysis of solid phases were kept frozen at −20°C until later analyses.
Age model
The age model is described in detail in Berg et al. (2022a) and its corrigendum (Berg et al., 2022b). In brief, the age model consists of 9 radiocarbon points of wood and macrofossils across an entire Lake Cadagno sediment core that were transferred from the study of Wirth et al. (2013). The age-depth modeling was conducted using Bayesian statistics (Blaauw and Christen, 2011). 14C calibrated ages were determined by the IntCal13 calibration curve (Reimer et al., 2013).
XRF element analysis
Vertical distributions of major and trace elements were determined at the University of Bern using the ITRAX μXRF core scanner equipped with a chromium X-ray tube (Cr-tube) set to 30 kV, 50 mA, 20 s integration time, and 0.5 mm resolution. For comparison with other data with a lower resolution, we applied an 11-point moving average to the XRF data. We focused on S and Mo as aquatic redox indicators (Wirth et al., 2013) and Br as a proxy for aquatic primary production (Cartapanis et al., 2011). Fe and Mn are redox-sensitive, with Fe (in combination with S) as an indicator for authigenic Fe-sulfide and bottom water anoxia and Mn as an indicator for the presence of Mn-oxides, which are only stable under oxic conditions during sediment deposition (Wirth et al., 2013). K, Si, Al, and Zr were used as indicators of terrestrial mineral input (Wirth et al., 2013), with Zr specifically serving as a proxy for coarse-grained igneous and metamorphic rock inputs owing to its almost exclusive presence in the abrasion-resistant and high-density mineral Zircon (density: 4.6–4.7 g/cm3) (Clift et al., 2020).
Grain size analyses
Sedimentary grain size distributions reveal changes from lacustrine sediments to event deposits, as well as gradations within event deposits. Pea-sized sediment amounts were dispersed in 2—3 mL of sodium polyphosphate and shaken overnight before analysis. Grain size distributions were then measured using a LS 13 320, laser diffraction particle size analyzer with polarization intensity differential scattering (PIDS, Beckman Coulter, Indianapolis, Umited States), equipped with an aqueous liquid module. Each sample was ultra-sonicated prior to analysis and measured for roughly 10 min. The relative contribution of the sediment fraction refers to the total sediment particle volume per sample.
Bulk organic matter analyses
Total organic carbon (TOC), total nitrogen (TN), and stable isotopic compositions of both (δ13C-TOC and δ15N-TN) were analyzed in all soil samples and compared to sedimentary data from Berg et al. (2022a). Samples were decalcified with 6 M HCl, neutralized with sodium hydroxide (NaOH), lyophilized and analyzed using an element analyzer (Flash-EA 1112, ThermoFisher Scientific) coupled to an isotope ratio mass spectrometer (IRMS, Delta V, ThermoFisher Scientific). The isotopic ratios refer to N2 (air) for nitrogen and Vienna Pee Dee Belemnite (V-PDB) notation for carbon. The reference standards were IAEA-N1 (15N = +0.45), IAEA N3 (15N = +4.72), and IAEA-N2 (15N = +20.41) for nitrogen, and NBS22 (13C =-30.03) and IAEA CH-6 (13C =-10.46) for carbon.
Chemical composition of major organic molecules
We used pyrolysis-GC/MS to investigate how organic matter chemical compositions change with age in sediments of Lake Cadagno and to what extent these changes can be attributed to differences in chemical reactivities (degradation rates), macromolecular structures (e.g., polymer types), and sources (e.g., lacustrine vs. terrestrial). Freeze-dried sediments (100—500 µg) were weighed into autosampler containers (Eco-cup SF, Frontier Laboratories, Japan) and pyrolyzed at 450°C and 650°C, according to Tolu et al. (2015). While 450°C delivered a diverse range of products suitable for further analysis, 650°C generated far fewer identifiable pyrolysis products that were not further analyzed. In addition, we analyzed nine carbohydrate standards (20–50 µg), including cellulose (microcrystalline, powder 20 µm), starch (from potato), pectin (SLCL6291), chitin (from shrimp shells), laminarin (from Laminaria digitata), alginic acid (from Macrocystis pyrifera), peptidoglycan (from Bacilius subtilis, all from Sigma Aldrich), mannan (from ivory nut), and arabinoxylan (both from Carbosynth Ltd., and were used as proxies for hemicellulose) at 450°C.
The pyrolyzer was connected to an autosampler (PY-2020iD and AS-1020E, FrontierLabs, Japan) and to a GC/MS system (Trace 1310, Thermo Scientific and ISQ 7000, Thermo Scientific) equipped with a DB-5MS capillary column (30 m x 0.25 mm i. d., 0.25 mm film thickness; J&W, Agilent Technologies AB, Sweden). Chromatograms were analyzed using R (version 2.15.2, 64 bits; http://www.R-project.org) based on a pipeline that identifies common mass fragments (Tolu et al., 2015). The mass spectra library software ‘NIST MS Search 2.0’ was used to determine pyrolysis products from mass fragment patterns, as described in Tolu et al. (2015). Pyrolysis products were then classified and annotated to OM constituents according to Tolu et al. (2015, 2017). In each sample, contributions of individual pyrolysis compound groups to total OC were expressed in percent of compound group peak area relative to total characterizable Py-GC/MS peak area ((total peak area of compound group/total characterizable Py-GC/MS peak area) × 100), as done previously (Tolu et al., 2015). The total characterizable Py-GC/MS peak area correlated positively with TOC across the analyzed sediments (R = 0.67; Supplementary Information (SI) - Supplementary Figure S8). Py-GC/MS data was produced to a depth of 727 cm, below which measurements were not possible, presumably due to the low TOC content (<1%).
Total hydrolyzable carbohydrates
We analyzed total hydrolyzable carbohydrates (THCHO) to determine how total carbohydrate contents and monomer compositions change over time in Lake Cadagno sediments. Freeze-dried samples were analyzed in duplicates (0.001 g–0.5 g) using the sequential extraction method of Zhu et al. (2020). The method involves a four-step extraction protocol with increasing HCl strength and temperature treatment: Step I (1 M HCl at room temperature for 2 h), Step II (1 M HCl at 50°C for 2 h), Step III (1 M HCl, at 105°C for 2 h), and Step IV (6 M HCl at 105°C for 8 h). This approach recovers, on average, ∼60% higher carbohydrate yields than one-step protocols and includes the separation of labile and more chemically resistant carbohydrates based on acid strength and temperature strength (Zhu et al., 2020). Fractions from each extraction step were individually, neutralized, purified, derivatized to aldononitrile acetate (Guerrant and Moss, 1984), and evaporated with a continuous N2 stream. Subsequently, all extracts were redissolved in 1 mL of ethyl acetate.1 µl of the extract was then injected into a gas chromatograph with a flame-ionization detector (GC-FID; Trace GC Ultra, Thermo-Finnigan equipped with a MEGA-5 MS Plus column - 60m x 0.25mm x 0.25um), using a modification of the protocol of Zhu et al. (2020). The program starts with an injector temperature of 250°C and an oven temperature of 70°C. The initial oven temperature is kept for 1 min, increased to 180°C at 4°C/min, held for 2 min, raised to 230°C at 4°C/min, kept for 15 min, and increased to 300°C at a gradient of 20°C/min and finally held for 1 min. The slower temperature gradient between 70°C and 180°C (4°C/min) compared to Zhu et al. (2020; 20°C/min) enabled a better separation between fucose and xylose.
Data visualization and statistical analyses
We determined the statistical relationship between lithological categories and organic and carbohydrate macromolecules with a non-parametric rank-based Kruskal-Wallis test. This followed by a Dunn’s Bonferroni test to compare the statistic significancy across the lithological classes of soil, surface sediment, subsurface event deposits and subsurface event deposits. All tests were performed in R. We also investigated relationships between organic macromolecules and carbohydrate compositions and other environmental variables, such as sediment age, bulk organic matter properties element distributions, and grain size data based on principal component analyses (PCA) and Spearman’s Rank Correlation Coefficient (ρ). PCA were calculated using the prcomp function, and visualized by autoplot using the ggplot2 package (Wickham, 2016). The Spearman correlation coefficients were calculated using the heatmap function and visualized using the heatmap.2 within the gplots package (Warnes et al., 2016).
Results
Sedimentation and redox history of Lake Cadagno based on XRF counts
XRF-based element distributions reflect how sediment sources and redox regimes have changed throughout the past 13.5 kyr. We differentiate between sediment layers that derive from lacustrine sedimentation and events deposits (flood and mass movement deposits) of the euxinic period (<10.9 kyr BP), the transition period (∼10.9–12.9 kyr BP) and glacial deposits (>12.9 kyr BP). The aquatic proxies, Br, S, and Mo, show higher elemental counts in euxinic lacustrine layers compared to event deposits and sediments deposited before euxinic times (Figure 2). The difference is most striking for S, which, moreover, in several cases, shows distinct peaks (5794 and 1603 yr BP) between lacustrine layers and event deposits. In glacial sediments, Br, S, and Mo show low elemental counts. S and Mo, but not Br, strongly increase during the transition from oxic to euxinic conditions.
The redox proxies Fe and Mn differ greatly in abundance (counts, cts) through time. Distinct peaks (Fe: >400,000 cts; Mn: >50,000 cts) at transitions from thick event deposits to lacustrine sediments are consistent with the formation of Fe-sulfides after mass movement-related oxidation events (Wirth et al., 2013; Berg et al., 2022a). Fe generally has lower counts in lacustrine-dominated layers than in event deposits and shows a clear decrease toward the sediment surface in the upper 20 cm. The highest Mn counts are found in sediments from the transition period (10.9–12.9 kyr BP), consistent with past research (Wirth et al., 2013; Berg et al., 2022a) and following specific events (5.8 and 3.3 kyr BP, Figure 2).
