Recent Progresses in the Design and Fabrication of Highly Efficient Ni-Based Catalysts With Advanced Catalytic Activity and Enhanced Anti-coke Performance Toward CO2 Reforming of Methane
- 1Jiangsu Key Laboratory of Atmospheric Environment Monitoring and Pollution Control, Collaborative Innovation Center of the Atmospheric Environment and Equipment Technology, School of Environmental Science and Engineering, Nanjing University of Information Science and Technology, Nanjing, China
- 2College of Light Industry and Food Engineering, Nanjing Forestry University, Nanjing, China
- 3School of Chemistry and Chemical Engineering, Shandong University of Technology, Zibo, China
- 4School of Material Science and Engineering, University of Jinan, Jinan, China
CO2 reforming of methane (CRM) can effectively convert two greenhouse gases (CO2 and CH4) into syngas (CO + H2). This process can achieve the efficient resource utilization of CO2 and CH4 and reduce greenhouse gases. Therefore, CRM has been considered as a significantly promising route to solve environmental problems caused by greenhouse effect. Ni-based catalysts have been widely investigated in CRM reactions due to their various advantages, such as high catalytic activity, low price, and abundant reserves. However, Ni-based catalysts usually suffer from rapid deactivation because of thermal sintering of metallic Ni active sites and surface coke deposition, which restricted the industrialization of Ni-based catalysts toward the CRM process. In order to address these challenges, scientists all around the world have devoted great efforts to investigating various influencing factors, such as the option of appropriate supports and promoters and the construction of strong metal-support interaction. Therefore, we carefully summarized recent development in the design and preparation of Ni-based catalysts with advanced catalytic activity and enhanced anti-coke performance toward CRM reactions in this review. Specifically, recent progresses of Ni-based catalysts with different supports, additives, preparation methods, and so on, have been summarized in detail. Furthermore, recent development of reaction mechanism studies over Ni-based catalysts was also covered by this review. Finally, it is prospected that the Ni-based catalyst supported by an ordered mesoporous framework and the combined reforming of methane will become the future development trend.
Introduction
The fossil energy derived from coal, naphtha, and natural gas has become an indispensable part of the development of human society. Therefore, with the rapid development of the human society and economy, fossil energy has been exploited in large quantity (Capellán-Pérez et al., 2014). The consumption of fossil fuels will produce a large amount of CO2 and thus cause lots of environmental problems, such as the abnormal climate change and global warming (Zecca and Chiari, 2010). Besides, CH4 is the main component of natural gas, which has been extensively considered as the second largest greenhouse gas and has a stronger greenhouse effect than CO2 (Jiang et al., 2018). Therefore, research on resource utilization of CO2 and CH4 is one of the feasible and effective methods to reduce greenhouse gas emissions and global warming (Hernández and Martín, 2019; Ruocco et al., 2019). The CO2 reforming of methane can be considered as a promising route to simultaneously utilize these greenhouse gases.
Generally, the reforming of methane can convert methane into syngas, which is the basic unit for the synthesis of high-value chemicals or fuels (Zhao et al., 2014). The reforming of methane can be divided into the following three categories based on the reactants: steam reforming of methane (SRM, CH4 + H2O → CO + 3H2), CO2 reforming of methane (CRM, CH4 + CO2 → 2CO + 2H2), and partial oxidation of methane (POM, CH4 + 1/2O2 → CO + 2H2) (Abdullah et al., 2017). Compared with the SRM and POM processes, the CRM process can simultaneously convert CH4 and CO2 greenhouse gases into the value-added syngas, which would contribute to reducing the greenhouse effect and alleviating the energy crisis (Kim et al., 2019; Dan et al., 2020). The CRM is also an intensively endothermic process owing to the high dissociation energies of CH4 and CO2 molecules and is carried out under high-temperature conditions according to thermodynamic calculation (de Dios García et al., in press). CRM reaction could produce syngas with a low H2/CO molar ratio (H2/CO = 1), which is more suitable for further utilization in Fischer–Tropsch synthesis, oxo synthesis, and hydroformylation (Niu et al., 2020). Besides, the CRM has been also considered as the advanced method of storage and transmission of solar or nuclear energy, which drives the endothermic CRM reaction (Delgado Dobladez et al., in press). The product gases are transported to consumers at remote areas, where the reverse exothermic methanation reaction can be carried out and the chemical energy will be released. Compared with SRM and POM, CRM can be considered as the promising process in view of both environmental and economical consideration (Sutthiumporn et al., 2012). However, the CRM process has two major disadvantages and challenges. On the one hand, the methane decomposition reaction (CH4 → C + 2H2) and Boudouard reaction (2CO → CO2 + C) are usually accompanied during the CRM reaction, which will cause the formation of coke deposition over the catalyst surface and thus cause the rapid deactivation of the catalyst (Erdogan et al., 2018). On the other hand, the CRM process is also simultaneously accompanied by the reverse water gas shift reaction (RWGS, CO2 + H2 → CO + H2O) and the coexistence of the RWGS will lead to the actual H2/CO ratio lower than the theoretical ratio (1.0) (Barelli et al., 2019; Yentekakis et al., 2019). Besides, the CRM reaction can only be conducted at a high temperature above 640°C based on the thermodynamic calculation due to the high activation energy of CO2 and CH4 molecules. This will inevitably cause the rapid deactivation of the Ni-based catalyst because of the thermal sintering of the metallic active sites (Chen et al., 2013). As a result, the design and preparation of the Ni-based catalyst with coke-resistant, sintering-proof, and advanced catalytic activity remains a big challenge for the industrial application of the CRM process (Ayodele and Abdullah, 2019).
The CRM catalysts can be mainly divided into two main categories: the noble metal-based catalysts and non-noble metal-based catalysts according to the cost of the active metal components (Lee and Lim, 2020). It is reported that most of the group VIII metals can be used as the active sites of the catalysts toward the CRM process (Horváth et al., 2017). Among these, noble metals, such as Pd, Rh, Pt, and Ru, perform high reactivity, excellent stability, and anti-coking performance in the long-term stability test (Anil et al., 2020). Although noble metal-based catalysts perform excellent catalytic performance, they are not suitable for large-scale industrial application owing to their high cost (Ma et al., 2020). Therefore, worldwide scientists are more interested in seeking non-noble metal-based catalyst alternatives to promote the future industrial application of CRM reaction (Bu et al., 2019; Gao et al., 2020).
Group VIII non-noble metals, such as Fe, Co, and Ni, have been extensively investigated as the effective catalysts for the CRM process (Taherian et al., 2017). Among these metals, the Ni-based catalysts are considered as the most promising catalysts for CRM reaction due to various advantages, such as high catalytic activity and affordable cost (Zhang J. et al., 2020). However, the coke deposition is easily formed over the surface of the Ni-based catalysts at high temperatures, which will make the catalysts to deactivate rapidly because of the coke coverage of the metallic Ni active sites (Xu et al., 2014b). Besides, the metallic Ni nanoparticles easily suffered from thermal sintering due to the low Tammann temperature of the metallic Ni, which also cause the rapid deactivation of the catalysts (Bu et al., 2019). Therefore, the development of Ni-based catalysts with outstanding coke-resistant and sintering-proof performance of the catalysts has gradually become the research focus in the field of CRM.
Generally, basic research and deep understanding of the design and fabrication of Ni-based catalysts will contribute to the configuration of CRM catalysts with excellent activity, sintering-proof property, and coke resistance, which will be beneficial for the successful application in future industry. In recent years, many reviews of CRM reaction catalysts have been reported from different aspects, such as catalyst components (Abdulrasheed et al., 2019), CRM reaction at low temperature (Wang Y. et al., 2018a), and influence of process parameters (Usman et al., 2015). However, the content of the review on this topic is still incomplete, and the prospect of the recent research progresses in the literature review is not enough. Therefore, it is necessary to summarize the recent research progress of nickel-based catalysts with advanced catalytic activity and enhanced anti-coke performance. This review comprehensively summarizes the recent development of Ni-based catalysts with particular attention to the influences of the supports, additives, and preparation methods on the improvement of the catalytic performance of Ni-based catalysts. Besides, the reaction mechanisms of the CRM over Ni-based catalysts are also summarized. Finally, the future development trend of the Ni-based catalysts for the CRM process is prospected.
Ni-based Catalysts for CRM
The catalytic properties of Ni-based catalysts for CRM reaction are affected by various influencing factors, such as the size of the metallic Ni active site, feature of the support, addition of the promoter addition, and metal–support interaction. Recently, Ni-based catalysts have attracted more and more attention because of their high activities comparable to the noble metals and their low cost (Zhang et al., 2019). However, Ni-based catalysts usually suffer from rapid deactivation due to surface coke deposition and thermal sintering of metallic Ni active sites during the CRM process (Bu et al., 2020). Therefore, great efforts have been devoted to the improvement of surface coke deposition resistance, sintering-proof property of the metallic Ni, and catalytic activity by selecting appropriate support, doping promoted additives, and developing a new preparation method for the catalyst. Several recently developed catalysts for CRM reaction are summarized in Table 1, which differ in terms of the types of supports and promoters, and preparation method.
Ni-Based Catalysts With Different Supports
As well-known, catalytic support is considered as an important component of the catalyst by determining the dispersion of the metallic active sites, thereby further influencing the catalytic performance of the catalyst (Sassi et al., 2017). Meanwhile, the metal–support interaction can also greatly affect various features of the catalyst, such as the dispersion, particle size, and metal–support interface, which will further influence the catalytic activity, coke-resistant property, and sintering-proof performances of the catalyst (Bu et al., 2020). Besides, the instinct properties of the support, such as acid–base property and redox property, also influence the activation process of CO2, which will further reduce the possibility of the side reactions (Silveira et al., 2017; Azancot et al., 2019). Various materials, such as metal oxides, silica, composite metal oxides, ordered mesoporous materials, zeolites, and carbon materials, have been widely investigated as the supports of Ni-based catalysts toward CRM reaction, which will be presented in the following text.
Oxide Supports
Single oxide supports
Oxide materials, such as CeO2 (Luisetto et al., 2019), SiO2 (Zhang L. et al., 2019; de la Cruz-Flores et al., 2020), Al2O3 (Rahbar Shamskar et al., 2017), MgO (Zhou et al., 2018), and ZrO2 (Wang Y. et al., 2018b), are widely utilized as supports of Ni-based catalysts for the CRM process. For example, CeO2 has been extensively investigated as a support of Ni-based catalysts because of the formation of a strong metal–support interaction, which can contribute to the enhancement of the reactivity by facilitating the CH4 dissociation over the metallic Ni active site (Yan et al., 2019). Besides, the CeO2 with a cubic fluorite structure can possess the unique oxidation state through the reversible redox transformation between Ce4+ and Ce3+ (Luisetto et al., 2019). Specifically, the oxygen formed via the CO2 dissociation process can be absorbed by oxygen vacancies on the CeO2 surface (Tu et al., 2019). Wang N. et al. (2016) synthesized a series of Ni-based catalysts with CeO2 supports with four kinds of morphologies (nanorod, nanocube, nanoocta, and nanoparticle, Figure 1) and investigated the influence of the morphology and crystal plane on the catalytic performance of the CRM reaction. As shown in Figure 2, the Ni/CeO2-nanorod catalyst displayed the highest catalytic performance and best stability in the CRM reaction among the investigated Ni/CeO2 catalysts. Therefore, the structure and catalytic performance of the Ni/CeO2 catalyst can be adjusted by tuning the morphology of the CeO2 support. Li and van Veen (2018) confirmed that the Ni nanoparticles could be highly dispersed because of the strong metal–support interaction between Ni and CeO2. Thus, no serious thermal sintering of the metallic Ni active site occurred during the CRM reaction.
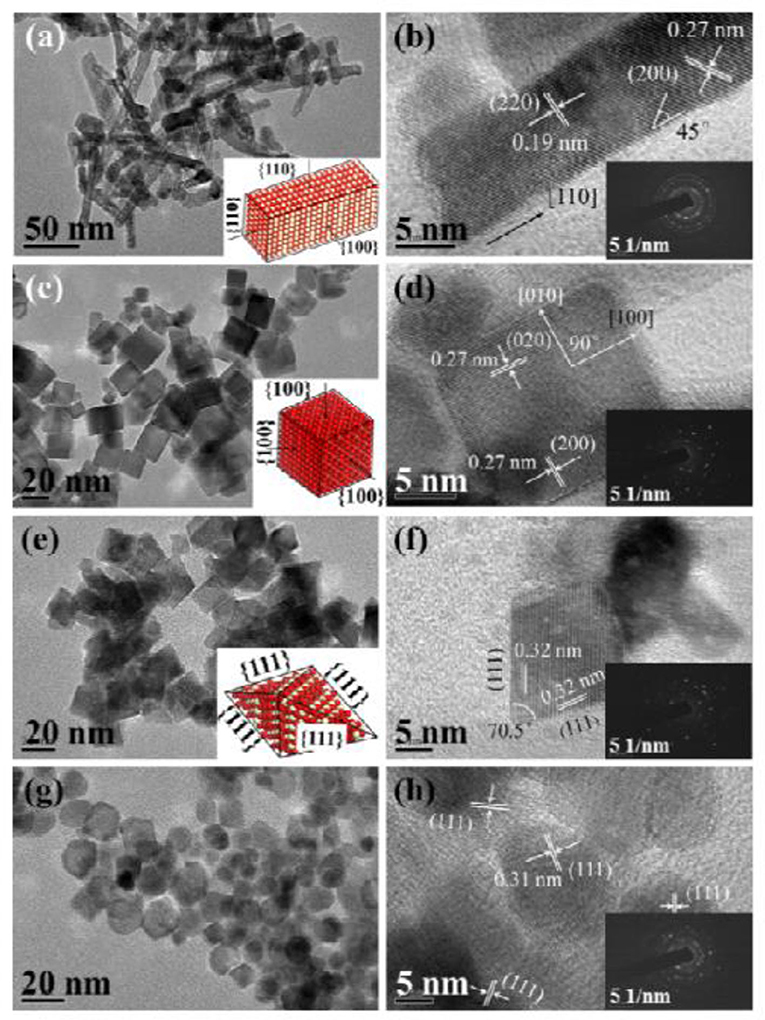
Figure 1. TEM, HRTEM, and SAED (inset) images of as-obtained CeO2: (a,b) NRs, (c,d) NCs, (e,f) NOs, and (g,h) NPs. Reproduced from Wang N. et al. (2016) with permission from Catalysis Science and Technology.
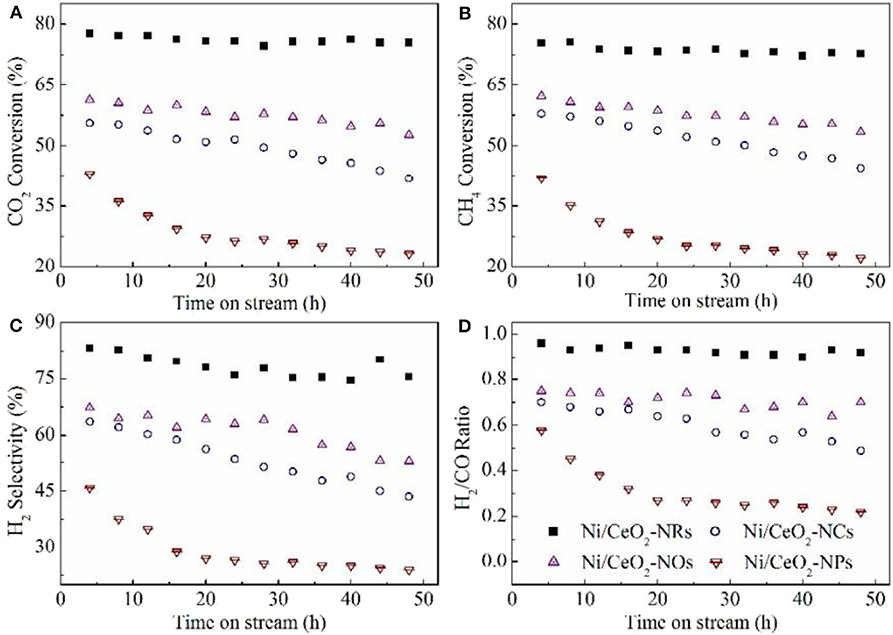
Figure 2. Catalytic stability test of the Ni/CeO2 samples for methane dry reforming at 700°C for 50 h. (A) CO2 conversion; (B) CH4 conversion; (C) H2 selectivity; (D) H2/CO ratio. Reproduced from Wang N. et al. (2016) with permission from Catalysis Science and Technology.
SiO2 is widely employed as the support of the Ni-based catalyst owing to its thermally stable feature. Besides, the low surface acidity of SiO2 can reduce the coke deposition over the catalyst surface (de la Cruz-Flores et al., 2020). However, it is very difficult to form a strong metal–support interaction over the SiO2-supported catalysts, which causes rapid deactivation of the catalysts because of the thermal agglomeration of the metallic Ni active site (Zhao et al., 2016). Therefore, a series of Ni@SiO2 core–shell catalysts have been widely investigated in this field (Wang F. et al., 2018). In this system, the SiO2 shell can encapsulate the metallic Ni active sites, which can effectively suppress the thermal sintering of the metallic Ni (Wang F. et al., 2016a). Zhang L. et al. (2019) fabricated the Ni@SiO2 core–shell catalyst by the microemulsion method with ultrafine Ni nanoparticles (<3.0 nm) for CRM reaction. They found that the catalytic performance of the catalyst was affected by the size of the Ni nanoparticle and the metal–support interaction between Ni and SiO2. Besides, the shape of SiO2 nanospheres did not obviously change even under high calcination and CRM reaction temperatures, demonstrating good thermal stability. Li et al. (2018) synthesized Ni@Ni phyllosilicate@SiO2 core–shell hollow sphere catalysts for CRM reaction by the hydrothermal and reduction method. Ni@NiPhy@SiO2 exhibited higher carbon resistance than the Ni@NiPhy catalyst without a SiO2 shell at a lower reaction temperature (600°C). The interaction of Ni and NiPhy materials on the Ni@Niphy@SiO2 catalyst increased because of the presence of the SiO2 shell, which inhibited the growth of Ni nanoparticles. Thus, the growth of CNTs was eliminated and high carbon-resistant performance could be obtained. Das et al. (2018) synthesized an innovative sandwiched core–shell structured Ni-SiO2@CeO2 catalyst with Ni nanoparticles encapsulated between SiO2 and CeO2. Ni-SiO2@CeO2 showed better anti-coking property than Ni-SiO2 and Ni-CeO2 under low-temperature (600°C) CRM reaction because of the confinement effect of the sandwich structure and the bifunctional mechanism of dry reforming.
As for the Al2O3 support, it can effectively disperse Ni particles because of its large surface area, favorable thermal stability, and strong metal–support interaction (Rahbar Shamskar et al., 2017). At the same time, the unique acid–base property of Al2O3 can play a synergistic role with the metallic active site (Shang et al., 2017). Pizzolitto et al. (2019) studied the role of the different kinds of supports in influencing the catalytic performance. They found that the Ni/Al2O3 catalyst performed much better catalytic stability than did the Ni/CeO2 catalyst because of strong metal–support interaction. Xu Y. et al. (2019) compared the catalytic performances of CRM over the Ni/SiO2 and Ni/Al2O3 catalysts. It was found that the Ni/SiO2 catalyst suffered rapid deactivation because of the weak metal–support interaction, which caused the thermal sintering of the metallic Ni and further accelerated the formation of the coke. As a comparison, the Ni/Al2O3 catalyst performed much better catalytic stability because of the strong metal–support interaction. This would facilitate the formation and stabilization of small Ni nanoparticles and further reduce the surface coke deposition over the Ni/Al2O3 catalyst.
