Structural Evolution of α-Fe2O3(0001) Surfaces Under Reduction Conditions Monitored by Infrared Spectroscopy
- Institute of Functional Interfaces (IFG), Karlsruhe Institute of Technology, Karlsruhe, Germany
The precise determination of the surface structure of iron oxides (hematite and magnetite) is a vital prerequisite to understand their unique chemical and physical properties under different conditions. Here, the atomic structure evolution of the hematite (0001) surface under reducing conditions was tracked by polarization-resolved infrared reflection absorption spectroscopy (IRRAS) using carbon monoxide (CO) as a probe molecule. The frequency and intensity of the CO stretch vibration is extremely sensitive to the valence state and electronic environments of surface iron cations. Our comprehensive IRRAS results provide direct evidence that the monocrystalline, stoichiometric α-Fe2O3(0001) surface is single Fe-terminated. The initial reduction induced by annealing at elevated temperatures produces surface oxygen vacancies, where the excess electrons are localized at adjacent subsurface iron ions (5-fold coordinated). A massive surface restructuring occurs upon further reduction by exposing to atomic hydrogen followed by Ar+-sputtering and annealing under oxygen poor conditions. The restructured surface is identified as a Fe3O4(111)/Fe1−xO(111)-biphase exposing both, Fe3+ and Fe2+ surface species. Here the well-defined surface domains of Fe3O4(111) exhibit a Feoct2-termination, while the reduced Fe1−xO(111) is Fe2+(oct)-terminated. These findings are supported by reference IRRAS data acquired for CO adsorption on magnetite (111) and (001) monocrystalline surfaces.
Introduction
Although iron oxides have been the topic of numerous studies, their surface is of complex nature and their structure depends strongly on the interaction with the ambience (Kuhlenbeck et al., 2013; Parkinson, 2016). Not only because of the comprehensive phase diagram and the ability to form polymorphs, but also because of the unique material properties including magnetism, the surface properties of iron oxide are among the most challenging research fields in inorganic chemistry and solid-state physics. In previous works, a number of structural conversions and surface reconstructions have been reported (Ketteler et al., 2001; Weiss and Ranke, 2002; Sakurai et al., 2009; Kuhlenbeck et al., 2013; Parkinson, 2016). It is therefore not surprising that the discussion on many common iron oxide facets toward surface stabilization and termination has remained controversial. For detailed insights, we refer the reader to two recent excellent review papers (Kuhlenbeck et al., 2013; Parkinson, 2016). In section Preview on Related Iron Oxide Crystal Structures, we have briefly summarized the important experimental and theoretical results reported in the literature for three relevant iron oxide surfaces [α-Fe2O3(0001), Fe3O4(111), Fe3O4(001)] in the form of both thin films and single crystals.
The chemical and physical properties of metal oxides strongly depend on the structural arrangement of atoms at their surfaces (Wang and Wöll, 2017). Furthermore, it has been shown that the chemical reactivity can be dramatically changed in the presence of defects like oxygen vacancies. Especially under reducing conditions there are numerous such defects, with their densities depending on temperature and the surrounding atmosphere (Parkinson, 2016). However, it is difficult to gain atomic-level information on metal oxide surface structures since some of the standard methods in surface science cannot be applied in a straightforward fashion, e.g., due to charging problems.
This problem can be avoided when using non-destructive surface analysis methods insensitive to charging effects. An important example is infrared (IR) spectroscopy. In combination with appropriate probe molecules like carbon monoxide (CO), this vibrational spectroscopy has been shown to provide insights into structural, electronic and reactive properties of model and nanostructured catalysts (Yang and Wöll, 2017). In addition, the interaction between CO and iron oxide (α-Fe2O3, hematite, Fe3O4, magnetite, Fe1−xO, wüstite) is of great interest in itself because of detailed knowledge about the binding of CO to iron oxides is required for a fundamental understanding of industrial catalytic processes such as CO oxidation and water gas-shift reactions (de Smit and Weckhuysen, 2008; Freund et al., 2011; Zhu and Wachs, 2016; Wei et al., 2018). The adsorption of CO on various well-defined metal surfaces has been extensively studied by infrared reflection absorption spectroscopy (IRRAS). However, the application of this approach on metal oxide single-crystal surfaces is challenging due to the inherent experimental difficulties arising from the low reflectivity of dielectric substrates in the IR regime. Recent progress in instrumentation, however, has allowed us to obtain high-resolution data for a number of different oxide surfaces (e.g., CeO2, TiO2, ZnO, α-Fe2O3) (Buchholz et al., 2016; Yu et al., 2016; Yang et al., 2017a,b; Chen et al., 2019; Schöttner et al., 2019). These IRRAS results have demonstrated that CO is well suited as a probe molecule to monitor the atomic structure evolution of oxide surfaces under different conditions. In particular, defects like oxygen vacancies and surface restructuring could be clearly identified (Xu et al., 2012; Yang et al., 2014, 2017a,b).