Lithogenic elements K, Si, Al, and Zr, are indicators of detrital clastic input and remain essentially unaltered by redox processes. These elements show the highest relative abundances in sediments deposited under oxic conditions (>12.5 yr BP) and decrease throughout the transition period and the onset of euxinic conditions (12.5 kyr BP; Figure 2), after which counts remain low (Figure 2A). Minor fluctuations occur at transitions between event deposit and lacustrine layers. All four elements decrease further toward the surface in the upper 40 cm as a result of increasing sediment pore water content.
Grain size distributions, bulk organic matter signatures, and carbohydrate pool sizes in sediments and soils
Sediment grain size distributions indicate that silt is the dominant fraction and accounts for 35%–83% of the sediment particle volume (Figure 3A). The sand fraction varies between 2% and 65%, with the highest percentages in event deposits and the lowest percentages in surface lacustrine and glacial deposits. The clay fraction ranges from 2% to 12%, with the highest abundances in glacial sediments. Soils from the lake catchment consistently have low clay contents (<5%) but vary in silt and sand contents between the different stations and generally have higher sand and lower silt and clay contents than lake sediments.
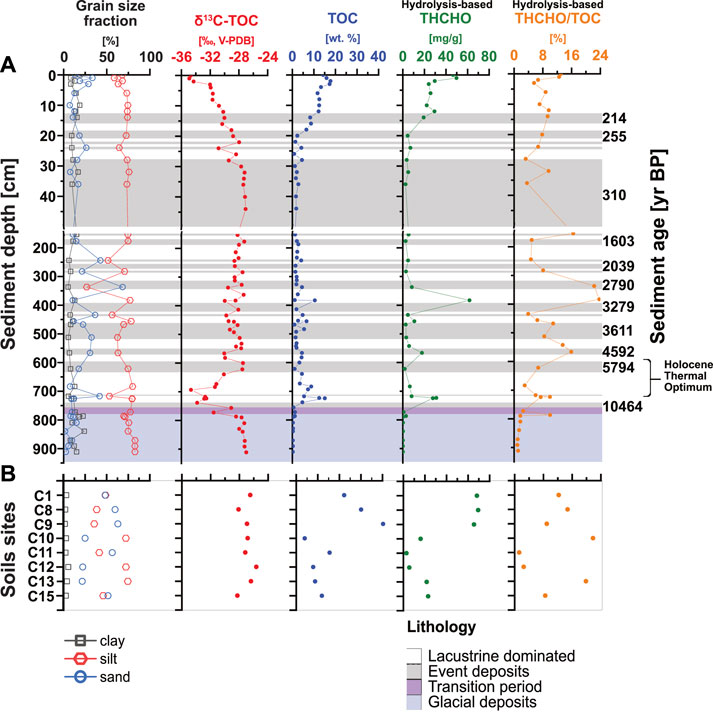
FIGURE 3. Profiles of grain size fractions, δ13C-TOC, TOC, THCHO, and THCHO/TOC versus sediment depth and age in lake sediments (Α) and soils (B).
TOC and δ13C-TOC (from Berg et al., 2022a) show opposite trends and suggest changes in OC inputs and sources throughout the Holocene (Figure 3A). TOC values are highest in soils (13% ± 9%; range 5%–40%; Figure 3B) and surface sediments (12% ± 5%), followed by subsurface lacustrine samples (8% ± 5%) and are clearly lower in subsurface event deposits (3% ± 2%) and glacial deposits (0.4% ± 0.3%; all Figure 3A). Sharp decreases in TOC occur between 20 and 30 cm and across the transition from deep euxinic to glacial sediments, whereas local increases to surface sedimentary values (∼15%, at 9 kyr BP) occur in sediments from the Holocene Thermal Optimum. δ13C-TOC is lowest in surface sediments (0–20 cm; −36‰; Figure 3A) but increases to −28‰ from 20 to 30 cm and remains between −30 and −28‰ in underlying euxinic and glacial sediments. The only exception is lacustrine-dominated layers from 9 to 5 kyr BP, where δ13C-TOC locally decreases to values as low as −36‰. In soils, δ13C-TOC is less negative than in lake sediments and varies between −28.5 and −25.5‰ (Figure 3B).
The THCHO distribution shows a depth trend similar to TOC (Figure 3A). Within the top 20 cm, THCHO decreases from 50 to 5 mg/g dry weight (wt.; Figure 3A) and remains below 10 mg/g dry wt. throughout the remainder of the core. Exceptions include samples with 60 mg/g dry wt. from ∼2.8 kyr BP and 30 mg/g dry wt. from ∼9 kyr BP, both from lacustrine-dominated layers. The lowest THCHO concentrations (<1 mg/g dry wt.) are found in glacial sediments (>11,500 yr BP). Contributions of THCHO to TOC (THCHO/TOC) drop from ∼15% in surface sediments to 5% at 30 cm. Throughout the remainder of the euxinic interval, values fluctuate from 4% to 24%, showing no clear differences between lacustrine-dominated layers and event deposits before dropping below 1% in deep glacial sediments. THCHO/TOC values in soils are in the same range and show similar variations as in sediments (1%–20%).
Compositions of macromolecular organic compounds in sediments and soils
Compositions of organic macromolecules differ clearly between Lake Cadagno sediments and catchment area soils and, in some cases, show clear depth-related trends in lake sediments (Figures 4A, B). All macromolecular organic compound groups except n-alkanes (C15-17-19) and n-alkenes (C16:1-18:1-20:1) show statistically significant variation across soils and sediment categories (surface sediments [0–20 cm], subsurface event deposits, subsurface lacustrine sediments [both below 20 cm]; note: crotonic acid was exclusively detected in soil and not part of this statistical comparison). Chlorophyll, sulfur compounds (S-compounds), and phenol values are always low in soils. Similarly, nitrogen-containing compounds (N-compounds), fatty acids, hopanoids, long-chain n-alkenes, and sterols are on average more abundant in lake sediments. In contrast, carbohydrates are always higher in soils, and short-chain n-alkenes, short- and long-chain n-alkanes, (poly)aromatic hydrocarbons (PAHs), and lignin do not show consistent differences between soils and sediments.
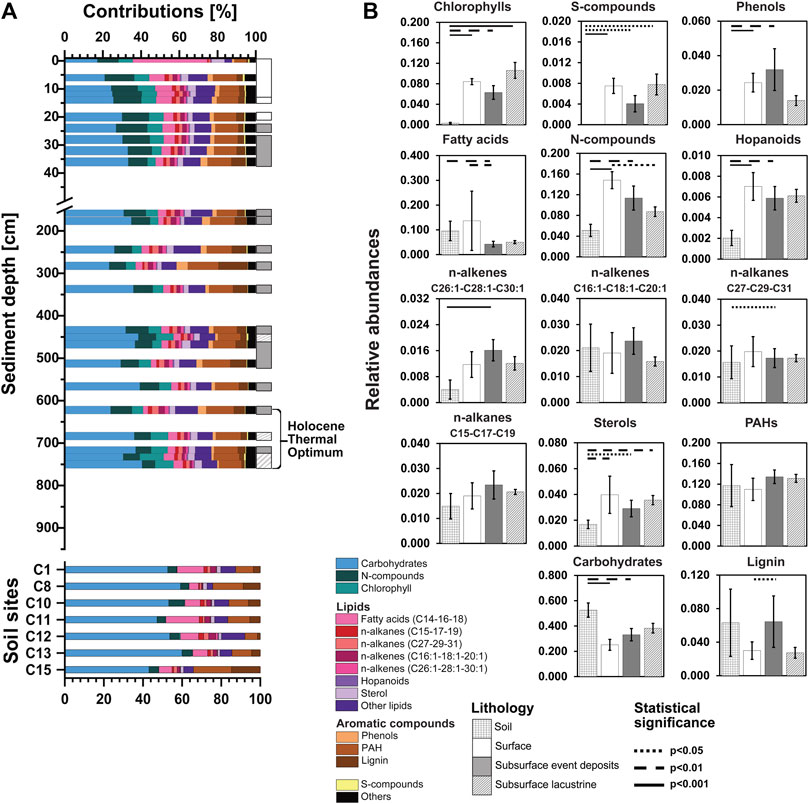
FIGURE 4. (A) Relative abundances of OM macromolecular compound classes are shown over sediment depth and sediment age for four different lithologies: soils as well as surface lacustrine sediments (<255 yr BP), event deposits, and deep lacustrine sediments (>255 yr BP). (B) Averaged relative abundance of each OM macromolecular compound class regarding the four lithologies. Kruskal-Wallis tests were performed with Dunn’s Bonferroni posthoc comparisons. Lithologies that differ significantly from each other in OM macromolecular content are connected by lines (solid: p<0.001; dashed: p<0.01; dotted: p<0.05). Note: The OC content of deep sediment samples of the transition and glacial period was below the detection limit of Py-GC/MS.
Carbohydrate contributions to are generally higher in soils (∼40–60% of total characterizable Py-GC/MS peak area; also see Materials and Methods) than in sediments (∼18–40%; both Figure 4), and moreover show clear depth-related trends in sediments. Within the top layer of sediments, carbohydrate contributions nearly double, from ∼16% at the sediment surface to ∼30% at 30 cm depth. Below 30 cm, the contribution of carbohydrates fluctuates between ∼20 and 40%, showing no systematic differences between lacustrine sediments and event deposits.
The observed increase in carbohydrate contents with sediment depth is accompanied by a decrease in the contributions of N-compounds from ∼10 to 20% in surface sediments to ∼5–10% in deeper layers. This is consistent with increases in C/N ratios reported previously (Berg et al., 2022a). In addition, fatty acids (C14-C16-C18) decrease with depth from ∼40% at the sediment surface to <5% below 6 cm. In soils, fatty acids represent around ∼5–15%, and N-compounds account for <5% of the total identified py-GC/MS peak area per sample. All other major compound groups are stable in the content below the sediment surface. Remarkably, chlorophyll, which is a suitable proxy for labile organic carbon in bioturbated surface sediments (e.g., Deng et al., 2020), has stable values of ∼5–10% throughout the entire sediment column of Lake Cadagno and is almost absent from soils. Furthermore, aromatic components, such as phenols, PAHs, and lignin together represent ∼20% of identifiable OM in most sediments and increase to ∼39% in the event at 282 cm and to ∼25% in events at 512 and 622 cm.