Similar to Al2O3, ZrO2 also possesses both basic and acidic properties and is widely used as the catalytic support (Shin et al., 2018). As a support, it can form the strong metal–support interaction with the Ni active site. Therefore, the metallic Ni nanoparticles can be well-dispersed on the support, which is further conducive to the improvement of the catalytic activity and anti-coke property of the catalyst (Nabgan et al., 2020). Wang Y. et al. (2018b) confirmed that the metal–support interaction of the Ni-Si/ZrO2 catalyst not only facilitated the formation of small metallic Ni active sites with strong electron donor capability but also maintained the state of the Ni particles under CRM reaction conditions.
MgO is widely employed as the support of the Ni-based catalyst owing to its thermally stable feature and low cost (Zanganeh et al., 2013). Besides, its strong Lewis basicity catalyst has a strong chemisorption effect toward CO2 and can inhibit coke deposition by accelerating the coke-eliminating reaction (Zhang et al., 2018). Zuo et al. (2018) used theoretical modeling and experimentation to study the catalytic performance of single-site Ni/MgO catalysts in CRM reactions. As the particle size of Ni increases, the single-site Ni/MgO catalyst provided stronger bindings and more sufficient sites to activate CH4 and CO2. Song et al. (2020) evaluated the catalytic activity of a Ni–Mo nanocatalyst, which were stabilized at the edges of a single-crystalline MgO. NiMoCat showed stable activity and high conversion rates of CH4 and CO2 in 850 h of continuous running test. The activity values of NiMoCat were much greater than many traditional catalysts.
As an alkaline support, La2O3 is also conducive to the adsorption and activation of CO2 on the surface of Ni-based catalysts (Li et al., 2017). Meanwhile, it can react with CO2 to produce La2O2CO3, thereby reacting with coke on the surface of nearby Ni nanoparticles to produce CO and regenerate La2O3 (Tsoukalou et al., 2016; Xu L. et al., 2019). Xu L. et al. (2019) used various methods, such as glycine nitrate combustion (GNC), precipitation (PP), and thermal decomposition (TD) to prepare La2O3 supports with different bulk and surface properties to study the structure–reactivity relationship of Ni/La2O3. Among these catalysts, the 5Ni/La2O3-GNC catalyst with the most surface alkaline and active oxygen sites displayed the best catalytic activity and stability. Li et al. (2017) tested the catalytic activity of the Ni/La2O3-LOC catalyst synthesized with La2O2CO3 nanorod as the support precursor. As shown in Figure 3, the activity of 5Ni/La2O3-LOC is higher than that of 5Ni/La2O3 because the nanorod support of 5Ni/La2O3-LOC improved the dispersion of nickel particles and increased the number of exposed active sites. They also found that the dispersion of Ni affected the distribution of coke and La2O2CO3 on the catalyst surface, which in turn affected the catalytic performance of the catalyst. Gao et al. (2018) doped the La2O3 into carbon fibers (CF) as a support to prepare the NI/CF-LA2O3 catalyst. The addition of La2O3 improved the activity of the Ni/CF catalyst because La2O3 could improve the dispersion of Ni and enhance the metal–support interaction between Ni and support. In addition, the conversion of La2O3 to La2O2CO3 affected the removal rate of deposited carbon and improved the catalyst activity of Ni/CF-La2O3.
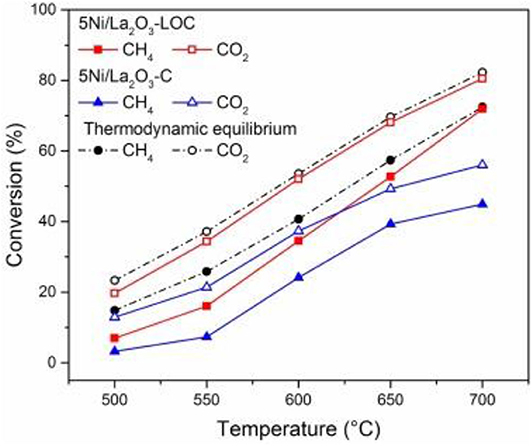
Figure 3. DRM activity of the catalysts. Reaction conditions: CH4/CO2/N2 = 15/15/70 mL/min, GHSV = 60,000 mL h−1 , 1 atm. Reproduced from Li et al. (2017) with permission from Applied Catalysis B: Environmental.
Composite oxides
In addition to the single oxides, the composite oxides are also often used as the supports of the Ni-based catalysts for CRM reactions because it can overcome the disadvantages of the single oxides and further improve the catalytic performance of the catalysts (Shah et al., 2019). For example, the CeO2 support possesses excellent redox properties; however, its thermal stability was relatively poor, especially under CRM conditions (Long et al., 2020). In order to address this challenge, doping another metal cation into the crystal structure of CeO2 or depositing another basic metal oxide (such as La2O3, Al2O3, ZrO2) over the CeO2 surface has been considered as an effective strategy (Padi et al., 2020). ZrO2 has excellent thermal stability at high temperature and thus the introduction of Zr4+ cation into the CeO2 lattice to form a CeO2-ZrO2 solid solution can greatly improve the oxygen storage capacity, oxygen mobility, and thermal stability, which further enhances the anti-coke property (Shi et al., 2019). The partial substitution of Ce4+ by Zr4+ causes the shrinkage and deformation of the CeO2 lattice and then more oxygen vacancies are formed, which induces enhanced mechanical properties and ion conductivity (Wang Y. et al., 2019). Furthermore, the low concentration of Lewis acid sites on the surface of the CeO2-ZrO2 solid solution support can effectively reduce the coke deposition caused by the surface acidity (Xu et al., 2012a). Dudek et al. (2019) evaluated the effect of CeO2-ZrO2 support prepared by supercritical fluid method on the performance of Ni-based catalysts. The NiOx/CeO2-ZrO2 catalyst displayed favorable thermal stability and catalytic performance. They also found that the employment of the continuous supercritical preparation techniques to prepare Ni-based catalysts could improve its resistance to deactivation. Jang et al. (2018) compared the catalytic performance of Ni-based catalysts supported on CeO2, ZrO2, and CeO2-ZrO2 solid solution toward the CRM reaction. As shown in Figure 4, the Ni-MgO-Ce0.8Zr0.2O2 catalyst performed the best stability and catalytic activity due to the highest degree of reduction and the smallest metallic Ni particle size. CeO2-Al2O3 composite oxide is also considered as an excellent support for CRM reaction because the presence of CeO2 can greatly improve the metal–support interaction and the stability of Al2O3 at high temperature (Stroud et al., 2018). According to the previous report, the CeO2-Al2O3 support is beneficial to dispersing the active sites, improving the reducing ability of the catalyst, and enhancing the oxygen flow (Jawad et al., 2019).
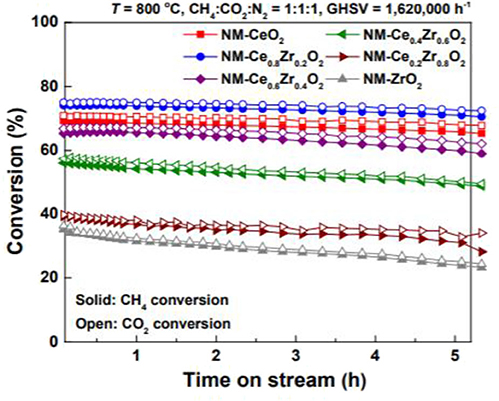
Figure 4. CH4 and CO2 conversions with time on stream (TOS) over NMCeO2, NM-ZrO2, and NM-Ce(1−x)Zr(x)O2 catalysts in CRM reaction (T = 800°C, CH4:CO2:N2 = 1:1:1, and GHSV = 1,620,000 mL·g−1·h−1). Reproduced from Jang et al. (2018) with permission from Green Chemistry.
For TiO2-Al2O3, TiO2 can effectively prevent coke deposition because of its reducible properties (Kim et al., 2015). At the same time, the presence of Al2O3 with a high surface area can overcome the shortcomings of TiO2 support, such as the low surface area and the change of crystalline phase during the CRM reaction (Shah et al., 2020). Shah et al. (2019) compared the catalytic activity and stability of Ni-based catalysts with different TiO2/Al2O3 molar ratios. The results of their study indicated that the synergistic effect of TiO2 and Al2O3 prevented the formation of coke and the occurrence of side reactions. The catalyst with the most suitable doping ratio of TiO2 and Al2O3 could improve the catalytic activity and stability of the catalyst. Shah et al. (2020) changed the synthesis parameters to design and optimize the nanocrystalline mesoporous Ni-based catalyst toward CRM reaction. They found that the TiO2-Al2O3 mixed oxide was the main driving force for the sustainable activity of the catalyst.
For MgO-Al2O3, it was found that the interaction between the active metal and the support was enhanced by the formation of MgAl2O4, thus improving the dispersion of Ni on the support and reducing the catalyst deactivation caused by coking (Bach et al., 2020). Jin et al. (2017) prepared a carbon-Ni/MgO-Al2O3 composite catalyst by using sucrose as the precursor and tested its catalytic performance toward CRM reaction. The results showed that the composite catalyst performed good coking resistance and could inhibit the growth of metallic Ni active sites. They also found that the carbonization temperature of the composite catalyst precursor can affect the activity of this catalyst. Chai et al. (2017a) synthesized a sort of NiO-MgO-Al2O3/FeCrAl-fiber catalyst with a microfiber structure for DRM reaction, and the obtained catalyst showed excellent catalytic activity and stability. The Ni nanoparticles in the catalyst were evenly nested in the MgO-Al2O3 nanosheet composites, which was crucial for the formation of rapid carbon filaments.
Zeolite Supports
Zeolites are a group of crystalline aluminosilicate materials with microporous structures and are widely investigated as supports of the Ni-based catalysts of the CRM reaction because of their advantages of abundant microporous channels, good thermal stabilities, large specific surface areas, and big pore volumes (Martínez Galeano et al., 2019; Kumar et al., 2020; Zhu et al., 2020). The Si/Al ratio of zeolite reflects the number of charge compensating ions and can modify the surface acidity (Li W. et al., 2019). In view of these advantages, the zeolites have been widely investigated as catalytic supports of metal or oxide clusters in many catalytic processes owing to the high metal dispersions and excellent stabilities (Xie et al., 2019). Moradi et al. (2016) confirmed that the Si/Al ratio plays an important role in affecting the catalytic performance of the nano-Ni/ZSM-5 catalyst in the CRM reaction. They prepared a series of Ni/ZSM-5 nano-catalysts with different Si/Al ratios. The experimental results showed that the acid catalyst with the lowest Si/Al ratio [5NZ(30)] had excellent catalytic performance and coke resistance in the CRM process. They also found that the specific surface area and pore volume of ZSM-5 zeolite would increase as the Si/Al ratio decreased. Vafaeian et al. (2013) prepared a series of Ni/ZSM-5 nanocatalysts with different Ni loading amounts by the ultrasonic assisted method. As shown in Figure 5, the Ni/ZSM-5 nanocatalyst with the Ni content of 8% showed excellent catalytic activity because of its high specific surface area, uniform particle size distribution, and high dispersion of metallic Ni active sites. Estephane et al. (2015) investigated the catalytic performances of ZSM-5-supported Ni and/or Co monometallic and bimetallic catalysts prepared by the wet impregnation method. Among these catalysts, the bimetallic catalyst with high Co loading amounts (1Ni2Co/ZSM5) performed the best catalytic activity and the lowest coke deposition after 12 h of operation.
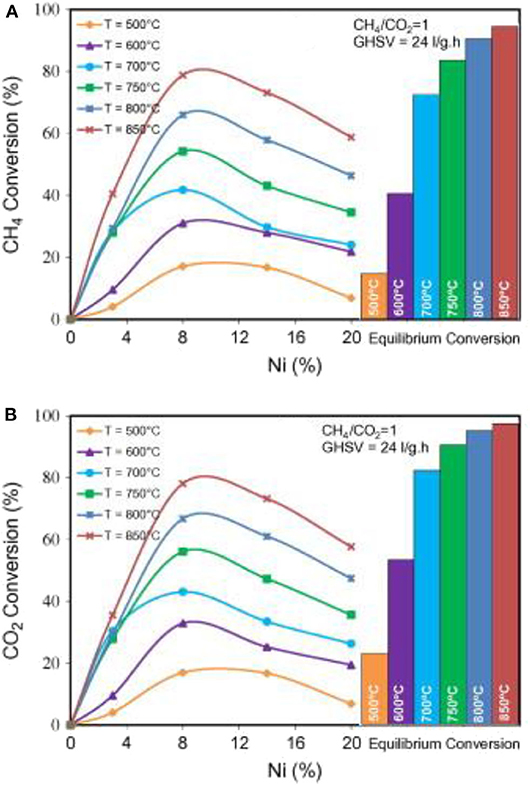
Figure 5. Effect of Ni loading on CH4 (A) and CO2 (B) conversion at different temperatures over nanostructured Ni/ZSM-5 catalysts synthesized via the ultrasound method. Reproduced from Vafaeian et al. (2013) with permission from Energy Conversion and Management.
Silicalite-1 (S-1) is a sort of zeolite with weak acidity owing to no alumina component in the zeolite framework (Wei et al., 2017). The diffusion rates of H2, CO, CO2, and CH4 in S-1-supported catalyst are very fast (Laprune et al., 2017). Bawah et al. (2018) confirmed that mesoporous Na-silicalite-1 (MFI) zeolite was a suitable support material for the CRM reaction catalyst. The ion exchange between MFI and Ce significantly increased the mesopore volume, improved the activity of Ni/silicalite-1, and reduced the formation of carbon deposition. The reduction in diffusion restrictions and the high surface area made the sintered Ni particles smaller and the catalytic activity of the catalyst increased. In recent years, hollow zeolite has been widely used in CRM reactions due to its excellent properties in product selectivity, anti-active site leaching, and anti-metal sintering (Dai et al., 2015a). Dai et al. (2015b) confirmed that the 1.5Ni-0.5 Pt@Hol S-1 catalyst obtained by encapsulating highly dispersed Ni-Pt bimetallic nanoparticles in hollow silicalite-1 single crystals performed good anti-coking and sintering properties in CRM reactions. As shown in Figure 6, Ni was wrapped in hollow zeolite crystals and the small Ni particles inhibited the formation of coke. The encapsulation shell prevented coke formed on the outside from reducing the activity of the Ni inside, resulting in a stable and high activity even in the presence of carbon.
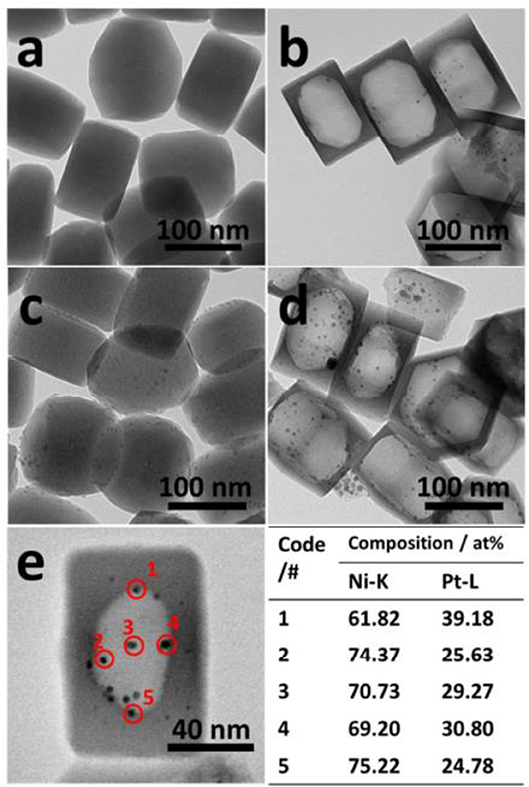
Figure 6. TEM images of prepared (a,c) 1.5NiO-0.5Pt/S-1 and (b,d) 1.5NiO-0.5Pt@Hol S-1 after calcination in air at 400°C for 2 h (a,b) and reduction under H2 at 800°C for 30 min (c,d). (e) STEM image of prepared 1.5NiO-0.5Pt@Hol S-1 after reduction under H2 at 800°C for 30 min, and quantified phase compositions in the Ni-Pd nanoparticles from STEM maps. Reproduced from Dai et al. (2015b) with permission from Journal of Materials Chemistry A.
Carbon Supports
Carbon materials, such as activated carbon (AC) (Wang H. et al., 2019), carbon nanotubes (CNT) (Figueira et al., 2018), and carbon fiber (CNF) (Chai et al., 2017b), have been widely used as supports of the Ni-based catalysts of the CRM reaction. Because the carbon-based materials usually have lots of advantages, such as large surface areas, excellent stability, chemical inertness, and low cost (Li et al., 2020). Their pore-size distributions and surface functionalities could be readily tuned by controlling specific parameters (Sun et al., 2018). In addition, the recovery of active metals from spent catalysts can be achieved and recycled by burning the carbon supports, especially for the precious metal-based catalysts (Khavarian et al., 2014). Besides, the surface oxygen-containing functional groups can be formed after the oxidation treatment of AC. The surface oxygen-containing functional groups can act as nucleation centers, which is beneficial to the high dispersion of the metallic active site over the surface of AC (Forouzesh et al., 2019). Tan et al. (2019) compared the difference between the wood-derived activated carbon (WAC) and coal-derived activated carbon (CAC) as the catalysts toward the microwave-assisted CRM. The results demonstrated that WAC performed much better catalytic activity toward the CRM reaction. The reason for this was that the WAC had a larger specific surface area and bigger specific pore volume than the CAC. Thus, WAC could provide more accessible active sites, which was conducive to the adsorption and diffusion of gas. Wang H. et al. (2019) reported that the synergistic effect between the Ni/AC and plasma could greatly improve the catalytic activity toward CRM reaction by intensifying the Ni-AC metal–support interaction. Sun et al. (2019b) prepared Co–M/AC–N (M = Ce, Fe, Zr) catalysts by co-impregnation and evaluated their catalytic performances toward CRM reaction. They found that the doping of N resulted in a higher Co2+/Co3+ ratio and improved the stronger interaction between Co and Ce. Therefore, the addition of N could increase the catalytic activity of the catalyst.
Carbon nanotube (CNT), well-known as a new nano-carbon catalyst support, possesses many advantages, such as large surface area, unique electronic properties, tubular structure, chemical inertness, thermal stability, and high mechanical strength (Figueira et al., 2018). Therefore, it can effectively improve the dispersion of metal particles and enhance the adsorption of reactants (Figueredo et al., 2018). Ma et al. (2013) prepared a series of catalysts for the CRM reaction of Ni nanoparticles loaded inside or outside CNTs and studied the effect of catalytic sites on the surface of CNTs. As shown in Figure 7, the metallic Ni nanoparticles loaded inside CNT catalysts showed the best catalytic activity among these catalysts. This could be attributed to the difference in electronic density between interior and exterior surfaces of CNTs and the confinement effect of CNTs. Donphai et al. (2014) prepared the composites between Ni and CNTs over mesocellular silica support (Ni-CNTs/MS) for CRM reactions. The results showed that the Ni-CNTs/MS catalyst had better stability than the Ni/MS catalyst due to selective formation of carbon by-products as the tube-length extension of the existing CNTs.