Carbohydrate macromolecule compositions in sediments and soils
Relative contributions of carbohydrates to TOC based on pyrolysis (based on peak areas) were higher than contributions of carbohydrates to TOC based on chemical extraction (based on C-content per g dry sediment; Table 1). This difference was the smallest for surface sediments and highest for subsurface sediments. Herein, the estimated contribution of carbohydrates based on chemical hydrolysis decrease from surface sediments to deeper sediments layers but shows the opposite trend based on pyrolysis data.

TABLE 1. Comparison of average carbohydrate contributions to TOC (%) across dominant lithologies based on Py-GC/MS (2nd column), chemical hydrolysis (3rd column), and ratios of both (4th column). [Note: carbohydrate contributions based on Py-GC/MS were calculated based on ratios of carbohydrate pyrolysis products to total characterizable carbohydrate pyrolysis products.].
Carbohydrate pyrolysis products differ between Lake Cadagno sediments and soils and change with depth and age in sediments (Figures 5A, B). Across the four lithological classes all major carbohydrate pyrolysis products except pyrans and crotonic acid vary significantly (Kruskal-Wallis test; for more information on the chemical structures of carbohydrate pyrolysis products, see SI—Supplementary Figure S9). While crotonic acid occurred exclusively in soils (Figure 5B), all other carbohydrate pyrolysis products were found throughout soil samples and lake sediments. A clear increase in average contribution with sediment depth was observed in levoglucosan (Figures 5A, B). Levoglucosan, which generally derive from glucose polymers (Schellekens et al., 2009; Tolu et al., 2015), collectively account for ∼30% of carbohydrates at the sediment surface, then decrease strongly, even going below detection at 6 and 12 cm before gradually increasing to ∼40% in the deepest sample (727 cm). In soils, Levoglucosan represent 20%—45% of the carbohydrate pyrolysis products and are the dominant carbohydrate fraction in most samples. The trends observed for levoglucosan also apply to quantitatively less important levoglucosenone.
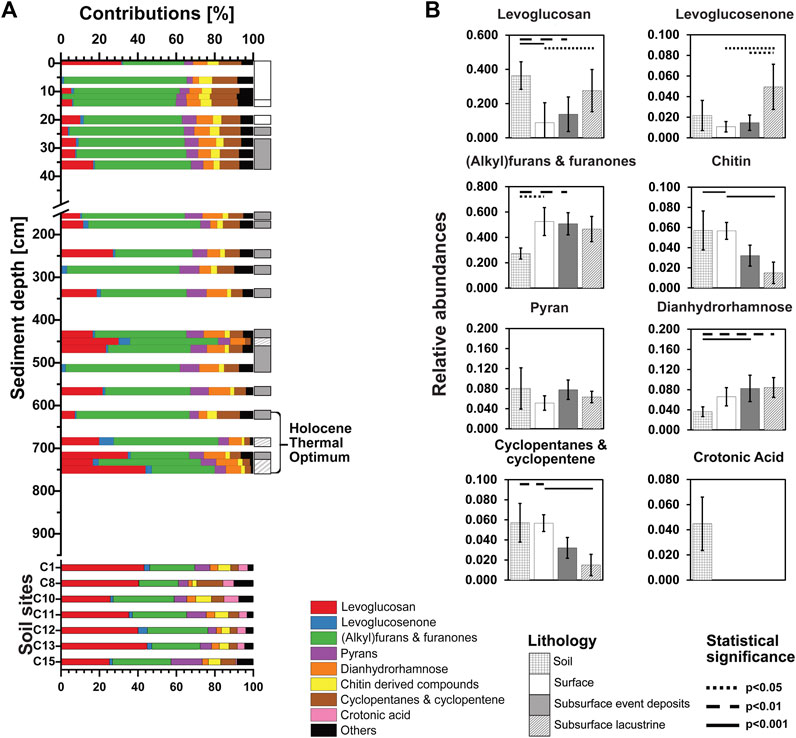
FIGURE 5. (A) The relative abundances of carbohydrate pyrolysis products are shown over depth and age for Lake Cadagno sediments at the top and for different soil sample sites surrounding the lake at the bottom. (B) Averaged relative abundance of each carbohydrate pyrolysis product regarding soils, surface lacustrine sediments (<255 yr BP), event deposits, and deep lacustrine sediments (>255 yr BP). Kruskal-Wallis tests were performed with Dunn’s Bonferroni posthoc comparisons. Lithologies that differ significantly from each other in OM macromolecular content are connected by lines (solid: p<0.001; dashed: p<0.01; dotted: p<0.05). Note: The OC content of deep sediment samples of the transition and glacial period was below the detection limit of Py-GC/MS.
(Alkyl)furans and furanones, which potentially derive from hemicellulose, pectins and/or alginic acids (Anastasakis et al., 2011; Patwardhan et al., 2011), are the dominant carbohydrate pyrolysis products in most lake sediment samples, increasing from ∼25% at the sediment surface to ∼60% at 5 cm sediment depth. Below 6 cm, (alkyl)furans and furanones remain the dominant carbohydrate pyrolysis product across lacustrine layers and event deposits, only being surpassed by levosugars in two deep samples. By contrast, (alkyl)furans and furanones only represent 15%–20% of soil carbohydrate pyrolysis products.
Pyrans and dianhydrorhamnose, which likely derive from lignocellulose (Kaal and Mailänder, 2019), chitin derived compounds and cyclopentanes and cyclopentenes, e.g., previously found in hemicelluloses and laminarin (Anastasakis et al., 2011; Patwardhan et al., 2011), account for on average from 5% to 10% of carbohydrate pyrolysis products across all sample types. While chitin derivatives and cyclopentanes and cyclopentenes decrease with sediment depth, pyrans or dianhydrorhamnose show no clear depth- or lithology-related trends.
Changes in carbohydrate monomeric composition
We found no clear compositional differences in carbohydrate monomers between lake sediments and soil samples. Galactose and glucose are the dominant monomers in sediments (average: ∼19± 5%) and soils (average: ∼20 ± 5%) (Figure 6A). Yet, certain event deposits (14 cm, 566 cm, 840 cm) have distinct monomer compositions, including elevated contributions of fucose. Moreover, the upper portion of glacial sediments contains higher relative contributions of the amino sugars glucosamine and galactosamine. In soil (Figure 6A), samples C1, C8, C9, and C15, along a stream (Figure 1) have similar monomeric compositions to lake sediments, whereas samples C10, C11, C12, and C13 from the northern slope side of Lake Cadagno contained an elevated percentage of amino sugars.
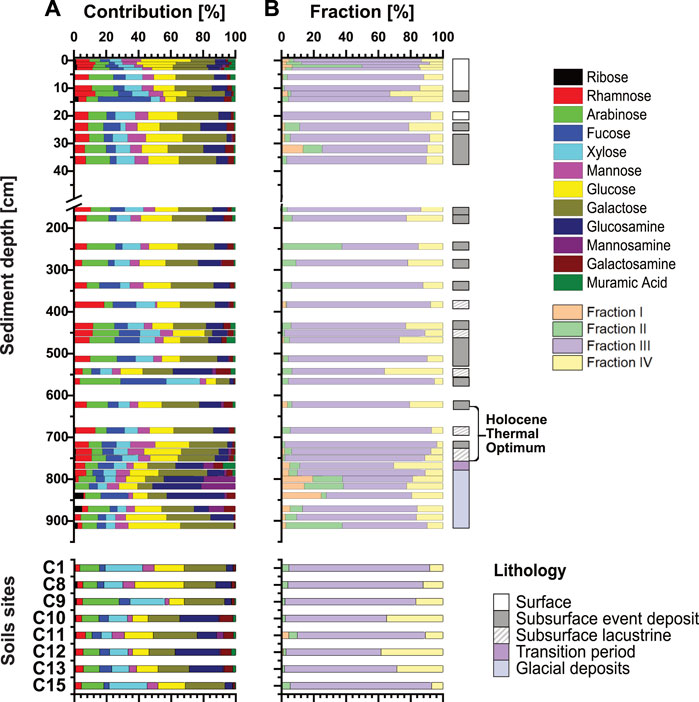
FIGURE 6. (A) Relative abundances of carbohydrate monomers from the total contribution of all extracted fractions. (B) Relative contributions of individual fractions for each sample. Lithologies refer to surface lacustrine sediments (<255 yr BP), event deposits, and deep lacustrine sediments (>255 yr BP). The bottom shows different Lake Cadagno soil sites.
Additional analyses on the four different carbohydrate hydrolysis fractions (Figure 6B) reveal that Fraction III makes up 60%–90% of the chemically extracted monomers. All samples also have significant contributions of Fraction IV with an average of 5%–25% in sediments and contributions of up to ∼40% in two soil samples (C10 and C12). In addition, surface sediments and Younger Dryas sediments contain elevated contributions of Fractions I and II. Hydrolysis fractions varied in monomer compositions, i.e., neutrals sugars were predominantly extracted at low temperatures and acid strengths (Fractions I-III), while amino sugars were primarily extracted in Fraction IV, which had the highest temperature and strongest acid treatment (Supplementary Figure S4).
Environmental clustering of organic macromolecules, carbohydrate macromolecules, and carbohydrate monomers
PCA on Py-GC/MS-based organic macromolecule and carbohydrate compositions confirms observations from Figures 4, 5 that OC molecular compositions differ systematically between soils and sediments and change with sediment age (Figures 7A, B). Similarly, as suggested by Figure 6, carbohydrate monomer compositions do not cluster in relation to lithology or sediment age (Figure 7C).
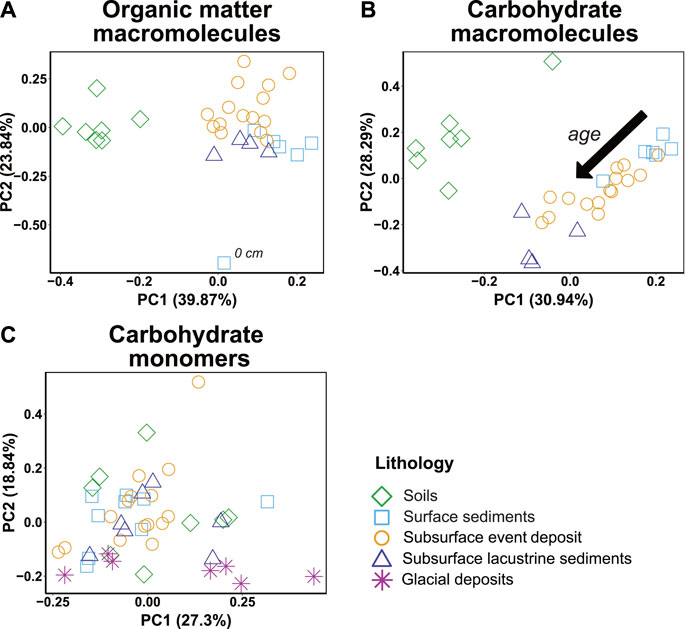
FIGURE 7. Principal component analyses of (A) all observed OM macromolecular compound classes in pyrolysis-gas chromatography/mass spectrometry (Py-GC/MS) (Figure 4) (B) all carbohydrate Py-GC/MS products (Figure 5) and (C) the total relative amounts of the 12 analyzed hydrolyzable carbohydrate monomers (Figure 6).
Discussion
We provide the first continuous lacustrine carbohydrate record from the entire Holocene epoch, to our knowledge, and show that carbohydrates account for a major portion of OC throughout sedimentary deposits from the past 13,500 years in meromictic alpine Lake Cadagno. Chemical hydrolysis data indicate carbohydrate contributions to TOC of 18% in surface sediments—percentages that are similar to those in other lake water column and surface sediment studies (Ogier et al., 2001; Zhu et al., 2020), and, on average, 7% in over 2000-year-old, deeper sediment layers (Table 1; Supplementary Table S1–S3). In contrast, While carbohydrate contents measured based on Py-GC/MS spectra are similar to those measured based on chemical hydrolysis at the sediment surface (17%), we observe an increase to carbohydrate contributions of 25%–38% in deeper layers based on Py-GC/MS (Table 1). The observed strong discrepancies between hydrolytic and pyrolytic methods in older sediments imply that even optimized chemical extraction methods with harsh final hydrolysis steps (6M HCl, 105°C) fail to extract a major fraction of carbohydrates in sediments that are thousands of years old (Table 1). This could be due to persistent physical protection (shielding) of residual carbohydrate pools, or chemical resistance of these residual pools, even to the harshest chemical hydrolysis conditions. Instead, the much higher temperature during pyrolysis (450°C) appears crucial in breaking resistant biological, chemical, and/or physical matrices and extracting residual carbohydrate pools. If the same principle applies to other settings, then chemical extraction methods can be expected to result in significant underestimations of carbohydrate pool sizes in older sediments.
Notably, carbohydrate macromolecule compositions show clear trends in relation to sediment age but overlap strongly between lacustrine and terrestrially dominated sediment layers and do not correlate with aquatic-terrestrial OM proxies, such as C/N ratios or δ13C-TOC (Supplementary Figure S2). This is different for well-established terrestrial biomarkers, such as phenols, PAHs, and lignin, which correlate positively with C/N and δ13C-TOC and are enriched in terrestrial layers (Supplementary Figure S5). A potential explanation is that the dominant carbohydrates in Lake Cadagno are of both aquatic and terrestrial origin.
Unlike previous environmental studies, in which carbohydrate monomer compositions were used as proxies for carbohydrate sources (Benner and Kaiser, 2003; Carstens et al., 2012), monomer compositions in Lake Cadagno do not differ significantly between soils, terrestrial-dominated sediments, and lacustrine sediments (Figure 7C). The only exception is deep glacial sediments, in which elevated contributions of muramic acid, a bacterial cell wall constituent (Moriarty, 1975; Carstens et al., 2012), indicate an elevated contribution of bacterial biomass. Similarly, past redox disruptions by event deposits, which are evident from depth profiles in S, Mo, Fe, and Mn (Figure 2), do not seem to have affected the compositions of carbohydrate macromolecules or monomers (Figures 5, 6; Supplementary Figure S6). In the following sections, we further analyze trends in OC and carbohydrate compositions and pool sizes in Lake Cadagno sediments, propose likely source molecules of dominant carbohydrate pyrolysis products, and discuss potential drivers of long-term carbohydrate preservation in the lake.
Lake Cadagno sediments as long-term OC and carbohydrate sinks
Surface sediments of Lake Cadagno have significantly higher TOC contents (18 wt%) compared to the average of other European lakes (∼2.8 ± 1.5 wt%) and relative to perialpine lakes at lower altitudes in Switzerland and bordering countries (∼3.1 ± 1.6 wt%; SI, Supplementary Table S2). Lakes with comparable TOC contents to Lake Cadagno include alpine lake Meidsee (TOC ∼12 wt%, Thevenon et al., 2012), perialpine lakes in the Tatra mountains (TOC ∼40 wt%, Gąsiorowski and Sienkiewicz, 2013), boreal lakes in Sweden (∼26.3 ± 5.0 wt%; Sobek et al., 2009) and arctic lakes in Greenland (e.g., SISI, AT; ∼9.8 ± 2.9 wt%; Anderson et al., 2018; Supplementary Table S2). The combination of seasonally high primary production, low temperature, short O2 exposure times due to shallow water depth, and permanent or seasonal bottom water anoxia, which excludes or greatly reduces macrofaunal bioturbation, may explain the high surface sedimentary OC contents in Lake Cadagno and these other lakes.
In contrast to surface OC contents, the preservation of OC over the last 12.5 kyr has so far only been investigated in a few lakes. During the Holocene Thermal Optimum, which ranged from roughly 9 to 5 kyr in Central Europe, specific accumulations of OC are found across different lakes. Lakes Cadagno contains TOC contents 15 wt% in sediments from ∼9.3 kyr BP and ∼8% on average between 9 and 5 kyr (Figure 3). Similar OC accumulations are observed in sediments of alpine Meidsee (∼20 wt%, 7.9 kyr; Thevenon et al., 2012; Supplementary Table S2) in arctic lakes in Greenland (on average 15 ± 5 wt%; 8—9 kyr BP; Anderson et al., 2018; Supplementary Table S2). In contrast, alpine Lake St. Moritz (∼6 wt%, 7 kyr; Ariztegui et al., 1996; Supplementary Table S2) and perialpine Lake Albano (∼7 wt%, 9 kyr; Ariztegui et al., 2001; Supplementary Table S2) have lower OC contents than Lake Cadagno between 9 and 5 kyr BP.
Carbohydrate contributions to TOC in Lake Cadagno sediments are in a similar range for perialpine Swiss lakes (e.g., Lake Lucerne: 21%—24% THCHO/TOC; 6–19 mg/g dry wt.; and Lake Greifen: 19%–27% THCHO/TOC; 8–13 mg/g dry wt.; Zhu et al., 2020). These contributions of >20%, which were all measured using the sequential extraction method of Zhu et al. (2020), exceed estimates obtained with one-step extraction protocols (Ogier et al., 2001; Zhu et al., 2020) and are only matched by carbohydrate contributions in coastal marine sediments after one-step extraction (Wang et al., 1998; Arnosti et al., 2021). To our knowledge, no data on higher-yielding carbohydrate measurements exist for sediments older than 2 kyr, aside from our study.
Shifts in OC pools through time provide insights into the reactivities of organic compound groups
The ∼80% decrease in TOC within the top 20 cm of sediment is mainly driven by high degradation rates of fatty acids (from 40% at the sediment surface down to 5% at 6 cm), as well as N-compounds (Figure 4). The concomitant steep increase in δ13C-TOC from - 35‰ at the sediment surface to - 29‰ at 20 cm indicates the selective depletion of carbon isotopically depleted bacterial biomass from below the chemocline in surface sediments. This bacterial biomass from below the chemocline has isotopic values of −42 to −31‰ (Posth et al., 2017) and largely consists of phototrophic purple and green sulfur bacterial OC (Putschew et al., 1995; Camacho et al., 2001; Saini et al., 2022). In addition, a significant fraction of OC from phytoplankton is mainly present above the chemocline where it has an average 13C isotopic composition of −25‰ (Posth et al., 2017). The debris of phytoplankton OC is degraded in surface sediments as evidenced by strong decreases in copy numbers of the gene encoding the large subunit of ribulose bisphosphate carboxylase (rbcL) of the two dominant phytoplankton groups in Lake Cadagno, Chlorophyta and Ochrophyta (Supplementary Figure S5; see Supplementary Text for DNA Methods).
The remaining TOC below 20 cm (mainly <5 wt%) is increasingly dominated by apparently more degradation-resistant carbohydrates (on average >30% of TOC; Figure 4) and aromatic compounds, such as PAHs, lignin, and phenol (Figure 4). Significant correlations of aromatic compounds with sand, Zr, and vascular plant (Tracheophyta) rbcL gene copy numbers (SI - Supplementary Figures S5, S6), and elevated contributions of aromatic compounds in specific, terrestrial-dominated event deposits (282, 512, and 612 cm; Figure 4), are consistent with mainly terrestrial sources. In contrast, carbohydrate sources are likely more diverse as no correlations were observed with δ13C-TOC or lithogenic elements, and only a weak correlation with Ochrophyta occurred (Supplementary Figure S6).