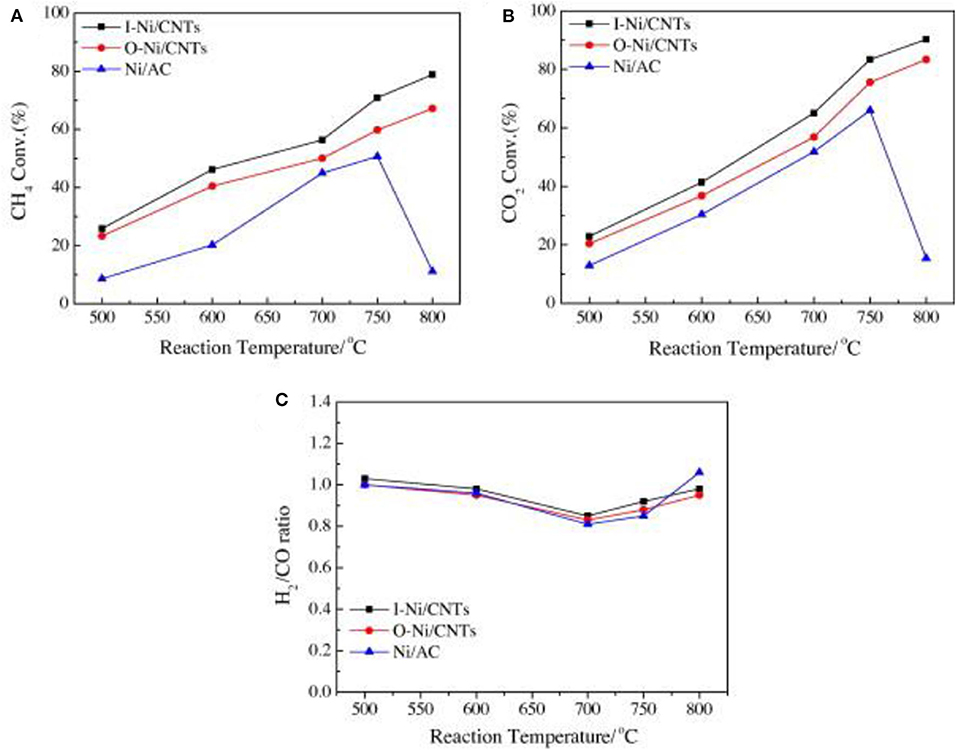
Figure 7. The conversion–temperature relationships of Ni/AC, O–Ni/CNT, and I–Ni/CNT catalysts. (A) CH4 conversion; (B) CO2 conversion; (C) H2/CO ratio (reaction conditions: W/F = 1 g h/mol). Reproduced from Ma et al. (2013) with permission from fuel.
As for carbon fiber, it has a similar thermal stability and high specific surface area to the activated carbon. Besides, their fibrous properties make them more beneficial to producing structurally defined catalysts (Wei et al., 2020). Wei et al. (2020) demonstrated that the carbon fiber with layered silicate could improve the sintering performance of the Ni-based catalyst at high temperature of CRM because carbon fiber favored the decomposition of CO2. Yu et al. (2018) prepared Ni-based catalysts with different ratios of Al2O3/ACF for CRM reaction. They found that the incorporation of the activated carbon fibers (ACF) to the Ni/γ-Al2O3/ACF catalyst was beneficial to improvement of the CRM catalytic activity. ACF could reduce the grain size of NiAl2O4 spinel in Ni-based catalysts, which was conducive to the conversion of methane. The addition of ACF could provide abundant functional groups on the catalyst surface and effectively promote the catalytic performance of the CRM reaction.
Ordered Mesoporous Materials
Ordered mesoporous materials are extensively employed as supports of the Ni-based catalysts for CRM reaction because of their outstanding advantages in textural properties, such as large specific surface areas, tunable pore sizes, and big volumes, which are beneficial to the high dispersion of the active sites (Xu et al., 2013). Furthermore, the confinement effect of the mesopore can effectively suppress the growth of metallic Ni nanoparticles within the size of the mesopore (Xu et al., 2016b). As a result, the metallic Ni active sites with uniform size distribution can be formed and the thermal sintering of the metallic Ni active sites can be inhibited during the CRM reactions (Xu et al., 2012a; Al-Fatesh et al., 2018). Among them, the ordered mesoporous metal (composite) oxides and ordered mesoporous silica material have been widely investigated as catalytic supports in CRM reaction (Xu et al., 2016b; Bukhari et al., 2017).
Recently, the ordered mesoporous alumina with high thermal stability and outstanding structural property have attracted more and more attention (Xu et al., 2016a). Li B. et al. (2019) incorporated the secondary metal (M = Fe, Co, Cu) into the Ni-based ordered mesoporous alumina catalyst (MNiAl) for CRM reaction. Among these catalysts, FeNiAl displayed the best catalytic activity owing to the formation of FeNi3 alloy during the CRM reaction. Xu et al. (2012c) prepared a series of Ni-based catalysts supported on ordered mesoporous alumina (OMA) for CRM reaction. These catalysts exhibited excellent catalytic activity and stability. They confirmed for the first time that the mesoporous frameworks of these catalysts could provide more “accessible” Ni active centers and effectively control the size of Ni nanoparticles through the confinement effect of mesoporous during the reaction. Xu et al. (2013) synthesized a series of ordered mesoporous MgO-Al2O3 composite oxides with different Mg contents as a support for Ni-based catalysts for CRM reactions. Figure 8 demonstrated a hexagonally ordered mesopore structure of the OM-xMgyAl materials. As shown in Figure 9, the conversion rates of CH4 and CO2 of 5% Ni/OM-5Mg95Al with mesoporous structure were higher than that of 5% Ni/NP-5Mg95Al with a non-mesoporous structure. Therefore, the mesoporous structure played an important role in improving the catalytic activity of the catalyst. Xu et al. (2016a) compared the catalytic performances of OMA-5Co5Ni, OMA-10Co, OMA-10Ni, and 5Co5Ni/Al2O3 catalysts toward CRM reaction. As shown in Figure 10, the OMA-5Co5Ni, OMA-10Co, and OMA-10Ni mesoporous catalysts did not suffer significant deactivation after 100-h long-term stability tests due to the confinement effect of the mesoporous framework, which effectively prevented the severe thermal sintering of the metallic Ni and/or Co active centers. In contrast, the traditional 5Co5Ni/Al2O3-supported catalyst was obviously deactivated because of the seriously thermal sintering of the metallic active sites.
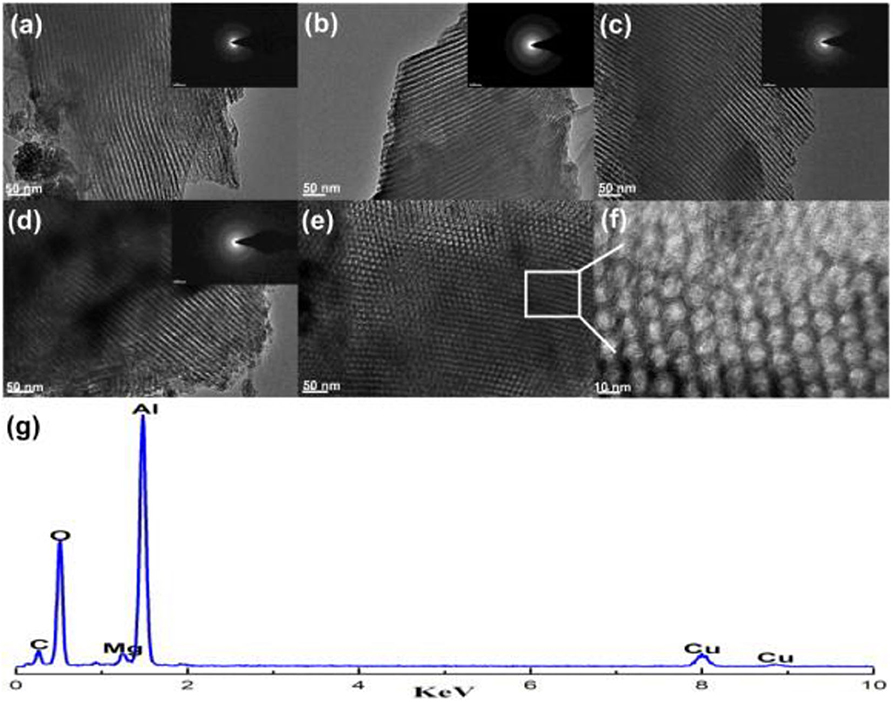
Figure 8. TEM and SAED images of the OM-xMgyAl materials calcined at 700°C: (a) OM-3Mg97Al, (b) OM-5Mg95Al, (c) OM-8Mg92Al, (d–f) OM-10Mg90Al, and (g) EDX measurement for OM-5Mg95Al. Reproduced from Xu et al. (2013) with permission from International Journal of Hydrogen Energy.
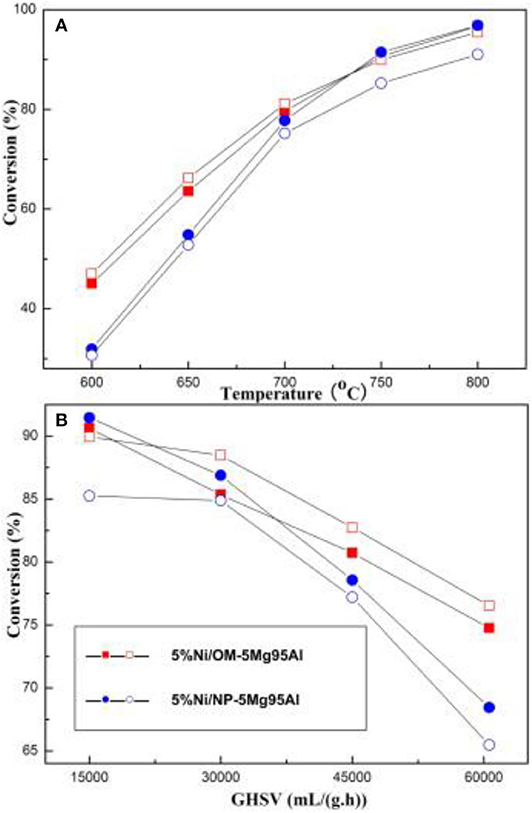
Figure 9. CH4 and CO2 conversions over the 5%Ni/OM-5Mg95Al and 5%Ni/NP-5Mg95Al catalysts under various reaction conditions: (A) T = 600–800°C, CH4/CO2 = 1, GHSV = 15,000 mL/(g h), 1 atm; (B) GHSV = 15,000–60,600 mL/(g·h), CH4/CO2 = 1, 750°C, 1 atm. Reproduced from Xu et al. (2013) with permission from International Journal of Hydrogen Energy.
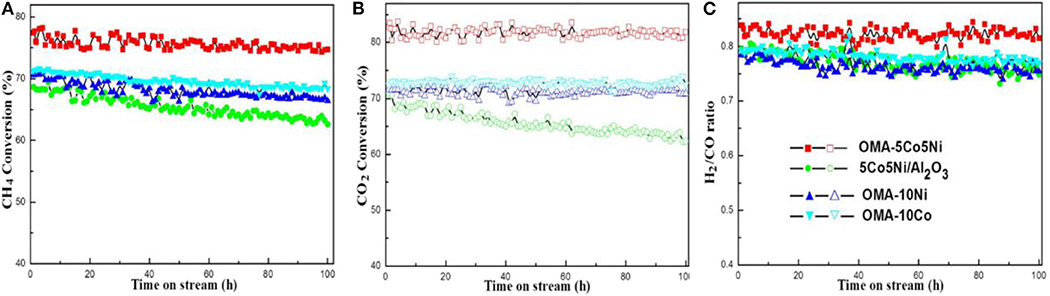
Figure 10. Long-term stability tests (100 h) over different catalysts: (A) CH4 conversion, (B) CO2 conversion, (C) H2/CO ratio; reaction conditions: CH4/CO2 = 1, GHSV = 15,000 mL/(g h), 700°C, 1 atm. Reproduced from Xu et al. (2016a) with permission from International Journal of Hydrogen Energy.
Mesoporous silica materials, such as SBA-15 (Bukhari et al., 2017) and MCM-41 (Tian et al., 2019), are also considered as promising candidates of the catalytic supports owing to their big pore volumes, high surface areas, and excellent thermal stabilities (Chong et al., 2018). Compared with the traditional silica materials, SBA-15 usually possesses an ordered two-dimensional hexagonal mesoporous network with enhanced thermal stability, which can be successfully maintained even after the severe high-temperature reaction conditions (Abdullah et al., 2019; Okutan et al., 2020). Besides, the metallic Ni active sites can be homogenously distributed among the SBA-15 pore channels, which greatly improves the dispersion of active sites over the catalyst surface and mesoporous channels. It is believed that the metal–support interface with strong interaction between the active site and the support can effectively reduce the mobility and thermal sintering of the active site, thereby reducing surface carbon deposition and slowing down the deactivation process of the catalyst (Bukhari et al., 2017). Razak et al. (2019) reported that the Ni/SBA-15 catalyst was synthesized with the oil palm ash as the raw material. The obtained material with the hexagonal ordered mesostructured, small NiO size (16 nm), and strong metal–support interaction performed high conversion rates of CH4 and CO2. Zhang et al. (2017) prepared ordered mesoporous silica catalysts (Ni-SBA-15, Ni-KIT-6, and Ni-MCM-41) using the solid-phase grinding method. Compared with other catalysts, Ni-SBA-15 showed the best stability in CRM reaction. The high dispersion of Ni species and the strong metal–support interaction between Ni and mesoporous supports reduced the coke deposition and metal sintering of Ni-SNA-15 in the CRM reaction. Yang et al. (2016) prepared a series of Ni/SBA-15-P123 catalysts for the CRM reaction using the P123-assisted impregnation method. The results showed that the SBA-15 support exhibited an excellent confinement effect during the CRM process, which indirectly proved the enrichment of Ni in the mesoporous channels of SBA-15.
In addition, the MCM-41 mesoporous silica also has distinguished structural properties, such as low surface acidity, big pore volume, large specific surface area, high-temperature structural stability, uniform pore-size distribution, and excellent absorption property, which is considered as a suitable catalytic support of the Ni-based catalyst for CRM (Wang X. et al., 2018; Al-Fatesh et al., 2019a). Tian et al. (2019) encapsulated the uniform metallic Ni nanoparticles into MCM-41 straight mesoporous channels driven by the capillary force induced by ethanol. In this way, a limited Ni/MCM-41 catalyst was prepared for CRM reaction. They found that Ni NPs were confined into mesoporous channels with strong metal–support interactions. This confined structure had a significant effect on the inhibition of metal NP agglomeration and coke deposition during the CRM process. Al-Fatesh et al. (2019a) prepared a series of Sc-promoted Ni/MCM-41 catalysts with different loading amounts. The MCM-41 material successfully maintained the mesoporous structure of hexagonal array characteristics after the impregnation of Ni and Sc.
In summary, different supports possess different structures, properties, metal-support interaction, and stability and reducibility of these Ni-based catalysts. Hence, the selection of suitable supports is considered as an important part in the study of Ni-based catalysts for CRM reactions. Compared with conventional supported catalysts, unique structures, such as core–shell structures, can provide relatively superior activity and stability for Ni-based catalysts. Therefore, it is necessary to consider whether they are suitable for large-scale industrial application in the study of Ni-based catalysts with different supports in CRM reaction.
Ni-Based Catalyst Modified With Different Additives
In order to improve the catalytic performance, various catalytic additives, such as alkaline earth metal oxides, and rare-earth metal oxides, have been extensively investigated toward CRM reaction (Swirk et al., 2020). Meanwhile, doping promoters can also improve the stability of the catalyst by enhancing the surface basicity, redox property, and pore structure of the catalyst by intensifying the coke eliminating process (Singha et al., 2016).
Alkaline Earth Metal Oxides
The incorporation of earth metal oxides, such as MgO (Xu et al., 2013) and CaO (Sun et al., 2020), could intensify the surface alkalinity and further intensify the ability of CO2 chemisorption and activation on the catalyst surface (Xu et al., 2016a). As a result, the high concentration of CO2 on the catalyst surface will make the balance of the Boudouard reaction shift to the left and consequently can reduce the generation of coke deposition (Sun et al., 2019a). Furthermore, the addition of alkaline earth promoters can also affect the degree of reduction and structural property of the catalyst (Ramezani et al., 2018).
The MgO promoter usually acts as an electron donor, and the electrons in MgO can be transferred to the metallic Ni active sites. In increasing electrons, the density around the metallic Ni could promote the activation and dissociation processes of CO2 (Jing et al., in press). It was reported that the addition of MgO can greatly enhance the catalytic activity and coke resistance of the catalyst by improving the dispersion and area of the metallic Ni active site. Besides, it was found that MgO can also effectively improve the activity and stability of the Ni-based catalyst by enhancing the metal–support interaction (Ma et al., 2019). Wang et al. (2013) synthesized the MgO-coated SBA-15 by the one-pot method. The obtained MgO-coated SBA-15 support displayed significantly larger medium basic sites and higher Ni dispersion than the MgO-impregnated SBA-15 counterpart, promising enhanced coke-resistant property and better catalytic stability. As displayed in Figure 11, there was almost no change for the conversions of CO2 and CH4 over Ni/8MgO-SBA-15 after a 40-h stability test, displaying much excellent stability. Bach et al. (2020) prepared the Ni/Al2O3 catalyst doped with the MgO promoter (3, 5, and 10 wt.%), performing an effect toward CRM. It was found that MgO could greatly improve the dispersion and metal area of the metallic Ni active site. The Ni/5MgO-Al2O3 catalyst performs the best anti-coke property and highest H2/CO ratio among these catalysts. Singha et al. (2016) compared the catalytic performances of CeO2- and MgO-promoted Ni-ZnO2 catalysts for CRM. The Ni-ZnO2 catalyst promoted by MgO showed better low-temperature activity and coke resistivity than the Ni-ZnO2 catalyst promoted by CeO2. The high alkalinity of MgO and the excellent redox performance of CeO2 increased the dispersion of Ni and formed a strong metal–support interaction, thereby effectively reducing coking on the catalyst surface. Xu et al. (2013) prepared a series of Ni-based catalysts with ordered mesoporous MgO–Al2O3 composite oxides with different Mg contents as the support for CRM reaction. They found that the MgO basic site was beneficial to enhancing the chemisorption and activation of CO2 and improving the catalytic activity of the catalyst.
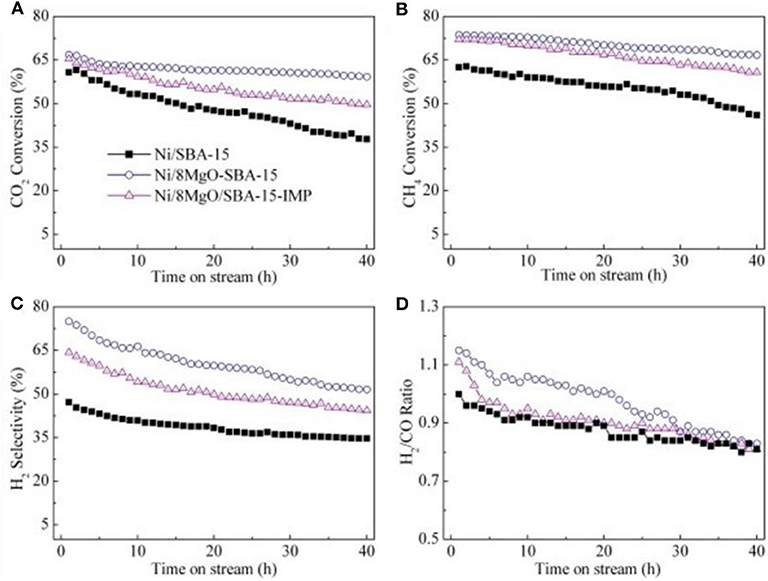
Figure 11. The results of stability test over the samples: (A) CO2 conversion, (B) CH4 conversion, (C) H2 selectivity, and (D) H2/CO ratio [conditions: GHSV = 36,000 mL/(h·gcat), CO2/CH4 = 1:1, T = 700°C, P = 0.1 MPa]. Reproduced from Wang et al. (2013) with permission from International Journal of Hydrogen Energy.
As for CaO, its doping can also improve the chemisorption of CO2 and thereby promote the catalytic stability by inhibiting the surface carbon deposition (Xu et al., 2012b). Xu et al. (2014b) synthesized the Ni-based catalyst supported on the ordered mesoporous CaO-Al2O3 and investigated its catalytic property toward CRM reaction. It could be observed in Figure 12 that the intensity of the CO2 desorption peak of OMA-3Ca was stronger than those of NPA-3Ca and OMA because of the addition of Ca, which enhanced the basicity of the OMA-3Ca framework and the number of Lewis basic sites. Xu et al. (2017) fabricated the MgO and CaO promoted ordered mesoporous Co–Ni–MO(=Mg, Ca)–Al2O3 catalysts. As shown in Figure 13, OMA-5Co5Ni3Mg and OMA-5Co5Ni3Ca displayed much higher CH4 and CO2 conversions than the reference OMA-5Co5Ni in the range of 500–800°C. This indicated that MgO and CaO basic modifiers could achieve the activation of CO2 and CH4 at low temperatures. Xu et al. (2012b) synthesized a series of three-compound NiO-CaO-Al2O3 catalysts with ordered mesoporous structures with different Ca contents. They found that the modification of CaO in the mesoporous framework was also conducive to improving catalytic performance and inhibiting coke deposition by enhancing CO2 chemisorption activation. Sun et al. (2020) prepared a series of Ni-Ca-HMS catalysts and investigated them as the catalysts in CRM reaction. Compared with the pristine Ni catalyst, Ca-promoted catalysts exhibited a better coke-resistant property because of their sufficient basic sites and strong metal–support interactions. In addition, the modification of CaO was beneficial to reducing the degree of carbon graphitization, thereby preventing the deposition of coke.
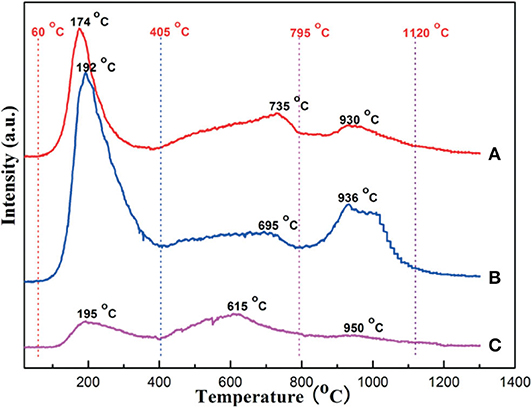
Figure 12. CO2-TPD profiles of the catalytic carriers: (A) OMA, (B) OMA-3Ca, and (C) NPA-3Ca. Reproduced from Xu et al. (2014b) with permission from Catalysis Science and Technology.
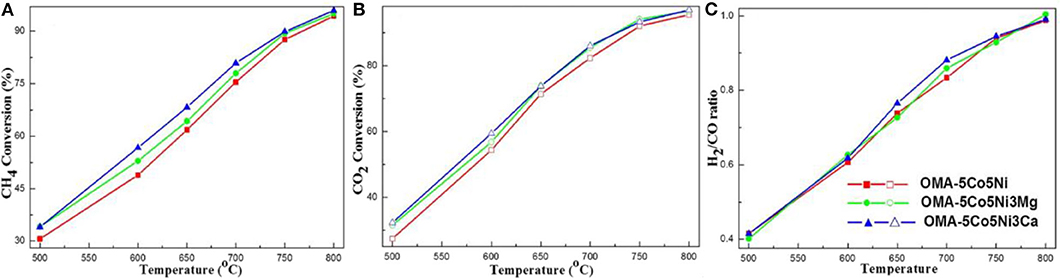
Figure 13. The curves of the (A) CH4 conversion, (B) CO2 conversion, and (C) H2/CO ratio vs. temperature over the catalysts under specific conditions (CH4/CO2 = 1, GHSV = 15,000 mL g−1 h−1, 1 atm). Reproduced from Xu et al. (2017) with permission from Journal of CO2 Utilization.
Rare-Earth Metal Oxides
The rare-earth metal oxides have been widely considered as the effective additives for the Ni-based catalysts toward CRM reaction (Liang et al., 2020; Muhammad et al., 2020). Rare-earth metal oxides can promote the adsorption of CO2 by their basic properties, provide large specific surface areas, and increase catalytic efficiency by increasing surface oxygen vacancies and accelerating charge separation (Tahir et al., 2018).
CeO2 is widely used as the promoter for Ni-based catalysts for CRM reaction because of its strong bonding interaction with metallic active site, excellent reduction–oxidation potential (Ce4+/Ce3+), high oxygen capacity, and abundant activated oxygen species (Tu et al., 2019). Besides, the doping of CeO2 could also increase the surface alkalinity of the catalyst, which is conducive to the chemisorption and activation capacity of the reactants, especially CO2 (Wang et al., 2020). Akbari et al. (2018) investigated the effect of the Ce loading amount on the catalytic performances of Ni-MgO-Al2O3 (12.5 wt.% Ni) catalysts by co-precipitation and impregnation methods toward CRM reaction. The CeO2-Ni-MgO-Al2O3 catalyst exhibited much higher activity, better stability, and more excellent anti-coking performance than the Ni-MgO-Al2O3 catalyst because of the improvement of Ni dispersion and the metal–support interaction by CeO2 promotion. Wang et al. (2020) prepared Ni-based activated carbon (NiCexC) catalysts promoted by Ce with different molar contents and investigated the catalytic performance of CRM in non-thermal plasma (NTP) fixed-bed reactors. Among them, the NiCe1C catalyst performed the best catalytic activities and highest product selectivity because the addition of CeO2 could form the strong metal–support interaction between Ni and CeO2. As a result, the thermal growth of the Ni particles could be effectively inhibited and the activation energy of CH4 was reduced due to the size effect. Li and Sibudjing (2018) compared the catalytic performance of multi-Ni core@NiPhy@CeO2 shell hollow spheres and Ni@NiPhy without coating of the CeO2 shell in CRM reaction. The results showed that Ni@NiPhy@CeO2 performed high sintering resistance to both Ni and CeO2, promising a high concentration of oxygen vacancies. Thus, it had better catalytic performance and coke resistance to CRM reactions. Li et al. (2011) prepared a CeO2-promoted Ni/Al2O3-ZrO2 catalyst by direct sol–gel method. They found that the Ni/Al2O3-ZrO2-CeO2 catalyst showed better activity and stability than the Ni/Al2O3 catalyst because the addition of CeO2 effectively improved the dispersion and stability of the Ni particles of the prepared catalyst and enhanced the adsorption of CO2 on the catalyst surface.
Recently, the studies revealed that La2O3 also possessed high oxygen storage capacity and could enhance the metal–support interaction of the catalyst during the CRM process (Wang F. et al., 2016b; Tran et al., 2020). Besides, La2O3 is also well-known as a strong alkaline oxide, which can intensify the catalyst surface alkalinity. As a result, CO2 can be chemisorbed on surface La2O3 to generate oxidized carbonate (La2O2CO3), which contributed to the elimination of the carbon deposition on the surface of the support (Khoja et al., 2020). Mo et al. (2019) prepared La2O3-NiO-Al2O3 catalysts promoted by La2O3 with different loading amounts to improve the catalytic performance toward CRM reaction. It was found that the doping of La2O3 could effectively promote the dispersion of metallic Ni and the carbon deposition over the catalyst surface was also further inhibited, accounting for the improved catalytic activity and stability. Abdulrasheed et al. (2020) prepared a Ni–La bimetallic catalyst supported on silica fiber KCC-1 and tested its catalytic activity in CRM. As shown in Figure 14, Ni-La@KCC-1 had higher CH4 and CO2 conversion rates than single metal Ni or La reference catalysts. The reducibility of NiO crystallites and the dispersion in the fibrous KCC-1 framework was enhanced because of the addition of La2O3. Thus, more exposed metallic Ni° active sites appeared over the catalyst surface.
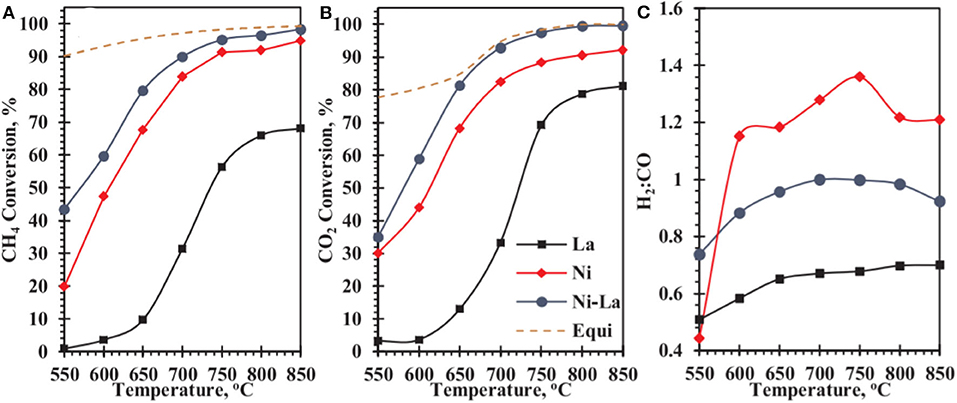
Figure 14. Catalytic conversions of (A) CH4, (B) CO2, and (C) product H2:CO ratio of catalysts as a function of reaction temperature. Reproduced from Abdulrasheed et al. (2020) with permission from Journal of CO2 Utilization.
In addition to this, many other rare-earth elements are also used as promoters of the Ni-based catalysts for CRM reactions. Al-Fatesh et al. (2019b) synthesized a series of Gd-promoted Ni-based catalysts supported on MCM-41 and tested their catalytic activity for DRM reactions. It was found that Gd not only promoted catalytic activity but also improved the stability of the catalyst. Zhang M. et al. (2020) changed the surface adsorbed oxygen species (SAOS) of the Ni/ZrO2 catalyst by doping rare-earth metals such as Ce, La, Sm, and Y. It was proved that the doping of rare-earth metals was beneficial to increasing the amount of SAOS, the Ni dispersion, and the metal–support interaction. The amount of SAOS strongly affected CO2 activation and CH4 dissociation. Xu et al. (2014a) fabricated a series of functionalized mesoporous Ni–Ln (Ln = Ce, La, Sm, Pr)–Al–O composite oxides. They found that the modification of the rare-earth elements (Ce, La, Sm, Pr) in the mesoporous frameworks could greatly improve the catalytic activity of the catalyst and inhibit coke deposition. They also confirmed that the rare-earth modifiers could affect the distribution of carbon species deposited on the catalyst.
Bimetallic Catalysts
The Ni-based bimetallic catalysts have been widely investigated as the catalysts for CRM reaction because of the synergistic effect between Ni and the second metal (Xu et al., 2016a). They are usually prepared by introducing noble or non-noble metals as the second metal. The bimetallic catalyst strategy can not only effectively overcome the drawback of the rapid deactivation caused by carbon deposition over the Ni-based catalyst but also reduce the cost of the catalyst (Niu et al., 2020). The electronic effect produced by metal–metal interaction reduces the sensitivity of the material to carbon deposition (Stroud et al., 2018). Bimetallic catalysts can also increase the dispersion of metallic active sites and reduce the size of metal particles, promising excellent catalytic activity and stability (Erdogan et al., 2018). The Ni-based catalyst is easy to form surface coke during the process of CRM reaction, which causes the decrease of catalyst activity. Therefore, the employment of bimetal or even tri-metal catalysts has been considered as one of the effective methods to improve the coke-resistant capacity of Ni-based catalysts for CRM reaction (Jawad et al., 2019).
It is reported that the addition of the noble metals into Ni-based catalysts can obviously improve the catalytic activity and stability of the catalyst owing to the synergistic effect of active bimetallic catalysts (Niu et al., 2020; Araiza et al., in press). For instance, the strong Ni–Ru interaction of the Ni–Ru bimetallic catalyst could enhance the resistance to carbon deposition because the presence of the Ru was conducive to the gasification of coke and prevented the dissociation of CO (Álvarez et al., 2018). Zhou et al. (2018) confirmed that Ru could accelerate CO2 oxidation of surface carbon, enhance the surface oxygen coverage, and slow down the dissociation of CH4 during the process of the Ni-MgO-catalyzed CRM, which determined the rate of carbon deposition. For Ni–In bimetal catalysts, Németh et al. (2019) found that the presence of In could change the surface structure of the adsorption site and thus inhibit the complete decomposition of CH4 because of the electronic effect on Ni, resulting in the strong chemisorption of H2. Therefore, the Ni–In/SiO2 catalyst performed much better anti-coking performance than the Ni/SiO2 reference catalyst. Pan et al. (2020) synthesized mesoporous silica-supported the bimetallic Ni–Pd catalyst for CRM reaction. The results revealed that the presence of Pd was beneficial to increasing the ratio of the surface-active Ni° of the catalyst. In addition, the doping of Pd and oleic acid was conducive to the formation of small Ni nanoparticles with good dispersion and further inhibiting the thermal sintering.
In recent years, the Ni–Co bimetallic catalysts have attracted more and more attention because of their outstanding catalytic performances. In the Ni–Co bimetal catalyst, the addition of Co can tune the size of Ni and improve the gasification of coke by enhancing CO2 chemisorption, which could reduce the coke yield and further increase the activity and stability of the catalyst (Movasati et al., 2019; Liu et al., in press). Previous reports also found that the Ni/Co atomic ratio is a key factor affecting the catalytic performance and only the appropriate Co/Ni ratio has a synergistic Ni–Co interaction, which improved the catalytic activity and stability of the catalyst (Xu et al., 2016a; Pang et al., 2018). Turap et al. (2020) investigated the effect of Co content on the performance of bimetallic Ni-Co/CeO2 catalysts. Their results showed that the catalytic activity over the 0.8Co–Ni/CeO2 catalyst was much higher than that over the Ni/CeO2 reference catalyst toward CRM reaction. This was attributed to the strong adsorption of oxygen over the Ni–Co alloy and the redox property of CeO2 support, which could directly break the carbon–oxygen bond and inhibit the occurrence of the RWGS reaction. Erdogan et al. (2018) compared the activities of Ni-, Co-, and Ni–Co-based catalysts supported on the SBA-15 toward CRM reaction. The 4Ni-1Co@SBA-15 catalyst performed the highest CO and H2 selectivity among these catalysts, indicating that the incorporation of Co had a significant effect on the catalytic activity of the Ni catalyst. The catalytic activity of the 4Ni-1Co@SBA-15 bimetallic catalyst was better than those of the Ni and Co single metal catalysts because of the formation of Ni–Co alloy. Xu et al. (2016a) prepared a series of Co–Ni bimetallic catalyst-doped ordered mesoporous Al2O3 composites oxides. Due to the synergistic effect of Co–Ni, ordered mesoporous Co–Ni bimetallic catalysts exhibited higher catalytic activity and better coking resistance than the corresponding Ni and Co monometallic counterpart catalysts. The specific synergistic effect mechanism in the Co–Ni bimetallic catalyst was illustrated in Figure 15.
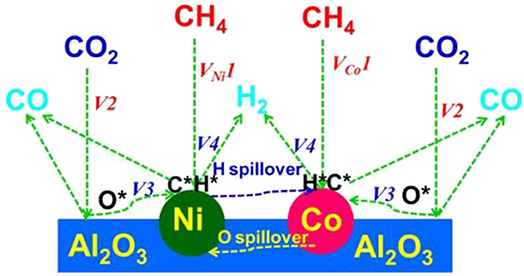
Figure 15. The synergistic effect mechanism in the Co–Ni bimetallic catalyst. Reproduced from Xu et al. (2016a) with permission from ChemCatChem.
It was reported that the Ni–Sn interaction is essential to the achievement of the good catalytic activity (Stroud et al., 2018). This is because Sn atom has an electronic structure similar to C atom. This will facilitate the interaction between the p orbital of Sn and the 3d electron of Ni and thereby slow down the formation of coke (Guharoy et al., 2018). In addition to this, Sn can also improve the dispersion of the metallic Ni and promote the oxidation of key reaction intermediates on the surface of the catalyst. Thereby, the formation of the final product will be facilitated (Bobadilla et al., 2016; Guharoy et al., 2018). Stroud et al. (2018) found that the addition of Sn could greatly promote the catalytic activities and stabilities over the investigated Ni-Sn/Al2O3 and Ni-Sn/CeO2-Al2O3 catalysts toward CRM reaction. As a result, the process of the coke formation was slowed down because the Sn atom could occupy the neighboring C-shaped nucleus site on the Ni atom. However, an excessive amount of Sn would exert the adverse effect on the catalytic performance, owing to the coverage of the Ni active site, which limited the access of the gaseous reactants and thereby reduce the conversion of reactants.
In general, adding alkali metal and alkaline earth metal oxides can increase the surface basicity of the catalyst and thus increase the amount of CO2 adsorbed on the catalyst surface and improve the carbon removal effect of CO2. The addition of La2O3 and CeO2 mainly enhances the ability of oxygen adsorption and dissociation, inhibit the generation of carbon deposits, and improve the antioxidant capacity of the active component Ni. Therefore, choosing appropriate additives plays a vital role in the catalytic performance of the Ni-based catalyst. Bimetallic catalysts exhibit unique properties and excellent catalytic performance, which are different from single metal catalysts due to their adjustable physical and chemical properties, electronic, and geometric effects between bimetals. Although the bimetallic catalysts with noble metals have good catalytic activity and selectivity, the non-metallic doped bimetallic catalysts have a wider application prospect because of the advantage of cost.
Preparation Method
The preparation method plays a crucial role in affecting the performances of the catalysts toward the CRM reaction. This is because it can affect the composition of the catalyst, particle size, dispersion of the active sites, and surface interaction between catalyst components (Hassani Rad et al., 2016). These properties could greatly affect the catalytic performances of CRM at high temperature by enhancing their anti-sintering and anti-coking properties (Hambali et al., 2020; Shah et al., 2020). Generally, impregnation method (Zou et al., 2017), sol–gel method (Aghamohammadi et al., 2017), and co-precipitation method (Zhang G. et al., 2019) are common methods for synthesizing the Ni-based catalysts. Specifically, the impregnation method usually puts the support into the solution with dissolved active components, and the solution enters the pore channels of the support by capillary pressure (Romero-Sáez et al., 2018). The impregnation method is easy to operate and control the dispersion of the metallic active sites on the support. However, it can only achieve the small loading amount because of the limitation of the saturation solubility of the metal precursor and the metal–support interaction between the Ni nanoparticles and the support is weak (Arbag, 2018). It was reported that the impregnation sequence of metal precursors could greatly affect the metal-support interaction over the polymetallic catalysts prepared by impregnation, which further had a certain effect on the performance of the catalyst (Zou et al., 2017). Romero-Sáez et al. (2018) prepared the Ni-ZrO2 catalysts supported on CNT by the sequential impregnation and co-impregnation methods. It was found that the NiO nanoparticles were wrapped by ZrO2 in the core–shell structure over the catalyst prepared by co-impregnation method and the NiO nanoparticles were on or near the surface of ZrO2 over the catalyst prepared by the sequential impregnation method. Arbag (2018) reported that the impregnation sequence of Mg had a great influence on the activity of Ni-based catalysts supported on mesoporous alumina. The catalyst subsequently impregnated with Mg and Ni could achieve the highest coking rate. As a comparison, the catalyst synthesized by co-impregnation of Mg and Ni almost behaved no coking phenomenon because of the strong interaction between Ni and Mg by forming NiO–MgO solid solution at high calcination temperature.
In case of the sol–gel method, it can quickly achieve molecular homogeneity of the active sites because they can be individually mixed on the molecular level during the formation of gel (Tao et al., 2013). During the sol–gel process, it is easy to achieve the uniform doping of the active ingredients in molecular level at low synthesis temperature (Aghamohammadi et al., 2017). However, the sol–gel process usually takes a long time and a large amount of gas and organic compounds will be released during the drying process (Lv et al., 2020). The catalysts prepared by the sol–gel method possess the advantages of narrow particle size distribution, high resistant property to thermal agglomeration, and low deactivation rate (Hassani Rad et al., 2016). Aghamohammadi et al. (2017) prepared a Ni/Al2O3-CeO2 nanocatalyst by the sol–gel method. As shown in Figure 16, the NAC-SG catalyst displayed much higher feed conversion, product yield, and H2/CO ratio than NAC-I catalyst. Marinho et al. (2020) found the positive effect of the sol–gel method on the stabilization of Ni particle size in the reduction process at high temperature (800°C) Ni@CeO2. Besides, the above catalysts prepared by the sol–gel method also generated more oxygen vacancies and stronger metal-support interaction with CeZrO2 and Ni than the impregnated Ni/CeO2 catalyst. Shin et al. (2018) reported that the Ni/ZrO2-Al2O3 catalyst prepared by Pechini sol–gel method showed higher stability than those synthesized by urea hydrolysis and physical mixing methods due to the strong metal–support interaction between Ni and ZrO2-Al2O3, which further promoted the dissociation of CO2.