Cellulose and pectin as potential source molecules of dominant carbohydrates in Lake Cadagno sediments
(Alkyl)furans and furanones are the most abundant pyrolysis compound class among carbohydrates in sediments of Lake Cadagno (Figure 5). The absence of clear correlation patterns with terrestrial and lacustrine source indicators (Supplementary Figure S6) and the similar average contributions of (alkyl)furans and furanones across lacustrine sediments and event deposits (Figure 5) argues against a uniquely lacustrine or terrestrial provenance. Previously, hemicellulose was proposed as a source of (alkyl)furans and furanones in soils (Patwardhan et al., 2011), whereas alginic acids, fucoidan, and laminarin from brown algae (Phaeophyta), which are absent from freshwater habitats, were proposed as aquatic sources (Anastasakis et al., 2011). To shed additional light on the potential sources of pyrolysis products in Lake Cadagno, we performed Py-GC/MS measurements on a set of carbohydrates that are ubiquitous in nature (Figure 8). These tests reveal that pyrolysis of pectin also produces high amounts of (alkyl)furans and furanones, even higher amounts than the tested hemicelluloses arabinoxylan and mannan. Thus pectin, which is present as a structural carbohydrate in terrestrial plants, macroalgae, and certain microalgal Chlorophyta (Zanchetta et al., 2021), could be the main source of (alkyl)furans and furanones in Lake Cadagno sediments and soils. Microalgal Chlorophyta dominate microalgal communities above the chemocline (Camacho et al., 2001) and could thus represent a significant source of pectin to lacustrine OC-dominated sediment layers of Lake Cadagno. However, more research is needed to confirm this hypothesis.
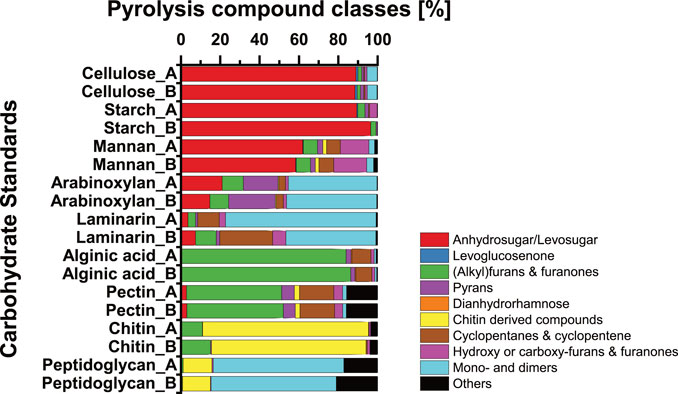
FIGURE 8. Relative abundances of carbohydrate pyrolysis products within nine different carbohydrate standards measured as replicates. Potential carbohydrate sources—cellulose: structural carbohydrate in vascular plants, aquatic algae, and bacteria (De Leeuw and Largeau, 1993; Patwardhan et al., 2009; Raghavendran et al., 2020; Zanchetta et al., 2021); starch: storage carbohydrates in vascular plants (De Leeuw and Largeau, 1993; Killops and Killops, 2013); mannan—structural carbohydrate in vascular plants and algae (De Leeuw and Largeau, 1993; Zanchetta et al., 2021); arabinoxylan—structural carbohydrate in vascular plants and algae (De Leeuw and Largeau, 1993); laminarin—storage carbohydrate in brown algae (Becker et al., 2020); alginic acid—structural carbohydrate in (brown) algae (Anastasakis et al., 2011); pectin—structural carbohydrate in vascular plants and green algae (De Leeuw and Largeau, 1993; Zanchetta et al., 2021); chitin—structural carbohydrate in arthropods, fungi, and algae (Gupta, 2011); peptidoglycan—structural carbohydrate in bacteria (Moriarty, 1975).
The levosugars represented mainly in levoglucosan and levoglucosenone are the second largest group of carbohydrate pyrolysis products in Lake Cadagno sediments and increase in carbohydrate contributions with sediment age independent of sediment origin. These pyrolysis products originate from a range of polyglucoses, including cellulose (Kuzhiyil et al., 2012; Kebelmann et al., 2013; Raghavendran et al., 2020; Zanchetta et al., 2021), glycan (Sanders et al., 2003), and starch (Figure 8). In addition, hemicelluloses are potential sources of levosugars (Zong et al., 2020), as supported by the hemicellulose standards mannan and arabinoxylan (Figure 8). While levosugars are produced naturally through the burning of biomass, e.g., forest fires (Schreuder et al., 2018), the uniformly high levosugar content throughout the sediment column and absence of observable ash layers suggest this is an unlikely main origin of levosugars in Lake Cadagno. Instead, it is more likely that these levosugars were produced during the pyrolysis of sedimentary polyglucose components. High contents of cellulose and/or hemicellulose from terrestrial vegetation could explain the high relative abundances of levosugars and levoglucosenone in watershed soils and terrestrial-dominated event deposits within the sediment column.
The high levosugar contents in lacustrine sediment layers are less straight forward to explain. In line with the other available data from this and previous studies, we propose a labile source of pyrolytic levosugar in surface sediments (Figure 5) that derives mainly from purple and green sulfur bacteria (Chromatium okenii and Chlorobium, respectively). These phototrophic bacteria account for most bacterial biomass in the lake (Camacho et al., 2001; Danza et al., 2017; Posth et al., 2017) and were previously shown to harbor large amounts of polyglucoses, such as glycogen, for energy storage (Del Don et al., 1994). The strong decrease in levosugar contribution in surface sediments could result from the rapid degradation of labile polyglucoses used for energy storage, both during starvation by phototrophic cells and as decaying phototrophic cells are being turned over by sedimentary organoheterotrophic microorganisms (Danza et al., 2017). The source of levosugars in deeper lacustrine OC-dominated sediment layers is even less clear. We propose that a significant fraction of these deep levosugars derive from degradation-resistant microalgal biomass. Chlorophyta and, to a lesser degree Ochrophyta, dominate microalgal communities above the chemocline in Lake Cadagno (Camacho et al., 2001). Both groups include cellulose- and hemicellulose-producing taxa (Zanchetta et al., 2021) and DNA of both groups was detected throughout the sediment column of Lake Cadagno (this study; SI - Supplementary Figure S5). Since structural carbohydrates from cell walls are generally more degradation resistant than energy storage carbohydrates (De Leeuw and Largeau, 1993; Wakeham et al., 1997), microalgal cellulose- and hemicellulose-containing cell walls could explain the high contributions of levoglucose and levoglucosenone in older lacustrine layers, including those deposited during the Holocene Thermal Optimum during which event deposits to the lake were minimal (Figure 5).
In addition, low percentages of pyrans and dianhydrorhamnose, chitin-derived compounds, and cyclopentane and cyclopentene were detected across all samples. Correlations of pyran and dianhydrorhamnose with lignin, Zr, and the size of the sedimentary sand fraction (SI - Supplementary Figure S6), suggest a predominantly terrestrial origin. This interpretation is consistent with previous research implicating lignocellulosic macromolecules from organic terrestrial debris as sources of these pyrolysis products (Kaal and Mailänder, 2019). At least for pyrans, this interpretation was supported by our standard tests, which indicated pyrans as major pyrolysis products of the hemicellulose arabinoxylan (Figure 8). By contrast, chitin-derived compounds and cyclopentane and cyclopentenes decrease significantly with sediment age, indicating selective degradation over other carbohydrate components (Figures 5B, 8). While the main organismal sources of chitin can presumably be narrowed down to fungi and zooplankton (Gooday, 1994; Gupta, 2011), the carbohydrate compound origins or organismal sources of cyclopentane and cyclopentenes remain enigmatic.
Potential drivers of long-term carbohydrate preservation in lake sediments
The efficient preservation of OC in sediments of Lake Cadagno could be related to the combination of short O2 exposure time and low temperatures (Sobek et al., 2009; Gudasz et al., 2010; Thevenon et al., 2012; Anderson et al., 2018). Previous research on boreal lakes has revealed a clear, positive relationship between temperature and OC mineralization rates (Gudasz et al., 2010). That said, even low-temperature sediments can have significant OC mineralization rates (Arnosti et al., 1998), and thus temperature alone may not be the main driver. Instead, short or no O2 exposure related to the meromixis and shallow water column of Lake Cadagno could result in minimal aerobic OC mineralization and be a key driver behind the high OC burial rates. Sinking OC that is produced above the chemocline or introduced to surface water from land is most likely rapidly exported to the underlying anoxic zone, and, moreover, a significant fraction of the OC within the lake is produced under permanently suboxic or anoxic conditions below the chemocline. The absence of O2 in bottom water and sediments could substantially slow microbial respiration rates. In addition, sedimentary macrofauna, which otherwise accelerate the breakdown of organic matter (Deng et al., 2020; Deng et al., 2022), are absent, and the prevailing sulfidic conditions could increase OC stability by OC sulfurization (Hebting et al., 2006).
In the absence of O2 and mechanical breakdown of OC matrices by macrofauna, physicochemical protection mechanisms could become more important. Mineral protection, e.g., due to chelation or ligand exchanges of organic compounds with clay minerals or inclusion of OC within enzymatically inaccessible clay matrices, could be an important factor in the long-term preservation of terrestrial (and lacustrine) organic matter (e.g., Keil and Mayer, 2014; Hemingway et al., 2019). Yet, in Lake Cadagno, recalcitrant carbohydrate pyrolysis products mostly correlate negatively with clay content (Supplementary Figure S6), suggesting that protection by clay minerals may not play a central role, at least not in the long-term preservation of carbohydrates. Instead, the long-term preservation of labile, intact (and therefore PCR-amplifiable) DNA of vascular plants, Chlorophyta, and Ochrophyta (Supplementary Figure S5) suggest that physical shielding of biomolecules by degradation-resistant cell wall structures could play an important role, similar to recent evidence from nearby perialpine lakes (Han et al., 2022). This would match the inferred dominance of the cell wall-derived structural carbohydrates cellulose and pectin in deep sediments of Lake Cadagno.
Further support for the important role of O2 exposure time and absence of macrofauna in driving carbohydrate preservation comes from perialpine Swiss lakes with deeper, oxygenated water columns and bioturbated surface sediments (Han, 2020). Surface sediments within these lakes, all of which have annually mixed water columns, invariably have >50% lower TOC-contributions of total hydrolysable carbohydrate contents than observed in meromictic Lake Cadagno in this study. If these differences are representative of a general trend, then one would expect meromictic lakes with oxygen-deficient bottom water to not only favor long-term OC burial, but to also disproportionately promote the long-term preservation of carbohydrates.