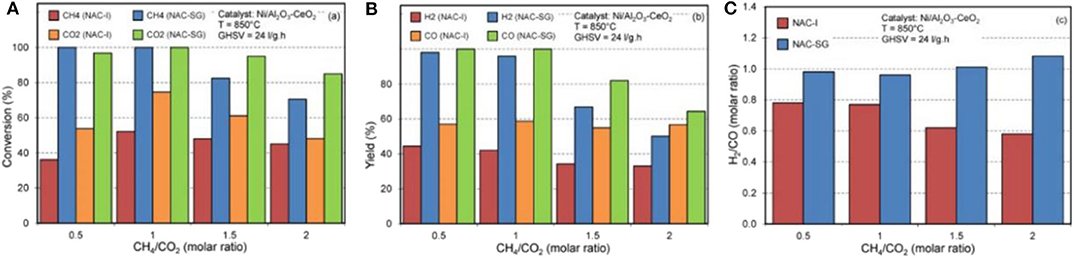
Figure 16. Effect of synthesis method on (A) feed conversion, (B) products yield, and (C) H2/CO molar ratio in a product at different feed ratios over Ni/Al2O3-CeO2 nanocatalyst. Reproduced from Aghamohammadi et al. (2017) with permission from Molecular Catalysis.
As for the co-precipitation method, it usually has many advantages, such as simple preparation process, low cost, easy preparation control, and short synthesis time. Therefore, it has been one of the most widely investigated preparation methods (Pejjai et al., 2020). However, the agglomeration or uneven composition usually takes place because the addition of the precipitant may make part of the concentration too dense (Marzano et al., 2019). Therefore, how to improve the activity and the resistance to carbon deposition over the Ni-based catalyst by employing unconventional preparation methods is still a challenge. Gurav et al. (2017) prepared the Gd-doped Ni-based catalyst supported on CeO2 by the co-precipitation method, citrate gel method, and impregnation method. As could be observed in Figure 17 12NGDC-CP performed the highest conversion rates of CH4 and CO2 among these three catalysts because of the highest metal dispersion. Therefore, the preparation method can obviously affect the metal dispersion and then influence the catalytic activity.
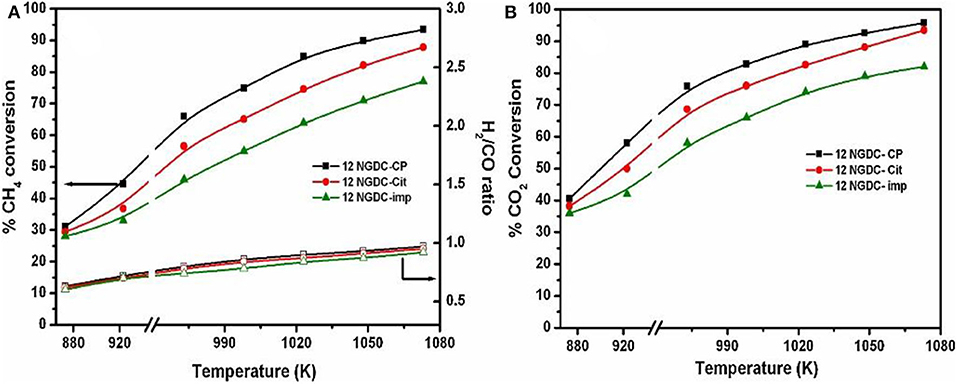
Figure 17. Effect of temperature on various 12NGDC catalysts, (A) CH4 conversion and H2/CO ratio and (B) CO2 conversion. Reaction conditions: CH4:CO2:N2 = 1:1:1, GHSV = 28,800 h−1 (filled symbols-conversion, hollow symbols-H2/CO ratios). Reproduced from Gurav et al. (2017) with permission from Journal of CO2 Utilization.
In recent years, the plasma method has attracted more and more attention because of its unique advantages, such as compact structure, strong material adaptability, and fast response (Snoeckx et al., 2015; Biset-Peir et al., 2020). Among the plasma techniques, the low-temperature plasma is commonly used for the preparation of the Ni-based catalysts toward CRM reaction. The advantage of the low-temperature plasma is that it can activate the gas through electron collisions to stimulate ionization and dissociation reactions rather than heat the entire reactor (Chai and Kwon, 2019). It was reported that the dielectric barrier discharge plasma (DBD) decomposition is an excellent method of preparing Ni-based catalysts with improved activity toward CRM reaction (Hu et al., 2019). Hu et al. (2019) investigated the Ni/ZrO2 catalysts prepared by traditional calcination (NiZrO2-C) and plasma (Ni/ZrO2-P) methods for the CRM reaction. Compared with NiZrO2-C, the Ni/ZrO2-P catalyst displayed more Ni(111) planes, smaller metallic Ni particles, and more oxygen vacancies. As a result, the Ni/ZrO2-P catalyst displayed better catalytic activity than Ni/ZrO2-C toward CRM reaction. Fang et al. (2016) investigated the catalytic activity of Ni/Y2Zr2O7 catalysts treated by DBD toward CRM reaction. They found that plasma treatment could enhance the metal–support interaction of Ni/Ni-Y2Zr2O7, generate small NiO nanoparticles, and produce a large metallic Ni active surface area. Thereby, the Ni/Y2Zr2O7 catalyst with the highest activity, the best stability, and the strongest coke resistance can be obtained by plasma treatment in H2/Ar mixture gas stream.
Some other catalyst preparing methods, such as homogeneous deposition (Park et al., 2019), combustion synthesis (Gonzalez-Delacruz et al., 2010), reflux digestion (Chen et al., 2013), and atomic layer deposition (ALD) (Zhao et al., 2018), are also investigated to synthesize the Ni-based catalysts for CRM reactions. As for the homogeneous precipitation method, the choice of precipitants has a great impact on the catalytic performance of the catalyst. Zhang G. et al. (2019) used alkaline precipitants to obtain Ni3Si2O5(OH)4 nanosheets in the catalyst precursor. Among them, the strong electrolytic capacity of NaOH made the most Ni3Si2O5(OH)4 nanoflakes formed in the Ni-MSC-1 catalyst, which results in the highest Ni dispersity and highest catalytic activity. Park et al. (2019) studied the effect of preparation methods on the catalytic performance of Ni-substituted CaZrOx catalysts toward CRM reaction. Compared with Ni/CaZrOx prepared by the impregnation method, CaZrNiOx prepared by the homogeneous deposition method behaved in a larger number of alkaline sites, higher surface area, better Ni dispersion, and stronger metal–support interaction. Therefore, CaZrNiOx demonstrated better catalytic activity and stability during the CRM reaction. Gonzalez-Delacruz et al. (2010) prepared the Ni-CeO2 catalyst effectively under various metal loadings through a combustion synthesis method, which was conducive to controlling the final stoichiometry and quantity production of catalysts without complicated equipment, separation process, and expensive precursors. Chen et al. (2013) prepared the Ni-CaO-ZrO2 nanocomposite catalyst by the co-precipitation method and reflux-digestion method. They found that reflux digestion conditions had a significant effect on the catalyst structure, crystal phase, and catalytic performance. Higher reflow temperature and longer reflow time were beneficial to the formation of smaller particles. The ALD method is widely known for its ability to form films with high uniformity, consistency, repeatability, and thickness accuracy at the atomic level (Shang et al., 2017). Zhao et al. (2018) developed a novel Al2O3/Ni/Al2O3-sandwiched catalyst by the ALD method. They found that the dual interactions of Ni with γ-Al2O3 support and Al2O3 film could effectively inhibit Ni aggregation at high temperature and subsequent carbon deposition. The catalyst showed excellent catalytic activity and stability in CRM reaction and displayed good industrial application potential.
In general, each preparation method has its own advantages in the process of preparing the catalyst and the performance of the prepared catalyst. Even for the same active component, the difference in preparation method will affect the reducing particle size dispersion of the active component and the microstructure of the catalyst, which will cause the significant difference in the reaction activity and selectivity of the catalyst, as well as the significant difference in the anti-carbon deposition ability. Therefore, the catalytic performance of the catalyst can be tuned by controlling the difference in the preparation method. Meanwhile, the new preparation method provides more possibilities for improving the catalytic performance of the Ni-based catalyst toward CRM reaction.
The Mechanism of CRM
With the recent development and wide application of the experimental characterization, the mechanism of DRM reaction has been extensively investigated. Abdulrasheed et al. (2019) illustrated the scheme of the CRM mechanism of CRM. It can be generally summarized into the following steps: dissociative adsorption of CH4, dissociative adsorption of CO2, hydroxyl group formation, intermediate oxidation, and desorption, as shown in Figure 18.
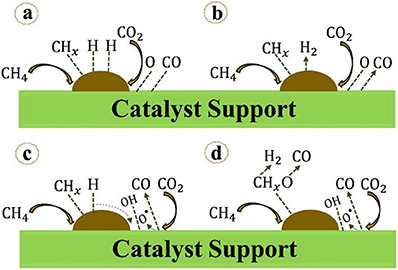
Figure 18. Mechanistic steps for methane reforming reaction involving: (A) chemisorption and dissociation of CH4 and CO2 on metal-support interface, (B) fast rate of H2 and CO desorption, (C) hydrogen and oxygen spillover forming surface hydroxyls, (D) oxidation of hydrogen-depleted CHx species by surface hydroxyls and oxygen groups to produce H2 and CO. Reproduced from Abdulrasheed et al. (2019) with permission from Renewable and Sustainable Energy Reviews.
The CRM reaction process is accompanied by multiple side reactions. Various catalytic systems and process conditions will possess different catalytic mechanisms. So far, the global scholars generally recognize that the process of CH4 conversion over the metallic active site mainly contains the following steps: (1) the gradual decomposition and dehydrogenation of CH4 to (0 < x <4) and H*, (2) the recombination of H* to generate H2, and (3) the desorption of the catalyst surface at high temperature (Zhou et al., 2018). However, the viewpoints of the CO2 conversion mechanism are controversy between different researchers. Some researchers believe that CO2 is chemisorbed on the metallic active site or catalytic support to form the carbonate. Subsequently, the activated carbonate further reacts with to generate CO and H2 (Wang et al., 2014; Foppa et al., 2016). Another viewpoint of the CO2 activation is that CO2 can be directly dissociated into CO and O* on the metallic active site of the catalyst. Then, O* reacts with to generate CO and H* (Zhu et al., 2009).
Chong et al. (2020) reported the mechanism of syngas production through CRM of 10Ni/DFSBA-15 as shown in Figure 19. CO2 tended to dissociate into CO* and O* or adsorb on the catalyst surface and then interact with the basic oxygen atoms on the surface to form intermediate species, such as unidentate carbonate, bidentate carbonate, and linear carbonyls, during the CRM reaction over the 10Ni/DFSBA-15 catalyst. Subsequently, these reaction intermediates further reacted with CH4 molecules to generate H2 and CO. Li K. et al. (2019) found that the interface between Ni and La2O3 promoted the formation of bidentate carbonate and further participated in the coke removal process over the Ni/La2O3 catalyst. Therefore, the reaction mechanisms and scheme of catalyst models was assumed as shown in Figure 20. The activation and decomposition of CH4 occurred on the Ni particle surface to generate activated coke precursors and H2. CO2 was adsorbed on the interface between Ni and La2O3 to forms bidentate carbonate. Bidentate carbonate reacted with adjacent activated coke precursors to form CO. During this process, it was observed that the strength of bidentate carbonate gradually decreased while the strength of monodentate carbonate remained stable. This indicates that the bidentate carbonate could react effectively with the coke intermediate. Moreover, it was found that the activation of methane and the formation of CO took place at the same time, demonstrating that CO2 participated in the coke-elimination reaction pathway and reacted with carbon species to inhibit coke deposition. They also found that the addition of Ni promoted the formation of bidentate carbonate on the La2O3 catalyst and the 5Ni/La2O3-m catalyst performed a strong promotion effect on the formation of bidentate carbonate intermediate. Bu et al. (2020) prepared the Ni/d-BN with defect confinement toward CRM reaction. The mechanism for the DRM reaction for Ni/d-BN was displayed in Figure 21. Firstly, CH4 and CO2 dissociate into CHx*, H*, O*, and CO* and other active species at the defect sites of d-BN and the interface between Ni and d-BN. Subsequently, the presence of H* can further facilitate the activation of CO2 and assist its conversion into –HCO2. Then,–HCO2 will be decomposed into –HCO and O*. Besides, H* and O* can easily combine into –OH at the B terminal of d-BN, and –OH can assist conversion into formyl (–HCO) species, and –HCO can rapidly decompose into CO and H*. Because of the presence of H* and O* in the reaction, even if –OH is consumed by CHx*, H* and O* can rapidly bind to B terminal sites to reform B–OH species. Kim et al. (2019) studied the catalytic performance of the reduced Co- and Mn-modified perovskite LaNiO3 catalyst toward CRM reaction. They found that CO2 could chemisorb on the La2O3 support and then generate the oxygen atom. CH4 could be dehydrogenated on the surface of metallic Ni (or Co) sites to generate carbon atom and hydrogen atom. Finally, the oxygen atom reacted with the carbon atom to produce CO. Furthermore, it could be observed in Figure 22 that the formation of coke was confirmed. The metallic active nanoparticles were separated from La2O2CO3 by inhibiting the oxygen supply from the filamentous carbon chains to the metal surface. The metal nanoparticles were kept in close contact with the supporting site to obtain a stable oxygen supply and CO generation. When a large amount of coke was formed, the metal particles were removed from the scaffold by filamentous carbon. As a result, there was no oxygen supply from La2O3. The carbon atoms formed on the metal surface could not be effectively eliminated and the polymerization of the carbon atoms accelerated the coking process. Therefore, the close contact between the metallic active site and the support had an important impact on the high activity and stability of the CRM catalyst because the CO formation reaction might take place at the metal–support interface. In summary, so far there has not been a single reaction mechanism that can summarize all CRM reactions. However, extensive research on CRM reaction mechanism contributes to a deeper understanding of CRM reaction and then design of the catalyst with better catalytic activity.
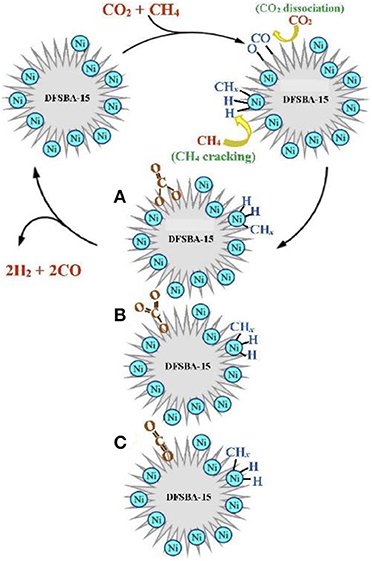
Figure 19. Reaction scheme of Ni/DFSBA-15 over CRM. (A) Bidentate carbonate, (B) unidentate carbonate, and (C) linear carbonyl. Reproduced from Chong et al. (2020) with permission from International Journal of Hydrogen Energy.
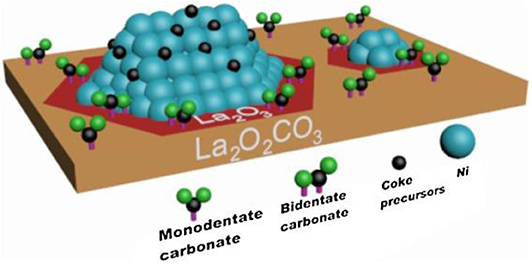
Figure 20. Proposed Ni/La2O3 catalyst model in the DRM process. Reproduced from Li K. et al. (2019) with permission from Applied Catalysis B: Environmental.
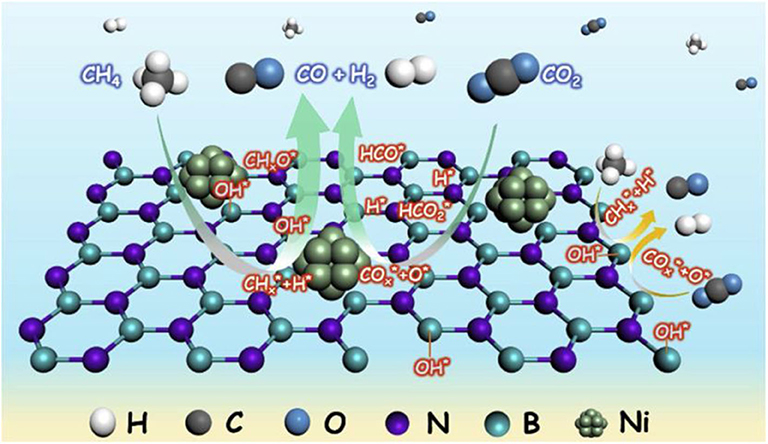
Figure 21. Possible reaction mechanism over Ni/d-BN catalysts. Reproduced from Bu et al. (2020) with permission from Applied Catalysis B: Environmental.
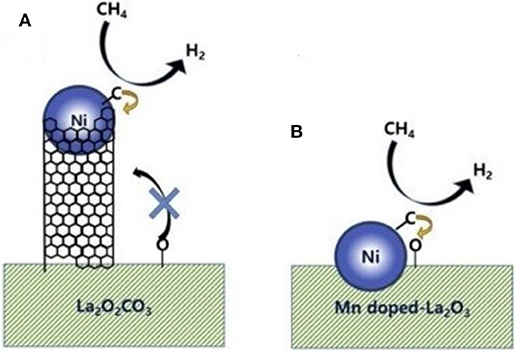
Figure 22. A schematic model of catalyst deactivation by coking. (A) Active metal nanoparticles depart from La2O2CO3 support by filamentous carbon growth that blocked oxygen supply to the metal surface. (B) The metal nanoparticles maintain close contact with the support to receive steady oxygen supply to produce CO. Reproduced from Kim et al. (2019) with permission from Applied Catalysis A: General.
Conclusions and Perspective
CO2 has been considered as one of the major incentives of many environmental problems, such as global warming and extreme weather. CRM not only effectively utilizes the CO2 resource and reduce carbon emissions but also produces the value-added syngas, which can be further employed as the building unit of the synthesis of alcohols, olefins, and other valuable products. For now, the main challenge of the CRM reaction is to develop a highly efficient catalyst with high catalytic activity, sinter proof, coke resistance, and low cost.
Ni-based catalysts have been widely regarded as promising alternatives to noble metal catalysts. However, their industrial application is limited due to the serious sintering of Ni nanoparticles and rapid catalyst deactivation caused by coke deposition. Various strategies of improving the performance of catalysts are carefully and comprehensively summarized in this review. In order to reduce carbon deposition on Ni-based catalysts, researchers have tried to add promoters to change the alkalinity of the support, such as alkaline earth metal oxides and rare-earth metal oxides, which can enhance the stability and decrease carbon formation. It is found that the catalytic performances can be affected by the preparation methods. Besides, the choice of the support is also very crucial. Various materials, such as metal oxides, ordered mesoporous silica gel materials, zeolite materials, and carbon materials, can be used as the supports of the Ni-based catalysts.
In future research, it can be prospected that the research hotspot of Ni-based catalysts in the field of CRM will focus on the Ni-based catalysts supported or confined by novel materials, such as ordered mesoporous materials and hollow zeolites. Specifically, the mesoporous framework in these catalysts facilitates the dispersion of active sites, and the confinement effect of mesoporous channels or hollow cavity can effectively control the size of Ni nanoparticles during CRM reactions. Besides, the combination of CRM with other reactions, such as SRM and POM, can alter the H2/CO ratio of syngas by changing the feedstock gas ratio, which will provide different approaches for large-scale industrial applications of CRM.
Author Contributions
LX conceived of the presented idea. LX encouraged XWu to investigate the project and supervised the whole progress of this work. CL, YC, XWen, CW, BY, and ZM assisted XWu to carry out the literature survey and summary. XWu, LX, and MC wrote the manuscript with support from XH. LX, MC, and XH supervised the whole project. All authors discussed the results and contributed to the final manuscript.