Summary
Our study indicates a major contribution of carbohydrates to total OC throughout the ∼13.5 kyr sediment sequence of Lake Cadagno—independent of lithology, age, and past redox conditions. High seasonal rates of primary production and (episodically) high terrestrial OC inputs combined with low water temperature, shallow water depth, and permanent bottom water anoxia are likely drivers of these high OC and carbohydrate contents. With increasing sediment age, microbial degradation-resistant carbohydrate macromolecule matrices that likely contain pectin and cellulose from aquatic and terrestrial phototrophs are selectively preserved over labile storage carbohydrates and chitinous components (Figure 5). Remarkably, despite these changes in dominant carbohydrate macromolecules with sediment age, no changes in carbohydrate monomer compositions were observed over time (Figure 6).
Our study underscores the fact that even optimized chemical hydrolysis methods fail to extract major portions of sedimentary OC compounds, especially for sediments that are thousands of years old and dominated by diagenetically altered and degradation-resistant organic matrices. In these sediments, average measured carbohydrate contributions to OC based on chemical hydrolysis were rather minor (<10%; Table 1), while Py-GC/MS measurements indicated carbohydrates as the dominant component of sedimentary OC (on average >30% of TOC; Table 1). The chemical mechanisms behind these yield differences are unclear. We propose that macromolecular matrices that include OC compounds and minerals with high resistance to the temperatures and acid strength of chemical hydrolysis prevent the release and extraction of most sedimentary carbohydrates by chemical hydrolysis. This challenge is overcome using the thermally harsher Py-GC/MS method, which may more effectively break these macromolecular matrices into their various components and thereby makes these detectable. By the same line of reasoning, the high temperatures used during pyrolysis may be necessary to break recalcitrant cell walls and release intracellular or macromolecular matrix-bound to make these measurable. In future studies, the chemical analysis of these structurally protected carbohydrates requires improvement to link monomeric contents to carbohydrate macromolecules across sediment age and to verify the sources of carbohydrate macromolecules such as pectin and (hemi)celluloses.
Data availability statement
The original contributions presented in the study are included in the article/Supplementary Files, further inquiries can be directed to the corresponding authors.
Author contributions
ML and NG designed the study. NG performed all organic matter compositional analyses. JB coordinated the sampling campaign and provided additional chemical data. HV contributed XRF data. NG and ND performed the grain size analyses. LD and SW contributed quantitative PCR data. JB and SB performed the isotopic analyses. CS assisted with the pyrolysis analyses and co-supervised the project. NG and ML wrote the manuscript. ML funded and supervised the project. All authors contributed to the article and approved the submitted version.
Funding
This project was funded by the ETH Zurich Research Grant “Controls on microbial chitin turnover in sediments” and the Swiss National Science Foundation project grant “Microbial cycling of sulfurized organic matter in freshwater sediments” (Grant No. 200021_182096; both to ML) Open access funding provided by ETH Zurich. Furthermore, Pyrolysis GC/MS analyses were funded by internal EAWAG funds to CS.
Acknowledgments
We acknowledge the Cadagno sampling teams of 2019 and 2020 for their field and sampling efforts and the Alpine Biology Center Foundation (Switzerland) for providing research facilities during sampling. Furthermore, we want to thank Julie Tolu and Serge Robert for their expertise and contributions to pyrolysis-GC/MS measurements and evaluation. Furthermore, we want to thank Irene Brunner and Madalina Jaggi for their support with chemical and sedimentological analyses.
Conflict of interest
The authors declare that the research was conducted in the absence of any commercial or financial relationships that could be construed as a potential conflict of interest.
Publisher’s note
All claims expressed in this article are solely those of the authors and do not necessarily represent those of their affiliated organizations, or those of the publisher, the editors and the reviewers. Any product that may be evaluated in this article, or claim that may be made by its manufacturer, is not guaranteed or endorsed by the publisher.
Supplementary material
The Supplementary Material for this article can be found online at: https://www.frontiersin.org/articles/10.3389/feart.2023.1047224/full#supplementary-material
References
Anastasakis, K., Ross, A. B., and Jones, J. M. (2011). Pyrolysis behaviour of the main carbohydrates of brown macro-algae. Fuel 90, 598–607. doi:10.1016/j.fuel.2010.09.023
Anderson, N. J., Leng, M. J., Osburn, C. L., Fritz, S. C., Law, A. C., and McGowan, S. (2018). A landscape perspective of Holocene organic carbon cycling in coastal SW Greenland lake-catchments. Quat. Sci. Rev. 202, 98–108. doi:10.1016/j.quascirev.2018.09.006
Ariztegui, D., Chondrogianni, C., Lami, A., Guilizzoni, P., and Lafargue, E. (2001). Lacustrine organic matter and the Holocene paleoenvironmental record of Lake Albano (central Italy). J. Paleolimnol. 26, 283–292. doi:10.1023/A:1017585808433
Ariztegui, D., Farrimond, P., and Mckenzie, J. A. (1996). Compositional variations in sedimentary lacustrine organic matter and their implications for high Alpine Holocene environmental changes: Lake St. Moritz, Switzerland., Int. Meet. Org. Geochem. 24, 453–461. doi:10.1016/0146-6380(96)00046-0
Arnosti, C., Jørgensen, B. B., Sagemann, J., and Thamdrup, B. (1998). Temperature dependence of microbial degradation of organic matter in marine sediments: Polysaccharide hydrolysis, oxygen consumption, and sulfate reduction. Mar. Ecol. Prog. Ser. 165, 59–70. doi:10.3354/meps165059
Arnosti, C., Wietz, M., Brinkhoff, T., Hehemann, J. H., Probandt, D., Zeugner, L., et al. (2021). The biogeochemistry of marine polysaccharides: Sources, inventories, and bacterial drivers of the carbohydrate cycle. Annu. Rev. Mar. Sci. 13, 81–108. doi:10.1146/annurev-marine-032020-012810
Becker, S., Tebben, J., Coffinet, S., Wiltshire, K., Iversen, M. H., Harder, T., et al. (2020). Laminarin is a major molecule in the marine carbon cycle. Proc. Natl. Acad. Sci. 117, 6599–6607. doi:10.1073/pnas.1917001117
Benner, R., and Kaiser, K. (2003). Abundance of amino sugars and peptidoglycan in marine particulate and dissolved organic matter. Limnol. Oceanogr. 48, 118–128. doi:10.4319/lo.2003.48.1.0118
Berg, J. S., Lepine, M., Laymand, E., Han, X., Vogel, H., Morlock, M. A., et al. (2022a). Ancient and modern geochemical signatures in the 13,500-year sedimentary record of Lake Cadagno. Front. Earth Sci. 9, 754888. doi:10.3389/feart.2021.754888
Berg, J. S., Lepine, M., Laymand, E., Han, X., Vogel, H., Morlock, M. A., et al. (2022b). Corrigendum: Ancient and modern geochemical signatures in the 13,500-year sedimentary record of Lake Cadagno. Front. Earth Sci. 10, 912255. doi:10.3389/feart.2022.912255
Bianchi, T. S., and Canuel, E. A. (2011). Chemical biomarkers in aquatic ecosystems. Princeton: Princeton University Press.