Funding
The authors sincerely acknowledge the financial support from the National Natural Science Foundation of China (Grant Nos. 21503113, 21577065, and 21976094), the National Key Research and Development Project (Grant No. 2018YFC0213802), Environmental Protection Projects of Jiangsu Province (2017022), and a Project Funded by the Priority Academic Program Development of Jiangsu Higher Education Institutions. This study was also supported by Startup Foundation of Nanjing University of Information Science and Technology.
Conflict of Interest
The authors declare that the research was conducted in the absence of any commercial or financial relationships that could be construed as a potential conflict of interest.
References
Abdullah, B., Abd Ghani, N. A., and Vo, D.-V. N. (2017). Recent advances in dry reforming of methane over Ni-based catalysts. J. Clean. Prod. 162, 170–185. doi: 10.1016/j.jclepro.2017.05.176
Abdullah, N., Ainirazali, N., Chong, C. C., Razak, H. A., Setiabudi, H. D., Jalil, A. A., et al. (2019). Influence of impregnation assisted methods of Ni/SBA-15 for production of hydrogen via dry reforming of methane. Int. J. Hydrog. Energy 45:18426–18439. doi: 10.1016/j.ijhydene.2019.09.089
Abdulrasheed, A., Jalil, A. A., Gambo, Y., Ibrahim, M., Hambali, H. U., and Shahul Hamid, M. Y. (2019). A review on catalyst development for dry reforming of methane to syngas: recent advances. Renew. Sustain. Energy Rev. 108, 175–193. doi: 10.1016/j.rser.2019.03.054
Abdulrasheed, A. A., Jalil, A. A., Hamid, M. Y. S., Siang, T. J., and Abdullah, T. A. T. (2020). Dry reforming of CH4 over stabilized Ni-La@KCC-1 catalyst: Effects of La promoter and optimization studies using RSM. J. CO2 Util. 37, 230-239. doi: 10.1016/j.jcou.2019.12.018
Aghamohammadi, S., Haghighi, M., Maleki, M., and Rahemi, N. (2017). Sequential impregnation vs. sol-gel synthesized Ni/Al2O3-CeO2 nanocatalyst for dry reforming of methane: effect of synthesis method and support promotion. Mol. Catal. 431, 39–48. doi: 10.1016/j.mcat.2017.01.012
Akbari, E., Alavi, S. M., and Rezaei, M. (2018). CeO2 promoted Ni-MgO-Al2O3 nanocatalysts for carbon dioxide reforming of methane. J. CO2 Util. 24, 128–138. doi: 10.1016/j.jcou.2017.12.015
Al-Fatesh, A. S., Arafat, Y., Ibrahim, A. A., Atia, H., Fakeeha, A. H., Armbruster, U., et al. (2018). Evaluation of Co-Ni/Sc-SBA−15 as a novel coke resistant catalyst for syngas production via CO2 reforming of methane. Appl. Catal. A Gen. 567, 102–111. doi: 10.1016/j.apcata.2018.09.012
Al-Fatesh, A. S., Atia, H., Abu-Dahrieh, J. K., Ibrahim, A. A., Eckelt, R., Armbruster, U., et al. (2019a). Hydrogen production from CH4 dry reforming over Sc promoted Ni/MCM-41. Int. J. Hydrog. Energy 44, 20770–20781. doi: 10.1016/j.ijhydene.2018.07.036
Al-Fatesh, A. S., Hanan, A., Ibrahim, A. A., Fakeeha, A. H., Singh, S. K., Labhsetwar, N. K., et al. (2019b). CO2 reforming of CH4: Effect of Gd as promoter for Ni supported over MCM-41 as catalyst. Renew. Energ. 140, 658–667. doi: 10.1016/j.renene.2019.03.082
Álvarez, A. M., Bobadilla, L. F., Garcilaso, V., Centeno, M. A., and Odriozola, J. A. (2018). CO2 reforming of methane over Ni-Ru supported catalysts: On the nature of active sites by operando DRIFTS study. J. CO2 Util. 24, 509–515. doi: 10.1016/j.jcou.2018.01.027
Anil, C., Modak, J. M., and Madras, G. (2020). Syngas production via CO2 reforming of methane over noble metal (Ru, Pt, and Pd) doped LaAlO3 perovskite catalyst. Mol. Catal. 484:110805. doi: 10.1016/j.mcat.2020.110805
Araiza, D. G., Arcos, D. G., Gómez-Cortés, A., and Díaz, G. (in press). Dry reforming of methane over Pt-Ni/CeO2 catalysts: effect of the metal composition on the stability. Catal. Today. doi: 10.1016/j.cattod.2019.06.018
Arbag, H. (2018). Effect of impregnation sequence of Mg on performance of mesoporous alumina supported Ni catalyst in dry reforming of methane. Int. J. Hydrog. Energy 43, 6561–6574. doi: 10.1016/j.ijhydene.2018.02.063
Ayodele, O. B., and Abdullah, A. Z. (2019). Exploring kaolinite as dry methane reforming catalyst support: Influences of chemical activation, organic ligand functionalization and calcination temperature. Appl. Catal. A Gen. 576, 20–31. doi: 10.1016/j.apcata.2019.02.034
Azancot, L., Bobadilla, L. F., Santos, J. L., Córdoba, J. M., Centeno, M. A., and Odriozola, J. A. (2019). Influence of the preparation method in the metal-support interaction and reducibility of Ni-Mg-Al based catalysts for methane steam reforming. Int. J. Hydrog. Energy 44, 19827–19840. doi: 10.1016/j.ijhydene.2019.05.167
Bach, V. R., de Camargo, A. C., de Souza, T. L., Cardozo-Filho, L., and Alves, H. J. (2020). Dry reforming of methane over Ni/MgO–Al2O3 catalysts: thermodynamic equilibrium analysis and experimental application. Int. J. Hydrog. Energy 45, 5252–5263. doi: 10.1016/j.ijhydene.2019.07.200
Barelli, L., Bidini, G., Di Michele, A., Gammaitoni, L., Mattarelli, M., Mondi, F., et al. (2019). Development and validation of a Ni-based catalyst for carbon dioxide dry reforming of methane process coupled to solid oxide fuel cells. Int. J. Hydrog. Energy 44, 16582–16593. doi: 10.1016/j.ijhydene.2019.04.187
Bawah, A.-R., Malaibari, Z. O., and Muraza, O. (2018). Syngas production from CO2 reforming of methane over Ni supported on hierarchical silicalite-1 fabricated by microwave-assisted hydrothermal synthesis. Int. J. Hydrog. Energy 43, 13177–13189. doi: 10.1016/j.ijhydene.2018.05.073
Biset-Peir,ó, M., Mey, R., Guilera, J., and Andreu, T. (2020). Adiabatic plasma-catalytic reactor configuration: energy efficiency enhancement by plasma and thermal synergies on CO2 methanation. Chem. Eng. J. 393:124786. doi: 10.1016/j.cej.2020.124786
Bobadilla, L. F., Romero-Sarria, F., Centeno, M. A., and Odriozola, J. A. (2016). Promoting effect of Sn on supported Ni catalyst during steam reforming of glycerol. Int. J. Hydrog. Energy 41, 9234–9244. doi: 10.1016/j.ijhydene.2016.04.119
Bu, K., Deng, J., Zhang, X., Kuboon, S., Yan, T., Li, H., et al. (2020). Promotional effects of B-terminated defective edges of Ni/boron nitride catalysts for coking- and sintering-resistant dry reforming of methane. Appl. Catal. B 267:118692. doi: 10.1016/j.apcatb.2020.118692
Bu, K., Kuboon, S., Deng, J., Li, H., Yan, T., Chen, G., et al. (2019). Methane dry reforming over boron nitride interface-confined and LDHs-derived Ni catalysts. Appl. Catal. B 252, 86–97. doi: 10.1016/j.apcatb.2019.04.007
Bukhari, S. N., Chin, C. Y., Setiabudi, H. D., and Vo, D.-V. N. (2017). Tailoring the properties and catalytic activities of Ni/SBA-15 via different TEOS/P123 mass ratios for CO2 reforming of CH4. J. Environ. Chem. Eng. 5, 3122–3128. doi: 10.1016/j.jece.2017.06.012
Capellán-Pérez, I., Mediavilla, M., de Castro, C., Carpintero, Ó., and Miguel, L. J. (2014). Fossil fuel depletion and socio-economic scenarios: an integrated approach. Energy 77, 641–666. doi: 10.1016/j.energy.2014.09.063
Chai, K.-B., and Kwon, D.-H. (2019). Optical emission spectroscopy and collisional-radiative modeling for low temperature Ar plasmas. J. Quant. Spectrosc. Radiat. Transfer 227, 136–144. doi: 10.1016/j.jqsrt.2019.02.015
Chai, R., Fan, S., Zhang, Z., Chen, P., Zhao, G., Liu, Y., et al. (2017a). Free-Standing NiO-MgO-Al2O3 Nanosheets Derived from Layered Double Hydroxides Grown onto FeCrAl-Fiber as Structured Catalysts for Dry Reforming of Methane. ACS Sustain. Chem. Eng. 5, 4517–4522. doi: 10.1021/acssuschemeng.7b00717
Chai, R., Zhao, G., Zhang, Z., Chen, P., Liu, Y., and Lu, Y. (2017b). High sintering-/coke-resistance Ni@SiO2/Al2O3/FeCrAl-fiber catalyst for dry reforming of methane: one-step, macro-to-nano organization via cross-linking molecules. Catal. Sci. Technol. 7, 5500–5504. doi: 10.1039/C7CY01491K
Chen, Q. J., Zhang, J., Jin, Q. W., Pan, B. R., Kong, W. B., Zhao, T. J., et al. (2013). Effect of reflux digestion treatment on the catalytic performance of Ni–CaO–ZrO2 nanocomposite catalysts for CO2 reforming of CH4. Catal. Today 215, 251–259. doi: 10.1016/j.cattod.2013.06.011
Chong, C. C., Cheng, Y. W., Setiabudi, H. D., Ainirazali, N., Vo, D.-V. N., and Abdullah, B. (2020). Dry reforming of methane over Ni/dendritic fibrous SBA-15 (Ni/DFSBA-15): Optimization, mechanism, and regeneration studies. Int. J. Hydrog. Energy 45, 8507–8525. doi: 10.1016/j.ijhydene.2020.01.056
Chong, C. C., Owgi, A. H. K., Ainirazali, N., Chin, S. Y., and Setiabudi, H. D. (2018). CO2 reforming of CH4 over Ni/SBA-15 prepared by surfactant-assisted impregnation method: comparative study of surfactant types. Mater. Today Proc. 5, 21644–21651. doi: 10.1016/j.matpr.2018.07.014
Dai, C., Li, X., Zhang, A., Liu, C., Song, C., and Guo, X. (2015a). Pd and Pd–CuO nanoparticles in hollow silicalite-1 single crystals for enhancing selectivity and activity for the Suzuki–Miyaura reaction. RSC Adv. 5, 40297–40302. doi: 10.1039/C5RA05952F
Dai, C., Zhang, S., Zhang, A., Song, C., Shi, C., and Guo, X. (2015b). Hollow zeolite encapsulated Ni–Pt bimetals for sintering and coking resistant dry reforming of methane. J. Mater. Chem. A 3, 16461–16468. doi: 10.1039/C5TA03565A
Dan, M., Mihet, M., and Lazar, M. D. (2020). Hydrogen and/or syngas production by combined steam and dry reforming of methane on nickel catalysts. Int. J. Hydrog. Energy. 45, 26254–26264 doi: 10.1016/j.ijhydene.2019.12.158
Das, S., Ashok, J., Bian, Z., Dewangan, N., Wai, M. H., Du, Y., et al. (2018). Silica–Ceria sandwiched Ni core–shell catalyst for low temperature dry reforming of biogas: coke resistance and mechanistic insights. Appl. Catal. B 230, 220–236. doi: 10.1016/j.apcatb.2018.02.041
de Dios García, I., Stankiewicz, A., and Nigar, H. (in press). Syngas production via microwave-assisted dry reforming of methane. Catal. Today. doi: 10.1016/j.cattod.2020.04.045
de la Cruz-Flores, V. G., Martinez-Hernandez, A., and Gracia-Pinilla, M. A. (2020). Deactivation of Ni-SiO2 catalysts that are synthetized via a modified direct synthesis method during the dry reforming of methane. Appl. Catal. A Gen. 594:117455. doi: 10.1016/j.apcata.2020.117455
Delgado Dobladez, J. A., Águeda Maté, V. I., Torrellas, S. Á., Larriba, M., and Brea, P. (in press). Efficient recovery of syngas from dry methane reforming product by a dual pressure swing adsorption process. Int. J. Hydrog. Energy. doi: 10.1016/j.ijhydene.2020.02.153
Donphai, W., Faungnawakij, K., Chareonpanich, M., and Limtrakul, J. (2014). Effect of Ni-CNTs/mesocellular silica composite catalysts on carbon dioxide reforming of methane. Appl. Catal. A Gen. 475, 16–26. doi: 10.1016/j.apcata.2014.01.014
Dudek, M., Adamski, A., Legutko, P., Dziadek, K., Parkhomenko, K., Aymonier, C., et al. (2019). Role of CeO2-ZrO2 support for structural. Textural and functional properties of Ni-based catalysts active in dry reforming of methane. E3S Web of Conf. 108:02018. doi: 10.1051/e3sconf/201910802018
Erdogan, B., Arbag, H., and Yasyerli, N. (2018). SBA-15 supported mesoporous Ni and Co catalysts with high coke resistance for dry reforming of methane. Int. J. Hydrog. Energy 43, 1396–1405. doi: 10.1016/j.ijhydene.2017.11.127
Estephane, J., Aouad, S., Hany, S., El Khoury, B., Gennequin, C., El Zakhem, H., et al. (2015). CO2 reforming of methane over Ni–Co/ZSM5 catalysts. Aging and carbon deposition study. Int. J. Hydrog. Energy 40, 9201–9208. doi: 10.1016/j.ijhydene.2015.05.147
Fang, X., Lian, J., Nie, K., Zhang, X., Dai, Y., Xu, X., et al. (2016). Dry reforming of methane on active and coke resistant Ni/Y2Zr2O7 catalysts treated by dielectric barrier discharge plasma. J. Energ. Chem. 25, 825–831. doi: 10.1016/j.jechem.2016.06.002
Figueira, C. E., Moreira, P. F., Giudici, R., Alves, R. M. B., and Schmal, M. (2018). Nanoparticles of Ce, Sr, Co in and out the multi-walled carbon nanotubes applied for dry reforming of methane. Appl. Catal. A Gen. 550, 297–307. doi: 10.1016/j.apcata.2017.11.019
Figueredo, G. P., Medeiros, R. L. B. A, Macedo, H. P., de Oliveira, Â. A. S., Braga, R. M., et al. (2018). A comparative study of dry reforming of methane over nickel catalysts supported on perovskite-type LaAlO3 and commercial α-Al2O3. Int. J. Hydrog. Energy 43, 11022–11037. doi: 10.1016/j.ijhydene.2018.04.224
Foppa, L., Silaghi, M.-C., Larmier, K., and Comas-Vives, A. (2016). Intrinsic reactivity of Ni, Pd and Pt surfaces in dry reforming and competitive reactions: insights from first principles calculations and microkinetic modeling simulations. J. Catal. 343, 196–207. doi: 10.1016/j.jcat.2016.02.030
Forouzesh, M., Ebadi, A., Aghaeinejad-Meybodi, A., and Khoshbouy, R. (2019). Transformation of persulfate to free sulfate radical over granular activated carbon: effect of acidic oxygen functional groups. Chem. Eng. J. 374, 965–974. doi: 10.1016/j.cej.2019.05.220
Gao, N., Cheng, M., Quan, C., and Zheng, Y. (2020). Syngas production via combined dry and steam reforming of methane over Ni-Ce/ZSM-5 catalyst. Fuel 273:117702. doi: 10.1016/j.fuel.2020.117702
Gao, R., Song, M., Wei, Y., Yu, L., and Meng, F. (2018). CO2 reforming of methane over Ni/carbon fibers-La2O3 catalyst: effects of ultrasound-assisted method and La2O3 doping on catalytic properties and activity. Waste Biomass Valoriz. 10, 3897–3905. doi: 10.1007/s12649-018-0224-y
Gonzalez-Delacruz, V. M., Ternero, F., Pereñíguez, R., Caballero, A., and Holgado, J. P. (2010). Study of nanostructured Ni/CeO2 catalysts prepared by combustion synthesis in dry reforming of methane. Appl. Catal. A Gen. 384, 1–9. doi: 10.1016/j.apcata.2010.05.027
Guharoy, U., Le Saché, E., Cai, Q., Reina, T. R., and Gu, S. (2018). Understanding the role of Ni-Sn interaction to design highly effective CO2 conversion catalysts for dry reforming of methane. J. CO2 Util. 27, 1–10. doi: 10.1016/j.jcou.2018.06.024
Gurav, H. R., Dama, S., Samuel, V., and Chilukuri, S. (2017). Influence of preparation method on activity and stability of Ni catalysts supported on Gd doped ceria in dry reforming of methane. J. CO2 Util. 20, 357–367. doi: 10.1016/j.jcou.2017.06.014
Hambali, H. U., Jalil, A. A., Abdulrasheed, A. A., Siang, T. J., and Vo, D.-V. N. (2020). Enhanced dry reforming of methane over mesostructured fibrous Ni/MFI zeolite: Influence of preparation methods. Energy Inst. J. 93, 1535–1543. doi: 10.1016/j.joei.2020.01.016
Hassani Rad, S. J., Haghighi, M., Alizadeh Eslami, A., Rahmani, F., and Rahemi, N. (2016). Sol–gel vs. impregnation preparation of MgO and CeO2 doped Ni/Al2O3 nanocatalysts used in dry reforming of methane: effect of process conditions, synthesis method and support composition. Int. J. Hydrog. Energy 41, 5335–5350. doi: 10.1016/j.ijhydene.2016.02.002
Hernández, B., and Martín, M. (2019). Optimal production of syngas via super-dry reforming. Analysis for natural gas and biogas under different CO2 taxes. Chem. Eng. Res. Des. 148, 375–392. doi: 10.1016/j.cherd.2019.06.030
Horváth, É., Baán, K., Varga, E., Oszkó, A., Vágó, Á., Töro, M., et al. (2017). Dry reforming of CH4 on Co/Al2O3 catalysts reduced at different temperatures. Catal. Today 281, 233–240. doi: 10.1016/j.cattod.2016.04.007
Hu, X., Jia, X., Zhang, X., Liu, Y., and Liu, C.-J. (2019). Improvement in the activity of Ni/ZrO2 by cold plasma decomposition for dry reforming of methane. Catal. Commun. 128:105720. doi: 10.1016/j.catcom.2019.105720
Jang, W.-J., Kim, H.-M., Shim, J.-O., Yoo, S.-Y., Jeon, K.-W., Na, H.-S., et al. (2018). Key properties of Ni–MgO–CeO2, Ni–MgO–ZrO2, and Ni–MgO–Ce(1−−x)Zr(x)O2catalysts for the reforming of methane with carbon dioxide. Green Chem. 20, 1621–1633. doi: 10.1039/C7GC03605A
Jawad, A., Rezaei, F., and Rownaghi, A. A. (2019). Highly efficient Pt/Mo-Fe/Ni-based Al2O3-CeO2 catalysts for dry reforming of methane. Catal. Today. doi: 10.1016/j.cattod.2019.06.004
Jiang, X., Mira, D., and Cluff, D. L. (2018). The combustion mitigation of methane as a non-CO2 greenhouse gas. Prog. Energy Combust. Sci. 66, 176–199. doi: 10.1016/j.pecs.2016.06.002
Jin, L., Xie, T., Ma, B., Li, Y., and Hu, H. (2017). Preparation of carbon-Ni/MgO-Al2O3 composite catalysts for CO2 reforming of methane. Int. J. Hydrog. Energy 42, 5047–5055. doi: 10.1016/j.ijhydene.2016.11.130
Jing, J.-Y., Wei, Z.-H., Zhang, Y.-B., Bai, H.-C., and Li, W.-Y. (in press). Carbon dioxide reforming of methane over MgO-promoted Ni/SiO2 catalysts with tunable Ni particle size. Catal. Today. doi: 10.1016/j.cattod.2020.01.006
Khavarian, M., Chai, S.-P., and Mohamed, A. R. (2014). Direct use of as-synthesized multi-walled carbon nanotubes for carbon dioxide reforming of methane for producing synthesis gas. Chem. Eng. J. 257, 200–208. doi: 10.1016/j.cej.2014.05.079
Khoja, A. H., Tahir, M., Amin, N. A. S., Javed, A., and Mehran, M. T. (2020). Kinetic study of dry reforming of methane using hybrid DBD plasma reactor over La2O3 co-supported Ni/MgAl2O4 catalyst. Int. J. Hydrog. Energy 45, 12256–12271. doi: 10.1016/j.ijhydene.2020.02.200
Kim, S. S., Lee, S. M., Won, J. M., Yang, H. J., and Hong, S. C. (2015). Effect of Ce/Ti ratio on the catalytic activity and stability of Ni/CeO2-TiO2 catalyst for dry reforming of methane. Chem. Eng. J. 280, 433–440. doi: 10.1016/j.cej.2015.06.027
Kim, W. Y., Jang, J. S., Ra, E. C., Kim, K. Y., Kim, E. H., and Lee, J. S. (2019). Reduced perovskite LaNiO3 catalysts modified with Co and Mn for low coke formation in dry reforming of methane. Appl. Catal. A Gen. 575, 198–203. doi: 10.1016/j.apcata.2019.02.029
Kumar, S., Bera, R., Das, N., and Koh, J. (2020). Chitosan-based zeolite-Y and ZSM-5 porous biocomposites for H2 and CO2 storage. Carbohydr. Polym. 232:115808. doi: 10.1016/j.carbpol.2019.115808
Laprune, D., Theodoridi, C., Tuel, A., Farrusseng, D., and Meunier, F. C. (2017). Effect of polyaromatic tars on the activity for methane steam reforming of nickel particles embedded in silicalite-1. Appl. Catal. B 204, 515–524. doi: 10.1016/j.apcatb.2016.12.004
Lee, S., and Lim, H. (2020). The effect of changing the number of membranes in methane carbon dioxide reforming: a CFD study. J. Ind. Eng. Chem. 87, 110–119. doi: 10.1016/j.jiec.2020.03.020
Li, B., Luo, Y., Li, B., Yuan, X., and Wang, X. (2019). Catalytic performance of iron-promoted nickel-based ordered mesoporous alumina FeNiAl catalysts in dry reforming of methane. Fuel Process. Technol. 193, 348–360. doi: 10.1016/j.fuproc.2019.05.033
Li, H., Xu, H., and Wang, J. (2011). Methane reforming with CO2 to syngas over CeO2-promoted Ni/Al2O3-ZrO2 catalysts prepared via a direct sol-gel process. J. Nat. Gas Chem. 20, 1–8. doi: 10.1016/S1003-9953(10)60156-9
Li, K., Chang, X., Pei, C., Li, X., Chen, S., Zhang, X., et al. (2019). Ordered mesoporous Ni/La2O3 catalysts with interfacial synergism towards CO2 activation in dry reforming of methane. Appl. Catal. B 259:118092. doi: 10.1016/j.apcatb.2019.118092
Li, M., and van Veen, A. C. (2018). Tuning the catalytic performance of Ni-catalysed dry reforming of methane and carbon deposition via Ni-CeO2-interaction. Appl. Catal. B 237, 641–648. doi: 10.1016/j.apcatb.2018.06.032
Li, S., Qin, X., Zhang, G., Xu, Y., and Lv, Y. (2020). Optimization of content of components over activated carbon catalyst on CO2 reforming of methane using multi-response surface methodology. Int. J. Hydrog. Energy 45, 9695–9709. doi: 10.1016/j.ijhydene.2020.01.226
Li, W., Sun, L., Xie, L., Deng, X., Guan, N., and Li, L. (2019). Coordinatively unsaturated sites in zeolite matrix: construction and catalysis. Chinese J. Catal. 40, 1255–1281. doi: 10.1016/S1872-2067(19)63381-4
Li, X., Li, D., Tian, H., Zeng, L., Zhao, Z.-J., and Gong, J. (2017). Dry reforming of methane over Ni/La2O3 nanorod catalysts with stabilized Ni nanoparticles. Appl. Catal. B 202, 683–694. doi: 10.1016/j.apcatb.2016.09.071
Li, Z., Jiang, B., Wang, Z., and Kawi, S. (2018). High carbon resistant Ni@Ni phyllosilicate@SiO2 core shell hollow sphere catalysts for low temperature CH4 dry reforming. J. CO2 Util. 27, 238–246. doi: 10.1016/j.jcou.2018.07.017
Li, Z., and Sibudjing, K. (2018). Facile synthesis of multi-Ni-Core@Ni phyllosilicate@CeO2 shell hollow spheres with high oxygen vacancy concentration for dry reforming of CH4. ChemCatChem 10, 2994–3001. doi: 10.1002/cctc.201800335
Liang, C., Wei, T., Wang, H., Yu, Z., Dong, D., Zhang, S., et al. (2020). Impacts of La addition on formation of the reaction intermediates over alumina and silica supported nickel catalysts in methanation of CO2. J. Energy Inst. 93, 723–738. doi: 10.1016/j.joei.2019.05.009
Liu, A., Praserthdam, S., and Phatanasri, S. (in press). Investigation on the increased stability of the Ni–Co bi-metallic catalysts for the carbon dioxide reforming of methane. Catal. Today. doi: 10.1016/j.cattod.2019.07.047
Long, Y., Li, K., Gu, Z., Zhu, X., Wei, Y., Lu, C., et al. (2020). Ce-Fe-Zr-O/MgO coated monolithic oxygen carriers for chemical looping reforming of methane to co-produce syngas and H2. Chem. Eng. J. 388:124190. doi: 10.1016/j.cej.2020.124190
Luisetto, I., Tuti, S., Romano, C., Boaro, M., Di Bartolomeo, E., Kesavan, J. K., et al. (2019). Dry reforming of methane over Ni supported on doped CeO2: new insight on the role of dopants for CO2 activation. J. CO2 Util. 30, 63–78. doi: 10.1016/j.jcou.2019.01.006
Lv, C., Xu, L., Chen, M., Cui, Y., Wen, X., Li, Y., et al. (2020). Recent progresses in constructing the highly efficient ni based catalysts with advanced low-temperature activity toward CO2 methanation. Front. Chem. 8:269. doi: 10.3389/fchem.2020.00269
Ma, Q., Han, Y., Wei, Q., Makpal, S., Gao, X., Zhang, J., et al. (2020). Stabilizing Ni on bimodal mesoporous-macroporous alumina with enhanced coke tolerance in dry reforming of methane to syngas. J. CO2 Util. 35, 288–297. doi: 10.1016/j.jcou.2019.10.010
Ma, Q., Wang, D., Wu, M., Zhao, T., Yoneyama, Y., and Tsubaki, N. (2013). Effect of catalytic site position: Nickel nanocatalyst selectively loaded inside or outside carbon nanotubes for methane dry reforming. Fuel 108, 430–438. doi: 10.1016/j.fuel.2012.12.028
Ma, Y., Ma, Y., Long, G., Li, J., Hu, X., Ye, Z., et al. (2019). Synergistic promotion effect of MgO and CeO2 on nanofibrous Ni/Al2O3 catalysts for methane partial oxidation. Fuel 258:116103. doi: 10.1016/j.fuel.2019.116103
Marinho, A. L. A., Rabelo-Neto, R. C., Epron, F., Bion, N., Toniolo, F. S., and Noronha, F. B. (2020). Embedded Ni nanoparticles in CeZrO2 as stable catalyst for dry reforming of methane. Appl. Catal. B 268:118387. doi: 10.1016/j.apcatb.2019.118387
Martínez Galeano, Y., Negri, F., Moreno, M. S., Múnera, J., Cornaglia, L., and Tarditi, A. M. (2019). Pt encapsulated into NaA zeolite as catalyst for the WGS reaction. Appl. Catal. A Gen. 572, 176–184. doi: 10.1016/j.apcata.2018.12.034
Marzano, M., Pontón, P. I., and Marinkovic, B. A. (2019). Co-precipitation of low-agglomerated Y2W3O12 nanoparticles: the effects of aging time, calcination temperature and surfactant addition. Ceram. Int. 45, 20189–20196. doi: 10.1016/j.ceramint.2019.06.288
Mo, W., Ma, F., Ma, Y., and Fan, X. (2019). The optimization of Ni–Al2O3 catalyst with the addition of La2O3 for CO2-CH4 reforming to produce syngas. Int. J. Hydrog. Energy 44, 24510–24524. doi: 10.1016/j.ijhydene.2019.07.204
Moradi, G., Khezeli, F., and Hemmati, H. (2016). Syngas production with dry reforming of methane over Ni/ZSM-5 catalysts. J. Nat. Gas. Sci. Eng. 33, 657–665. doi: 10.1016/j.jngse.2016.06.004
Movasati, A., Alavi, S. M., and Mazloom, G. (2019). Dry reforming of methane over CeO2-ZnAl2O4 supported Ni and Ni-Co nano-catalysts. Fuel 236, 1254–1262. doi: 10.1016/j.fuel.2018.09.069
Muhammad, A., Tahir, M., Al-Shahrani, S. S., Mahmood Ali, A., and Rather, S. U. (2020). Template free synthesis of graphitic carbon nitride nanotubes mediated by lanthanum (La/g-CNT) for selective photocatalytic CO2 reduction via dry reforming of methane (DRM) to fuels. Appl. Surf. Sci. 504:144177. doi: 10.1016/j.apsusc.2019.144177
Nabgan, W., Nabgan, B., Tuan Abdullah, T. A., Ngadi, N., Jalil, A. A., Hassan, N. S., et al. (2020). Conversion of polyethylene terephthalate plastic waste and phenol steam reforming to hydrogen and valuable liquid fuel: synthesis effect of Ni–Co/ZrO2 nanostructured catalysts. Int. J. Hydrog. Energy 45, 6302–6317. doi: 10.1016/j.ijhydene.2019.12.103
Németh, M., Sáfrán, G., Horváth, A., and Somodi, F. (2019). Hindered methane decomposition on a coke-resistant Ni-In/SiO2 dry reforming catalyst. Catal. Commun. 118, 56–59. doi: 10.1016/j.catcom.2018.10.003
Niu, J., Ran, J., and Chen, D. (2020). Understanding the mechanism of CO2 reforming of methane to syngas on Ni@Pt surface compared with Ni(1 1 1) and Pt(1 1 1). Appl. Surf. Sci. 513:145840. doi: 10.1016/j.apsusc.2020.145840
Okutan, C., Arbag, H., Yasyerli, N., and Yasyerli, S. (2020). Catalytic activity of SBA-15 supported Ni catalyst in CH4 dry reforming: effect of Al, Zr, and Ti co-impregnation and Al incorporation to SBA-15. Int. J. Hydrog. Energy 45, 13911–13928. doi: 10.1016/j.ijhydene.2020.03.052
Padi, S. P., Shelly, L., Komarala, E. P., Schweke, D., Hayun, S., and Rosen, B. A. (2020). Coke-free methane dry reforming over nano-sized NiO-CeO2 solid solution after exsolution. Catal. Commun. 138:105951. doi: 10.1016/j.catcom.2020.105951
Pan, C., Guo, Z., Dai, H., Ren, R., and Chu, W. (2020). Anti-sintering mesoporous Ni–Pd bimetallic catalysts for hydrogen production via dry reforming of methane. Int. J. Hydrog. Energy 45, 16133–16143. doi: 10.1016/j.ijhydene.2020.04.066
Pang, Y., Dou, Y., Zhong, A., Jiang, W., Gu, L., Feng, X., et al. (2018). Nanostructured Ru-Co@SiO2: highly efficient yet durable for CO2 reforming of methane with a desirable H2/CO ratio. Appl. Catal. A Gen. 555, 27–35. doi: 10.1016/j.apcata.2018.02.003
Park, J.-H., Heo, I., and Chang, T.-S. (2019). Dry reforming of methane over Ni-substituted CaZrNiOx catalyst prepared by the homogeneous deposition method. Catal. Commun. 120, 1–5. doi: 10.1016/j.catcom.2018.11.006
Pejjai, B., Reddivari, M., and Kotte, T. R. R. (2020). Phase controllable synthesis of CuS nanoparticles by chemical co-precipitation method: effect of copper precursors on the properties of CuS. Mater. Chem. Phys. 239:122030. doi: 10.1016/j.matchemphys.2019.122030
Peng, H., Zhang, X., Zhang, L., Rao, C., Lian, J., Liu, W., et al. (2017). One-Pot Facile Fabrication of Multiple Nickel Nanoparticles Confined in Microporous Silica Giving a Multiple-Cores@Shell Structure as a Highly Efficient Catalyst for Methane Dry Reforming. ChemCatChem 9, 127–136. doi: 10.1002/cctc.201601263
Pizzolitto, C., Pupulin, E., Menegazzo, F., Ghedini, E., Di Michele, A., Mattarelli, M., et al. (2019). Nickel based catalysts for methane dry reforming: effect of supports on catalytic activity and stability. Int. J. Hydrog. Energy 44, 28065–28076. doi: 10.1016/j.ijhydene.2019.09.050
Rahbar Shamskar, F., Rezaei, M., and Meshkani, F. (2017). The influence of Ni loading on the activity and coke formation of ultrasound-assisted co-precipitated Ni–Al2O3 nanocatalyst in dry reforming of methane. Int. J. Hydrog. Energy 42, 4155–4164. doi: 10.1016/j.ijhydene.2016.11.067
Ramezani, Y., Meshkani, F., and Rezaei, M. (2018). Promotional effect of Mg in trimetallic nickel-manganese-magnesium nanocrystalline catalysts in CO2 reforming of methane. Int. J. Hydrog. Energy 43, 22347–22356. doi: 10.1016/j.ijhydene.2018.09.222
Razak, H., Abdullah, N., Dina Setiabudi, H., Sim Yee, C., and Ainirazali, N. (2019). Refluxed synthesis of SBA-15 using sodium silicate extracted from oil palm ash for dry reforming of methane. Mater. Today Proc. 19, 1363–1372. doi: 10.1016/j.matpr.2019.11.150
Romero-Sáez, M., Dongil, A. B., Benito, N., Espinoza-González, R., Escalona, N., and Gracia, F. (2018). CO2 methanation over nickel-ZrO2 catalyst supported on carbon nanotubes: a comparison between two impregnation strategies. Appl. Catal. B 237, 817–825. doi: 10.1016/j.apcatb.2018.06.045
Ruocco, C., de Caprariis, B., Palma, V., Petrullo, A., Ricca, A., Scarsella, M., et al. (2019). Methane dry reforming on Ru perovskites, AZrRuO3: Influence of preparation method and substitution of A cation with alkaline earth metals. J. CO2 Util. 30, 222–231. doi: 10.1016/j.jcou.2019.02.009
Sassi, R., Bond, R. R., Cairns, A., Finlay, D. D., Guldenring, D., Libretti, G., et al. (2017). Dry reforming of methane over the cobalt catalyst: Theoretical insights into the reaction kinetics and mechanism for catalyst deactivation. J Electrocardiol 50, 776–780. doi: 10.1016/j.jelectrocard.2017.08.001
Shah, M., Bordoloi, A., Nayak, A. K., and Mondal, P. (2019). Effect of Ti/Al ratio on the performance of Ni/TiO2-Al2O3 catalyst for methane reforming with CO2. Fuel Process. Technol. 192, 21–35. doi: 10.1016/j.fuproc.2019.04.010
Shah, M., Mondal, P., Nayak, A. K., and Bordoloi, A. (2020). Advanced titania composites for efficient CO2 reforming with methane: Statistical method vs. experiment. J. CO2 Util. 39:101160. doi: 10.1016/j.jcou.2020.101160
Shang, Z., Li, S., Li, L., Liu, G., and Liang, X. (2017). Highly active and stable alumina supported nickel nanoparticle catalysts for dry reforming of methane. Appl. Catal. B 201, 302–309. doi: 10.1016/j.apcatb.2016.08.019
Shi, H., Luo, J., Wang, F., Pu, Y. F., Yang, J. H., Xiao, F. K., et al. (2019). Synthesis of CeO2-ZrO2 solid solutions for thermochemical CO2 splitting. Energy Technol. 7:1800890. doi: 10.1002/ente.201800890
Shin, S. A., Noh, Y. S., Hong, G. H., Park, J. I., Song, H. T., Lee, K.-Y., et al. (2018). Dry reforming of methane over Ni/ZrO2-Al2O3 catalysts: Effect of preparation methods. J. Taiwan Inst. Chem. 90, 25–32. doi: 10.1016/j.jtice.2017.11.032
Silveira, E. B., Rabelo-Neto, R. C., and Noronha, F. B. (2017). Steam reforming of toluene, methane and mixtures over Ni/ZrO2 catalysts. Catal. Today 289, 289–301. doi: 10.1016/j.cattod.2016.08.024
Singha, R. K., Yadav, A., Shukla, A., Iqbal, Z., Pendem, C., Sivakumar, K., et al. (2016). Promoting effect of CeO2 and MgO for CO2 reforming of methane over Ni-ZnO catalyst. Chem Select 1, 3075–3085. doi: 10.1002/slct.201600685
Snoeckx, R., Zeng, Y. X., Tu, X., and Bogaerts, A. (2015). Plasma-based dry reforming: improving the conversion and energy efficiency in a dielectric barrier discharge. RSC Adv. 5, 29799–29808. doi: 10.1039/C5RA01100K
Song, Y., Ozdemir, E., Ramesh, S., Adishev, A., Subramanian, S., Harale, A., et al. (2020). Dry reforming of methane by stable Ni-Mo nanocatalysts on single-crystalline MgO. Science 367, 777–781. doi: 10.1126/science.aav2412
Stroud, T., Smith, T. J., Le Sach,é, E., Santos, J. L., Centeno, M. A., Arellano-Garcia, H., et al. (2018). Chemical CO2 recycling via dry and bi reforming of methane using Ni-Sn/Al2O3 and Ni-Sn/CeO2-Al2O3 catalysts. Appl. Catal. B 224, 125–135. doi: 10.1016/j.apcatb.2017.10.047
Sun, H., Zhang, Q., Wen, J., Tang, T., Wang, H., Liu, M., et al. (2020). Insight into the role of CaO in coke-resistant over Ni-HMS catalysts for CO2 reforming of methane. Appl. Surf. Sci. 521:146395. doi: 10.1016/j.apsusc.2020.146395
Sun, Y., Zhang, G., Liu, J., Zhao, P., Hou, P., Xu, Y., et al. (2018). Effect of different activated carbon support on CH4CO2 reforming over Co-based catalysts. Int. J. Hydrog. Energy 43, 1497–1507. doi: 10.1016/j.ijhydene.2017.11.170
Sun, Y., Zhang, G., Xu, Y., and Zhang, R. (2019a). Dry reforming of methane over Co-Ce-M/AC-N catalyst: effect of promoters (Ca and Mg) and preparation method on catalytic activity and stability. Int. J. Hydrog. Energy 44, 22972–22982. doi: 10.1016/j.ijhydene.2019.07.010
Sun, Y., Zhang, G., Xu, Y., Zhang, Y., Lv, Y., and Zhang, R. (2019b). Comparative study on dry reforming of methane over Co-M (M = Ce, Fe, Zr) catalysts supported on N-doped activated carbon. Fuel Process. Technol. 192, 1–12. doi: 10.1016/j.fuproc.2019.04.017