Blaauw, M., and Christen, J. A. (2011). Flexible paleoclimate age-depth models using an autoregressive gamma process. Bayesian Anal. 6, 457–474. doi:10.1214/11-ba618
Boyer, J. N. (1994). Aerobic and anaerobic degradation and mineralization of 14C-chitin by water column and sediment inocula of the York River estuary, Virginia. Appl. Env. Microbiol. 60, 174–179. doi:10.1128/aem.60.1.174-179.1994
Burdige, D. J. (2007). Preservation of organic matter in marine sediments: Controls, mechanisms, and an imbalance in sediment organic carbon budgets? Chem. Rev. 107, 467–485. doi:10.1021/cr050347q
Camacho, A., Erez, J., Chicote, A., Florín, M., Squires, M. M., Lehmann, C., et al. (2001). Microbial microstratification, inorganic carbon photoassimilation and dark carbon fixation at the chemocline of the meromictic Lake Cadagno (Switzerland) and its relevance to the food web. Aquat. Sci. 63, 91–106. doi:10.1007/PL00001346
Canfield, D. E., and Thamdrup, B. (2009). Towards a consistent classification scheme for geochemical environments, or, why we wish the term ‘suboxic’ would go away. Geobiology 7, 385–392. doi:10.1111/j.1472-4669.2009.00214.x
Carstens, D., Köllner, K. E., Bürgmann, H., Wehrli, B., and Schubert, C. J. (2012). Contribution of bacterial cells to lacustrine organic matter based on amino sugars and d-amino acids. Geochim. Cosmochim. Acta 89, 159–172. doi:10.1016/j.gca.2012.04.052
Cartapanis, O., Tachikawa, K., and Bard, E. (2011). Northeastern Pacific oxygen minimum zone variability over the past 70 kyr: Impact of biological production and oceanic ventilation. Paleoceanography 26, 2126. doi:10.1029/2011PA002126
Clift, P. D., Kulhanek, D. K., Zhou, P., Bowen, M. G., Vincent, S. M., Lyle, M., et al. (2020). Chemical weathering and erosion responses to changing monsoon climate in the Late Miocene of Southwest Asia. Geol. Mag. 157, 939–955. doi:10.1017/S0016756819000608
Dahl, T. W., Anbar, A. D., Gordon, G. W., Rosing, M. T., Frei, R., and Canfield, D. E. (2010). The behavior of molybdenum and its isotopes across the chemocline and in the sediments of sulfidic Lake Cadagno, Switzerland. Geochim. Cosmochim. Acta 74, 144–163. doi:10.1016/j.gca.2009.09.018
Danza, F., Storelli, N., Roman, S., Lüdin, S., and Tonolla, M. (2017). Dynamic cellular complexity of anoxygenic phototrophic sulfur bacteria in the chemocline of meromictic Lake Cadagno. PLOS ONE 12, e0189510. doi:10.1371/journal.pone.0189510
Dauwe, B., and Middelburg, J. J. (1998). Amino acids and hexosamines as indicators of organic matter degradation state in North Sea sediments. Limnol. Oceanogr. 43, 782–798. doi:10.4319/lo.1998.43.5.0782
De Leeuw, J. W., and Largeau, C. (1993). “A review of macromolecular organic compounds that comprise living organisms and their role in kerogen, coal, and petroleum formation,” in Organic geochemistry: Principles and applications, topics in geobiology. Editors M. H. Engel, and S. A. Macko (Boston, MA: Springer US), 23–72. doi:10.1007/978-1-4615-2890-6_2
Del Don, C., Hanselmann, K. W., Peduzzi, R., and Bachofen, R. (1994). Biomass composition and methods for the determination of metabolic reserve polymers in phototrophic sulfur bacteria. Aquat. Sci. 56, 1–15. doi:10.1007/BF00877431
Del Don, C., Hanselmann, K. W., Peduzzi, R., and Bachofen, R. (2001). The meromictic alpine Lake Cadagno: Orographical and biogeochemical description. Aquat. Sci. 63, 70–90. doi:10.1007/pl00001345
Deng, L., Bölsterli, D., Kristensen, E., Meile, C., Su, C. C., Bernasconi, S. M., et al. (2020). Macrofaunal control of microbial community structure in continental margin sediments. Proc. Natl. Acad. Sci. 117, 15911–15922. doi:10.1073/pnas.1917494117
Deng, L., Meile, C., Fiskal, A., Bölsterli, D., Han, X., Gajendra, N., et al. (2022). Deposit-feeding worms control subsurface ecosystem functioning in intertidal sediment with strong physical forcing. PNAS Nexus 1, pgac146. doi:10.1093/pnasnexus/pgac146
Gąsiorowski, M., and Sienkiewicz, E. (2013). The sources of carbon and nitrogen in mountain lakes and the role of human activity in their modification determined by tracking stable isotope composition. Air Soil Pollut. 224, 1498. doi:10.1007/s11270-013-1498-0
Gooday, G. W. (1994). “Physiology of microbial degradation of chitin and chitosan,” in Biochemistry of microbial degradation. Editor C. Ratledge (Dordrecht: Springer Netherlands), 279–312. doi:10.1007/978-94-011-1687-9_9
Gooday, G. W. (1990). “The ecology of chitin degradation,” in Advances in microbial ecology, advances in microbial ecology (Boston, MA: Springer), 387–430. doi:10.1007/978-1-4684-7612-5_10
Gudasz, C., Bastviken, D., Steger, K., Premke, K., Sobek, S., and Tranvik, L. J. (2010). Temperature-controlled organic carbon mineralization in lake sediments. Nature 466, 478–481. doi:10.1038/nature09186
Guerrant, G. O., and Moss, C. W. (1984). Determination of monosaccharides as aldononitrile, O-methyloxime, alditol, and cyclitol acetate derivatives by gas chromatography. Anal. Chem. 56, 633–638. doi:10.1021/ac00268a010
Gupta, N. S. (2011). “Chitin: Formation and diagenesis,” in Topics in geobiology (Netherlands: Springer). doi:10.1007/978-90-481-9684-5
Hamilton, S. E., and Hedges, J. I. (1988). The comparative geochemistries of lignins and carbohydrates in an anoxic fjord. Geochim. Cosmochim. Acta 52, 129–142. doi:10.1016/0016-7037(88)90062-2
Han, X. (2020). Influence of eutrophication on microbial community structure, organic carbon sources, and organic carbon degradation in lake sediments through time. Doctoral Thesis. Zurich: ETH Zurich. doi:10.3929/ethz-b-000488233
Han, X., Tolu, J., Deng, L., Fiskal, A., Schubert, C. J., Winkel, L. H. E., et al. (2022). Long-term preservation of biomolecules in lake sediments: Potential importance of physical shielding by recalcitrant cell walls. PNAS Nexus 1, pgac076. doi:10.1093/pnasnexus/pgac076
Handa, N., and Mizuno, K. (1973). Carbohydrates from lake sediments. Geochem. J. 7, 215–230. doi:10.2343/geochemj.7.215
Hartnett, H. E., Keil, R. G., Hedges, J. I., and Devol, A. H. (1998). Influence of oxygen exposure time on organic carbon preservation in continental margin sediments. Nature 391, 572–575. doi:10.1038/35351
Hayakawa, K. (2004). Seasonal variations and dynamics of dissolved carbohydrates in Lake Biwa. Biwa. Org. Geochem. 35, 169–179. doi:10.1016/j.orggeochem.2003.09.002
Hebting, Y., Schaeffer, P., Behrens, A., Adam, P., Schmitt, G., Schneckenburger, P., et al. (2006). Biomarker evidence for a major preservation pathway of sedimentary organic carbon. Science 312, 1627–1631. doi:10.1126/science.1126372
Hedges, J. I., Eglinton, G., Hatcher, P. G., Kirchman, D. L., Arnosti, C., Derenne, S., et al. (2000). The molecularly-uncharacterized component of nonliving organic matter in natural environments. Org. Geochem. 31, 945–958. doi:10.1016/S0146-6380(00)00096-6
Hedges, J. I., and Keil, R. G. (1995). Sedimentary organic matter preservation: An assessment and speculative synthesis. Mar. Chem. 49, 81–115. doi:10.1016/0304-4203(95)00008-F
Hemingway, J. D., Rothman, D. H., Grant, K. E., Rosengard, S. Z., Eglinton, T. I., Derry, L. A., et al. (2019). Mineral protection regulates long-term global preservation of natural organic carbon. Nature 570, 228–231. doi:10.1038/s41586-019-1280-6
Hernes, P. J., Hedges, J. I., Peterson, M. L., Wakeham, S. G., and Lee, C. (1996). Neutral carbohydrate geochemistry of particulate material in the central equatorial Pacific. Deep Sea Res. Part II Top. Stud. Oceanogr. 43, 1181–1204. doi:10.1016/0967-0645(96)00012-4
Jensen, M. M., Holmer, M., and Thamdrup, B. (2005). Composition and diagenesis of neutral carbohydrates in sediments of the Baltic-North Sea transition. Geochim. Cosmochim. Acta 69, 4085–4099. doi:10.1016/j.gca.2005.01.021
Kaal, J., and Mailänder, S. (2019). Molecular properties of soil organic matter in dark buried colluvium from South Germany show abundance of fire residues from Early Neolithic vegetation clearance and slash and burn agriculture. Anal. Pyrolysis Lett. 4, 1–10.
Kanzog, C., and Ramette, A. (2009). Microbial colonisation of artificial and deep-sea sediments in the Arctic Ocean. Mar. Ecol. 30, 391–404. doi:10.1111/j.1439-0485.2009.00290.x
Kebelmann, K., Hornung, A., Karsten, U., and Griffiths, G. (2013). Intermediate pyrolysis and product identification by TGA and Py-GC/MS of green microalgae and their extracted protein and lipid components. Biomass Bioenergy 49, 38–48. doi:10.1016/j.biombioe.2012.12.006
Kerhervé, P., Buscail, R., Gadel, F., and Serve, L. (2002). Neutral monosaccharides in surface sediments of the northwestern Mediterranean Sea. Org. Geochem. 33, 421–435. doi:10.1016/S0146-6380(02)00003-7
Killops, S. D., and Killops, V. J. (2013). Introduction to organic geochemistry. John Wiley and Sons.
Klok, J., Cox, H. C., Baas, M., de Leeuw, J. W., and Schenck, P. A. (1984). Carbohydrates in recent marine sediments—II. Occurrence and fate of carbohydrates in a recent stromatolitic deposit: Solar lake, sinai. Org. Geochem. 7, 101–109. doi:10.1016/0146-6380(84)90123-2
Kuzhiyil, N., Dalluge, D., Bai, X., Kim, K. H., and Brown, R. C. (2012). Pyrolytic sugars from cellulosic biomass. ChemSusChem 5, 2228–2236. doi:10.1002/cssc.201200341
LaRowe, D. E., Arndt, S., Bradley, J. A., Estes, E. R., Hoarfrost, A., Lang, S. Q., et al. (2020). The fate of organic carbon in marine sediments - new insights from recent data and analysis. Earth-Sci. Rev. 204, 103146. doi:10.1016/j.earscirev.2020.103146
Middelburg, J. J., Vlug, T., Jaco, F., and van der Nat, W. A. (1993). Organic matter mineralization in marine systems. Glob. Planet. Change 8, 47–58. doi:10.1016/0921-8181(93)90062-S
Miyajima, T., Ogawa, H., and Koike, I. (2001). Alkali-extractable polysaccharides in marine sediments: Abundance, molecular size distribution, and monosaccharide composition. Geochim. Cosmochim. Acta 65, 1455–1466. doi:10.1016/S0016-7037(00)00612-8
Moriarty, D. J. W. (1975). A method for estimating the biomass of bacteria in aquatic sediments and its application to trophic studies. Oecologia 20, 219–229. doi:10.1007/BF00347474
Niemann, H., Stadnitskaia, A., Wirth, S. B., Gilli, A., Anselmetti, F. S., Sinninghe Damsté, J. S., et al. (2012). Bacterial GDGTs in Holocene sediments and catchment soils of a high Alpine lake: Application of the MBT/CBT-paleothermometer. Clim. Past. 8, 889–906. doi:10.5194/cp-8-889-2012
Ogier, S., Disnar, J. R., Albéric, P., and Bourdier, G. (2001). Neutral carbohydrate geochemistry of particulate material (trap and core sediments) in an eutrophic lake (Aydat, France). Org. Geochem. 32, 151–162. doi:10.1016/S0146-6380(00)00138-8
Patwardhan, P. R., Brown, R. C., and Shanks, B. H. (2011). Product distribution from the fast pyrolysis of hemicellulose. ChemSusChem 4, 636–643. doi:10.1002/cssc.201000425
Patwardhan, P. R., Satrio, J. A., Brown, R. C., and Shanks, B. H. (2009). Product distribution from fast pyrolysis of glucose-based carbohydrates. J. Anal. Appl. Pyrolysis 86, 323–330. doi:10.1016/j.jaap.2009.08.007
Posth, N. R., Bristow, L. A., Cox, R. P., Habicht, K. S., Danza, F., Tonolla, M., et al. (2017). Carbon isotope fractionation by anoxygenic phototrophic bacteria in euxinic Lake Cadagno. Geobiology 15, 798–816. doi:10.1111/gbi.12254
Putschew, A., Scholz-Böttcher, B. M., and Rullkötter, J. (1995). “Organic geochemistry of sulfur-rich surface sediments of meromictic Lake Cadagno, Swiss alps,” in Geochemical transformations of sedimentary sulfur (Washington, DC: ACS Symposium Series American Chemical Society), 59–79. doi:10.1021/bk-1995-0612.ch004
Quijada, M., Riboulleau, A., Guerardel, Y., Monnet, C., and Tribovillard, N. (2015). Neutral aldoses derived from sequential acid hydrolysis of sediments as indicators of diagenesis over 120,000years. Org. Geochem. 81, 53–63. doi:10.1016/j.orggeochem.2015.01.011
Raghavendran, V., Asare, E., and Roy, I. (2020). “Chapter three - bacterial cellulose: Biosynthesis, production, and applications,” in Advances in microbial physiology. Editor R. K. Poole (Academic Press), 89–138. doi:10.1016/bs.ampbs.2020.07.002
Reimer, P. J., Bard, E., Bayliss, A., Beck, J. W., Blackwell, P. G., Ramsey, C. B., et al. (2013). IntCal13 and Marine13 radiocarbon age calibration curves 0–50,000 Years cal BP. Radiocarbon 55, 1869–1887. doi:10.2458/azu_js_rc.55.16947
Saini, J. S., Hassler, C., Cable, R., Fourquez, M., Danza, F., Roman, S., et al. (2022). Bacterial, phytoplankton, and viral distributions and their biogeochemical contexts in meromictic Lake Cadagno offer insights into the proterozoic ocean microbial loop. mBio 0, e0005222–22. doi:10.1128/mbio.00052-22
Sanders, E. B., Goldsmith, A. I., and Seeman, J. I. (2003). A model that distinguishes the pyrolysis of d-glucose, d-fructose, and sucrose from that of cellulose. Application to the understanding of cigarette smoke formation. J. Anal. Appl. Pyrolysis 66, 29–50. doi:10.1016/S0165-2370(02)00104-3
Schellekens, J., Buurman, P., and Pontevedra-Pombal, X. (2009). Selecting parameters for the environmental interpretation of peat molecular chemistry – a pyrolysis-GC/MS study. Org. Geochem. 40, 678–691. doi:10.1016/j.orggeochem.2009.03.006
Schreuder, L. T., Hopmans, E. C., Stuut, J. B. W., Sinninghe Damsté, J. S., and Schouten, S. (2018). Transport and deposition of the fire biomarker levoglucosan across the tropical North Atlantic Ocean. Geochim. Cosmochim. Acta 227, 171–185. doi:10.1016/j.gca.2018.02.020
Sobek, S., Durisch-Kaiser, E., Zurbrügg, R., Wongfun, N., Wessels, M., Pasche, N., et al. (2009). Organic carbon burial efficiency in lake sediments controlled by oxygen exposure time and sediment source. Limnol. Oceanogr. 54, 2243–2254. doi:10.4319/lo.2009.54.6.2243
Stankiewicz, B. A., Mastalerz, M., Hof, C. H. J., Bierstedt, A., Flannery, M. B., Briggs, D. E. G., et al. (1998). Biodegradation of the chitin-protein complex in crustacean cuticle. Org. Geochem. 28, 67–76. doi:10.1016/S0146-6380(97)00113-7
Thevenon, F., Adatte, T., Spangenberg, J. E., and Anselmetti, F. S. (2012). Elemental (C/N ratios) and isotopic (δ15Norg, δ13Corg) compositions of sedimentary organic matter from a high-altitude mountain lake (Meidsee, 2661 m a.s.l., Switzerland): Implications for Lateglacial and Holocene Alpine landscape evolution. Holocene 22, 1135–1142. doi:10.1177/0959683612441841
Tolu, J., Gerber, L., Boily, J. F., and Bindler, R. (2015). High-throughput characterization of sediment organic matter by pyrolysis–gas chromatography/mass spectrometry and multivariate curve resolution: A promising analytical tool in (paleo)limnology. Anal. Chim. Acta 880, 93–102. doi:10.1016/j.aca.2015.03.043
Tolu, J., Rydberg, J., Meyer-Jacob, C., Gerber, L., and Bindler, R. (2017). Spatial variability of organic matter molecular composition and elemental geochemistry in surface sediments of a small boreal Swedish lake. Biogeosciences 14, 1773–1792. doi:10.5194/bg-14-1773-2017
Tonolla, M., Storelli, N., Danza, F., Ravasi, D., Peduzzi, S., Posth, N. R., et al. (2017). “Lake Cadagno: Microbial life in crenogenic meromixis,” in Ecology of meromictic lakes, ecological studies. Editors R. D. Gulati, E. S. Zadereev, and A. G. Degermendzhi (Cham: Springer International Publishing), 155–186. doi:10.1007/978-3-319-49143-1_7
Veuger, B., van Oevelen, D., and Middelburg, J. J. (2012). Fate of microbial nitrogen, carbon, hydrolysable amino acids, monosaccharides, and fatty acids in sediment. Geochim. Cosmochim. Acta 83, 217–233. doi:10.1016/j.gca.2011.12.016
Wahlström, N., Edlund, U., Pavia, H., Toth, G., Jaworski, A., Pell, A. J., et al. (2020). Cellulose from the green macroalgae ulva lactuca: Isolation, characterization, optotracing, and production of cellulose nanofibrils. Cellulose 27, 3707–3725. doi:10.1007/s10570-020-03029-5
Wakeham, S. G., Lee, C., Hedges, J. I., Hernes, P. J., and Peterson, M. J. (1997). Molecular indicators of diagenetic status in marine organic matter. Geochim. Cosmochim. Acta 61, 5363–5369. doi:10.1016/S0016-7037(97)00312-8
Wang, X. C., Druffel, E. R. M., Griffin, S., Lee, C., and Kashgarian, M. (1998). Radiocarbon studies of organic compound classes in plankton and sediment of the northeastern Pacific Ocean. Geochim. Cosmochim. Acta 62, 1365–1378. doi:10.1016/S0016-7037(98)00074-X
Warnes, M. G. R., Bolker, B., Bonebakker, L., Gentleman, R., and Huber, W. (2016). Package gplots var R program tools plotting data. Avaliable At: https://github.com/talgalili/gplots.
Wickham, H. (2016). ggplot2: Elegant graphics for data analysis. Cham: Springer. doi:10.1007/978-3-319-24277-4
Wirth, S. B., Gilli, A., Niemann, H., Dahl, T. W., Ravasi, D., Sax, N., et al. (2013). Combining sedimentological, trace metal (Mn, Mo) and molecular evidence for reconstructing past water-column redox conditions: The example of meromictic Lake Cadagno (Swiss Alps). Geochim. Cosmochim. Acta 120, 220–238. doi:10.1016/j.gca.2013.06.017
Wörner, S., and Pester, M. (2019). Microbial succession of anaerobic chitin degradation in freshwater sediments. Appl. Environ. Microbiol. 85, 009633–e1019. doi:10.1128/AEM.00963-19
Xiong, Y., Guilbaud, R., Peacock, C. L., Cox, R. P., Canfield, D. E., Krom, M. D., et al. (2019). Phosphorus cycling in Lake Cadagno, Switzerland: A low sulfate euxinic ocean analogue. Geochim. Cosmochim. Acta 251, 116–135. doi:10.1016/j.gca.2019.02.011
Zanchetta, E., Damergi, E., Patel, B., Borgmeyer, T., Pick, H., Pulgarin, A., et al. (2021). Algal cellulose, production and potential use in plastics: Challenges and opportunities. Algal Res. 56, 102288. doi:10.1016/j.algal.2021.102288
Zhu, R., Tolu, J., Deng, L., Fiskal, A., Winkel, L. H., and Lever, M. A. (2020). Improving the extraction efficiency of sedimentary carbohydrates by sequential hydrolysis. Org. Geochem. 141, 103963. doi:10.1016/j.orggeochem.2019.103963
Zong, P., Jiang, Y., Tian, Y., Li, J., Yuan, M., Ji, Y., et al. (2020). Pyrolysis behavior and product distributions of biomass six group components: Starch, cellulose, hemicellulose, lignin, protein and oil. Energy Convers. Manag. 216, 112777. doi:10.1016/j.enconman.2020.112777
Keywords: organic matter preservation, pyrolysis-GC/MS, neutral sugar, amino sugar, redox history, organic carbon sources, meromixis, lacustrine sediments
Citation: Gajendra N, Berg JS, Vogel H, Deng L, Wolf SM, Bernasconi SM, Dubois N, Schubert CJ and Lever MA (2023) Carbohydrate compositional trends throughout Holocene sediments of an alpine lake (Lake Cadagno). Front. Earth Sci. 11:1047224. doi: 10.3389/feart.2023.1047224
Received: 17 September 2022; Accepted: 21 July 2023;
Published: 10 August 2023.
Edited by:
Paula Alexandra Gonçalves, Institute of Earth Science—pole Porto, PortugalReviewed by:
Richard Vachula, Auburn University, United StatesAndrew Decker Steen, The University of Tennessee, United States
Copyright © 2023 Gajendra, Berg, Vogel, Deng, Wolf, Bernasconi, Dubois, Schubert and Lever. This is an open-access article distributed under the terms of the Creative Commons Attribution License (CC BY). The use, distribution or reproduction in other forums is permitted, provided the original author(s) and the copyright owner(s) are credited and that the original publication in this journal is cited, in accordance with accepted academic practice. No use, distribution or reproduction is permitted which does not comply with these terms.
*Correspondence: Niroshan Gajendra, niroshan.gajendra@usys.ethz.ch, gajendra.science@gmail.com; Mark A. Lever, mark.lever@austin.utexas.edu