Sutthiumporn, K., Maneerung, T., Kathiraser, Y., and Kawi, S. (2012). CO2 dry-reforming of methane over La0.8Sr0.2Ni0.8M0.2O3 perovskite (M = Bi, Co, Cr, Cu, Fe): Roles of lattice oxygen on C–H activation and carbon suppression. Int. J. Hydrog. Energy 37, 11195–11207. doi: 10.1016/j.ijhydene.2012.04.059
Swirk, K., Zhang, H., Li, S., Chen, Y., Rønning, M., Motak, M., et al. (2020). Carbon-resistant NiO-Y2O3-nanostructured catalysts derived from double-layered hydroxides for dry reforming of methane. Catal. Today. doi: 10.1016/j.cattod.2020.03.032
Taherian, Z., Yousefpour, M., Tajally, M., and Khoshandam, B. (2017). Catalytic performance of Samaria-promoted Ni and Co/SBA-15 catalysts for dry reforming of methane. Int. J. Hydrog. Energy 42, 24811–24822. doi: 10.1016/j.ijhydene.2017.08.080
Tahir, B., Tahir, M., and Amin, N. A. S. (2018). Tailoring performance of La-modified TiO2 nanocatalyst for continuous photocatalytic CO2 reforming of CH4 to fuels in the presence of H2O. Energy Convers. Manag. 159, 284–298. doi: 10.1016/j.enconman.2017.12.089
Tan, Y., Wang, S., Li, L., Meng, B., Chen, J., Yang, Z., et al. (2019). Application of microwave heating for methane dry reforming catalyzed by activated carbon. Chem. Eng. Process. 145:107662. doi: 10.1016/j.cep.2019.107662
Tao, K., Zhou, S., Zhang, Q., Kong, C., Ma, Q., Tsubaki, N., et al. (2013). Sol–gel auto-combustion synthesis of Ni–CexZr1−−xO2 catalysts for carbon dioxide reforming of methane. RSC Adv. 3, 22285–22294. doi: 10.1039/c3ra42522c
Tian, J., Li, H., Zeng, X., Wang, Z., Huang, J., and Zhao, C. (2019). Facile immobilization of Ni nanoparticles into mesoporous MCM-41 channels for efficient methane dry reforming. Chin J. Catal. 40, 1395–1404. doi: 10.1016/S1872-2067(19)63403-0
Tran, N. T., Van Le, Q., Van Cuong, N., Nguyen, T. D., Huy Phuc, N. H., Phuong, P. T. T., et al. (2020). La-doped cobalt supported on mesoporous alumina catalysts for improved methane dry reforming and coke mitigation. Energy Inst. J. 93, 1571–1580. doi: 10.1016/j.joei.2020.01.019
Tsoukalou, A., Imtiaz, Q., Kim, S. M., Abdala, P. M., Yoon, S., and Müller, C. R. (2016). Dry-reforming of methane over bimetallic Ni–M/La2O3 (M = Co, Fe): the effect of the rate of La2O2CO3 formation and phase stability on the catalytic activity and stability. J Catal. 343, 208–214. doi: 10.1016/j.jcat.2016.03.018
Tu, P. H., Le, D. N., Dao, T. D., Tran, Q.-T., Doan, T. C. D., Shiratori, Y., et al. (2019). Paper-structured catalyst containing CeO2-Ni flowers for dry reforming of methane. Int. J. Hydrog. Energy. 45, 18363–18375. doi: 10.1016/j.ijhydene.2019.10.247
Turap, Y., Wang, I., Fu, T., Wu, Y., Wang, Y., and Wang, W. (2020). Co–Ni alloy supported on CeO2 as a bimetallic catalyst for dry reforming of methane. Int. J. Hydrog. Energy 45, 6538–6548. doi: 10.1016/j.ijhydene.2019.12.223
Usman, M., Wan, W., Daud, M. A., and Abbas, H. F. (2015). Dry reforming of methane: influence of process parameters-a review. Renew. Sustain. Energy Rev. 45, 710–744. doi: 10.1016/j.rser.2015.02.026
Vafaeian, Y., Haghighi, M., and Aghamohammadi, S. (2013). Ultrasound assisted dispersion of different amount of Ni over ZSM-5 used as nanostructured catalyst for hydrogen production via CO2 reforming of methane. Energy Convers. Manag. 76, 1093–1103. doi: 10.1016/j.enconman.2013.08.010
Wang, F., Han, B., Zhang, L., Xu, L., Yu, H., and Shi, W. (2018). CO2 reforming with methane over small-sized Ni@SiO2 catalysts with unique features of sintering-free and low carbon. Appl. Catal. B 235, 26–35. doi: 10.1016/j.apcatb.2018.04.069
Wang, F., Xu, L., and Shi, W. (2016a). Syngas production from CO2 reforming with methane over core-shell Ni@SiO2 catalysts. J. CO2 Util. 16, 318–327. doi: 10.1016/j.jcou.2016.09.001
Wang, F., Xu, L., Shi, W., Zhang, J., Wu, K., Zhao, Y., et al. (2016b). Thermally stable Ir/Ce0.9La0.1O2 catalyst for high temperature methane dry reforming reaction. Nano Res. 10, 364–380. doi: 10.1007/s12274-016-1296-2
Wang, H., Han, J., Bo, Z., Qin, L., Wang, Y., and Yu, F. (2019). Non-thermal plasma enhanced dry reforming of CH4 with CO2 over activated carbon supported Ni catalysts. Mol. Catal. 475:110486. doi: 10.1016/j.mcat.2019.110486
Wang, H., Zhao, B., Qin, L., Wang, Y., Yu, F., and Han, J. (2020). Non-thermal plasma-enhanced dry reforming of methane and CO2 over Ce-promoted Ni/C catalysts. Mol. Catal. 485:110821. doi: 10.1016/j.mcat.2020.110821
Wang, N., Qian, W., Chu, W., and Wei, F. (2016). Crystal-plane effect of nanoscale CeO2 on the catalytic performance of Ni/CeO2 catalysts for methane dry reforming. Catal. Sci. Technol. 6, 3594–3605. doi: 10.1039/C5CY01790D
Wang, N., Yu, X., Shen, K., Chu, W., and Qian, W. (2013). Synthesis, characterization and catalytic performance of MgO-coated Ni/SBA-15 catalysts for methane dry reforming to syngas and hydrogen. Int. J. Hydrog. Energy 38, 9718–9731. doi: 10.1016/j.ijhydene.2013.05.097
Wang, X., Zhu, L., Liu, Y., and Wang, S. (2018). CO2 methanation on the catalyst of Ni/MCM-41 promoted with CeO2. Sci. Total. Environ. 625, 686–695. doi: 10.1016/j.scitotenv.2017.12.308
Wang, Y., Yao, L., Wang, S., Mao, D., and Hu, C. (2018a). Low-temperature catalytic CO2 dry reforming of methane on Ni-based catalysts: a review. Fuel Process. Technol. 169, 199–206. doi: 10.1016/j.fuproc.2017.10.007
Wang, Y., Yao, L., Wang, Y., Wang, S., Zhao, Q., Mao, D., et al. (2018b). Low-temperature catalytic CO2 dry reforming of methane on Ni-Si/ZrO2 catalyst. ACS Catal. 8, 6495–6506. doi: 10.1021/acscatal.8b00584
Wang, Y., Zheng, Y., Wang, Y., Li, K., Wang, Y., Jiang, L., et al. (2019). Syngas production modified by oxygen vacancies over CeO2-ZrO2-CuO oxygen carrier via chemical looping reforming of methane. Appl. Surf. Sci. 481, 151–160. doi: 10.1016/j.apsusc.2019.03.050
Wang, Z., Cao, X. M., Zhu, J., and Hu, P. (2014). Activity and coke formation of nickel and nickel carbide in dry reforming: a deactivation scheme from density functional theory. J Catal. 311, 469–480. doi: 10.1016/j.jcat.2013.12.015
Wei, Q., Yang, G., Gao, X., Yamane, N., Zhang, P., Liu, G., et al. (2017). Ni/Silicalite-1 coating being coated on SiC foam: a tailor-made monolith catalyst for syngas production using a combined methane reforming process. Chem. Eng. J. 327, 465–473. doi: 10.1016/j.cej.2017.06.109
Wei, Y., Horlyck, J., Song, M., Scott, J., Amal, R., and Cao, Q. (2020). Inducing Ni phyllosilicate formation over a carbon fiber support as a catalyst for the CO2 reforming of methane. Appl. Catal. A Gen. 592:117418. doi: 10.1016/j.apcata.2020.117418
Xie, L.-F., Duan, P.-G., Jiao, J.-L., and Xu, Y.-P. (2019). Hydrothermal gasification of microalgae over nickel catalysts for production of hydrogen-rich fuel gas: effect of zeolite supports. Int. J. Hydrog. Energy 44, 5114–5124. doi: 10.1016/j.ijhydene.2018.09.175
Xu, L., Liu, W., Zhang, X., Tao, L., Xia, L., Xu, X., et al. (2019). Ni/La2O3 catalysts for dry reforming of methane: insights into the factors improving the catalytic performance. ChemCatChem 11, 2887–2899. doi: 10.1002/cctc.201900331
Xu, L., Miao, Z., Song, H., Chen, W., and Chou, L. (2014b). Significant roles of mesostructure and basic modifier for ordered mesoporous Ni/CaO–Al2O3 catalyst towards CO2 reforming of CH4. Catal. Sci. Technol. 4, 1759–1770. doi: 10.1039/C3CY01037F
Xu, L., Miao, Z., Song, H., and Chou, L. (2014a). CO2 reforming of CH4 over rare earth elements functionalized mesoporous Ni–Ln (Ln = Ce, La, Sm, Pr)–Al–O composite oxides. Int. J. Hydrog. Energy 39, 3253–3268. doi: 10.1016/j.ijhydene.2013.12.077
Xu, L., Song, H., and Chou, L. (2012a). Mesoporous nanocrystalline ceria–zirconia solid solutions supported nickel based catalysts for CO2 reforming of CH4. Int. J. Hydrog. Energy 37, 18001–18020. doi: 10.1016/j.ijhydene.2012.09.128
Xu, L., Song, H., and Chou, L. (2012b). One-pot synthesis of ordered mesoporous NiO–CaO–Al2O3 composite oxides for catalyzing CO2 reforming of CH4. ACS Catal. 2, 1331–1342. doi: 10.1021/cs3001072
Xu, L., Song, H., and Chou, L. (2013). Ordered mesoporous MgO–Al2O3 composite oxides supported Ni based catalysts for CO2 reforming of CH4: effects of basic modifier and mesopore structure. Int. J. Hydrog. Energy 38, 7307–7325. doi: 10.1016/j.ijhydene.2013.04.034
Xu, L., Wang, F., Chen, M., Fan, X., Yang, H., Nie, D., et al. (2017). Alkaline-promoted Co-Ni bimetal ordered mesoporous catalysts with enhanced coke-resistant performance toward CO2 reforming of CH4. J. CO2 Util. 18, 1–14. doi: 10.1016/j.jcou.2017.01.003117
Xu, L., Wang, F., Chen, M., Zhang, J., Yuan, K., Wang, L., et al. (2016a). Carbon dioxide reforming of methane over cobalt-nickel bimetal-doped ordered mesoporous alumina catalysts with advanced catalytic performances. ChemCatChem 8, 2536–2548. doi: 10.1002/cctc.201600472114
Xu, L., Zhang, X., Chen, M., Qi, L., Nie, D., and Ma, Y. (2016b). Facilely fabricating Mg. Ca modified Co based ordered mesoporous catalysts for CO2 reforming of CH4: the effects of basic modifiers. Int. J. Hydrog. Energy 41, 17348–17360. doi: 10.1016/j.ijhydene.2016.08.013
Xu, L., Zhao, H., Song, H., and Chou, L. (2012c). Ordered mesoporous alumina supported nickel based catalysts for carbon dioxide reforming of methane. Int. J. Hydrog. Energy 37, 7497–7511. doi: 10.1016/j.ijhydene.2012.01.105
Xu, Y., Du, X.-H., Li, J., Wang, P., Zhu, J., Ge, F. J., et al. (2019). A comparison of Al2O3 and SiO2 supported Ni-based catalysts in their performance for the dry reforming of methane. J. Fuel Chem. Technol. 47, 199–208. doi: 10.1016/S1872-5813(19)30010-6
Yan, X., Hu, T., Liu, P., Li, S., Zhao, B., Zhang, Q., et al. (2019). Highly efficient and stable Ni/CeO2-SiO2 catalyst for dry reforming of methane: effect of interfacial structure of Ni/CeO2 on SiO2. Appl. Catal. B 246, 221–231. doi: 10.1016/j.apcatb.2019.01.070
Yang, W., Liu, H., Li, Y., Wu, H., and He, D. (2016). CO2 reforming of methane to syngas over highly-stable Ni/SBA-15 catalysts prepared by P123-assisted method. Int. J. Hydrog. Energy 41, 1513–1523. doi: 10.1016/j.ijhydene.2015.11.044
Yentekakis, I. V., Goula, G., Hatzisymeon, M., Betsi-Argyropoulou, I., Botzolaki, G., Kousi, K., et al. (2019). Effect of support oxygen storage capacity on the catalytic performance of Rh nanoparticles for CO2 reforming of methane. Appl. Catal. B 243, 490–501. doi: 10.1016/j.apcatb.2018.10.048
Yu, L., Song, M., Wei, Y., and Xiao, J. (2018). Combining carbon fibers with Ni/γ-Al2O3 used for syngas production: part A: preparation and evaluation of complex carrier catalysts. Catalysts 8:658. doi: 10.3390/catal8120658
Zanganeh, R., Rezaei, M., and Zamaniyan, A. (2013). Dry reforming of methane to synthesis gas on NiO–MgO nanocrystalline solid solution catalysts. Int. J. Hydrog. Energy 38, 3012–3018. doi: 10.1016/j.ijhydene.2012.12.089
Zecca, A., and Chiari, L. (2010). Fossil-fuel constraints on global warming. Energy Policy 38, 1–3. doi: 10.1016/j.enpol.2009.06.068
Zhang, G., Liu, J., Xu, Y., and Sun, Y. (2019). Ordered mesoporous Ni/Silica-carbon as an efficient and stable catalyst for CO2 reforming of methane. Int. J. Hydrog. Energy 44, 4809–4820. doi: 10.1016/j.ijhydene.2019.01.017
Zhang, J., Ren, M., Li, X., Hao, Q., Chen, H., and Ma, X. (2020). Ni-based catalysts prepared for CO2 reforming and decomposition of methane. Energy Convers. Manag. 205:112419. doi: 10.1016/j.enconman.2019.112419
Zhang, L., Wang, F., Zhu, J., Han, B., Fan, W., Zhao, L., et al. (2019). CO2 reforming with methane reaction over Ni@SiO2 catalysts coupled by size effect and metal-support interaction. Fuel 256:115954. doi: 10.1016/j.fuel.2019.115954
Zhang, M., Zhang, J., Zhou, Z., Chen, S., Zhang, T., Song, F., et al. (2020). Effects of the surface adsorbed oxygen species tuned by rare-earth metal doping on dry reforming of methane over Ni/ZrO2 catalyst. Appl. Catal. B 264:118522. doi: 10.1016/j.apcatb.2019.118522
Zhang, Q., Sun, M., Ning, P., Long, K., Wang, J., Tang, T., et al. (2019). Effect of thermal induction temperature on re-dispersion behavior of Ni nanoparticles over Ni/SBA-15 for dry reforming of methane. Appl. Surf. Sci. 469, 368–377. doi: 10.1016/j.apsusc.2018.10.222
Zhang, Q., Zhang, T., Shi, Y., Zhao, B., Wang, M., Liu, Q., et al. (2017). A sintering and carbon-resistant Ni-SBA-15 catalyst prepared by solid-state grinding method for dry reforming of methane. J. CO2 Util. 17, 10–19. doi: 10.1016/j.jcou.2016.11.002
Zhang, X., Zhang, L., Peng, H., You, X., Peng, C., Xu, X., et al. (2018). Nickel nanoparticles embedded in mesopores of AlSBA-15 with a perfect peasecod-like structure: a catalyst with superior sintering resistance and hydrothermal stability for methane dry reforming. Appl. Catal. B 224, 488–499. doi: 10.1016/j.apcatb.2017.11.001
Zhao, K., He, F., Huang, Z., Zheng, A., Li, H., and Zhao, Z. (2014). La1−xSrxFeO3 perovskites as oxygen carriers for the partial oxidation of methane to syngas. Chinese J. Catal. 35, 1196–1205. doi: 10.1016/S1872-2067(14)60084-X
Zhao, X., Li, H., Zhang, J., Shi, L., and Zhang, D. (2016). Design and synthesis of NiCe@m-SiO2 yolk-shell framework catalysts with improved coke- and sintering-resistance in dry reforming of methane. Int. J. Hydrog. Energy 41, 2447–2456. doi: 10.1016/j.ijhydene.2015.10.111
Zhao, Y., Kang, Y., Li, H., and Li, H. (2018). CO2 conversion to synthesis gas via DRM on the durable Al2O3/Ni/Al2O3 sandwich catalyst with high activity and stability. Green Chem. 20, 2781–2787. doi: 10.1039/C8GC00743H
Zhou, H., Zhang, T., Sui, Z., Zhu, Y. A., Han, C., Zhu, K., et al. (2018). A single source method to generate Ru-Ni-MgO catalysts for methane dry reforming and the kinetic effect of Ru on carbon deposition and gasification. Appl. Catal. B 233, 143–159. doi: 10.1016/j.apcatb.2018.03.103
Zhu, Q., Wang, Y., Wang, L., Yang, Z., Wang, L., Meng, X., et al. (2020). Solvent-free crystallization of ZSM-5 zeolite on SiC foam as a monolith catalyst for biofuel upgrading. Chinese J. Catal. 41, 1118–1124. doi: 10.1016/S1872-2067(20)63550-1
Zhu, Y.-A., Chen, D., Zhou, X.-G., and Yuan, W.-K. (2009). DFT studies of dry reforming of methane on Ni catalyst. Catal. Today 148, 260–267. doi: 10.1016/j.cattod.2009.08.022
Zou, H., Chen, S., Huang, J., and Zhao, Z. (2017). Effect of impregnation sequence on the catalytic performance of NiMo carbides for the tri-reforming of methane. Int. J. Hydrog. Energy 42, 20401–20409. doi: 10.1016/j.ijhydene.2017.06.203
Keywords: advanced Ni-based catalyst, sintering-proof and anti-coke, carbon dioxide, dry reforming of methane, recent progresses
Citation: Wu X, Xu L, Chen M, Lv C, Wen X, Cui Y, Wu C-e, Yang B, Miao Z and Hu X (2020) Recent Progresses in the Design and Fabrication of Highly Efficient Ni-Based Catalysts With Advanced Catalytic Activity and Enhanced Anti-coke Performance Toward CO2 Reforming of Methane. Front. Chem. 8:581923. doi: 10.3389/fchem.2020.581923
Received: 10 July 2020; Accepted: 13 August 2020;
Published: 24 September 2020.
Edited by:
Tianyi Ma, The University of Newcastle, AustraliaReviewed by:
Wenming Liu, Nanchang University, ChinaBamidele Victor Ayodele, Universiti Tenaga Nasional, Malaysia
Bawadi Abdullah, University of Technology Petronas, Malaysia
Copyright © 2020 Wu, Xu, Chen, Lv, Wen, Cui, Wu, Yang, Miao and Hu. This is an open-access article distributed under the terms of the Creative Commons Attribution License (CC BY). The use, distribution or reproduction in other forums is permitted, provided the original author(s) and the copyright owner(s) are credited and that the original publication in this journal is cited, in accordance with accepted academic practice. No use, distribution or reproduction is permitted which does not comply with these terms.
*Correspondence: Leilei Xu, leileixu88@gmail.com; Mindong Chen, chenmdnuist@163.com; Xun Hu, xun.hu@outlook.com
†These authors have contributed equally to this work