To the best of our knowledge, no IRRAS data of CO adsorption on surfaces of macroscopic iron oxide single crystals have been reported so far. Several studies have been reported on related systems including CO adsorbed on α-Fe2O3 powders (Zecchina et al., 1988) and Fe3O4(111) thin films grown on Pt(111) (Lemire et al., 2004, 2005; Kuhlenbeck et al., 2013; Li et al., 2018). Here, we present a thorough polarization-resolved IRRAS study of CO adsorption on differently treated iron (III/II) oxide single-crystal surfaces, focusing on α-Fe2O3(0001) that has shown to be the most abundant facet exposed by hematite nanoparticles (Hartman, 1989). Our systematic IRRAS results allow to gain detailed insight into the structural evolution of the α-Fe2O3(0001) surface under reducing conditions. The assignment of the IR bands was assisted by additional reference measurements of CO adsorption on well-defined Fe3O4(111) and Fe3O4(001) monocrystalline substrates.
Models And Methods
Preview on Related Iron Oxide Crystal Structures
α-Fe2O3 crystallizes in a corundum structure characterized by a slightly distorted hexagonal close-packed oxide-ion lattice where 2/3 of the octahedral sites are occupied by Fe3+ ions with non-degenerated energy levels on metal d-electrons due to a ligand field splitting originating from Fe-O hybridization (Figure S1) (de Groot et al., 1989). Oxygen ions that are located parallel to (0001) plane levels, are separated by an iron double layer, yielding a stacking sequence of O3-Fe-Fe repeat units. There are three different surface terminations possible for α-Fe2O3(0001): a single iron layer (Fe-O3-Fe-R), a double iron layer (Fe-Fe-O3-R) and an oxygen (O3-Fe-Fe-R) layer (Figure 1A).
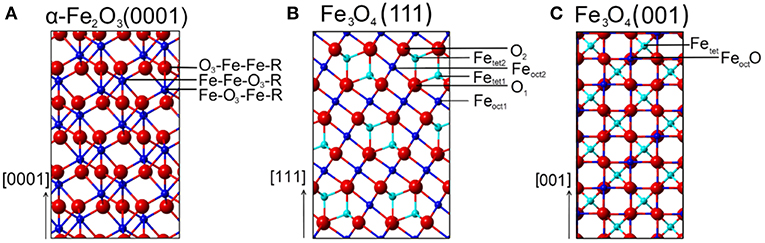
Figure 1. Schematic representations (side view) of various surface terminations for (A) α-Fe2O3(0001), (B) Fe3O4(111), and (C) Fe3O4(001) surfaces. Red spheres correspond to oxide lattice ions; dark blue spheres and cyan spheres represent iron ions on octahedral (oct) and tetrahedral (tet) lattice sites, respectively.
It is known that the structural and electronic properties of hematite (0001) depends strongly on the interaction with the ambience and the preparation parameters (Ketteler et al., 2001; Weiss and Ranke, 2002; Kuhlenbeck et al., 2013; Parkinson, 2016). In particular, the surface termination α-Fe2O3(0001) is highly sensitive to the oxygen partial pressure and preparation temperature. Freund and coworkers have systematically studied the surface structures of α-Fe2O3(0001) thin films supported on Pt(111) by scanning tunneling microscopy (STM) and IRRAS (Lemire et al., 2005). The results revealed a ferryl (Fe = O) termination coexisting with domains of the Fe-terminated surface in 10−3-1 mbar O2 at ~1,050 K, while the preparation at higher pressures of O2 (>1 mbar) yields O-terminated surfaces (Shaikhutdinov and Weiss, 1999). In contrast, an exclusive Fe-termination was reported on thin films (Thevuthasan et al., 1999) as well as on single crystal substrates under O-deficient conditions (<10−5 mbar) (Yamamoto, 2010; Schöttner et al., 2019). For α-Fe2O3(0001) single crystals, it has been reported that Ar+-sputtering followed by annealing in 10−6 mbar of O2 at about 1,000 K leads to a massive surface restructuring, forming a Fe3O4(111) layer (Condon et al., 1994, 1998; Camillone et al., 2002). A biphase structure was observed upon heating in oxygen (10−6 mbar) to higher temperatures (~1,100 K) (Condon et al., 1995, 1998; Camillone et al., 2002). The STM results revealed the formation of long-range order of α-Fe2O3(0001) and Fe1−xO(111) domains, which are characterized by floret-like spots in the low-energy electron diffraction (LEED) pattern described as (√3 × √3)R30° reconstruction (Kurtz and Henrich, 1983; Lad and Henrich, 1988; Condon et al., 1995). In addition, the surface chemistry and physics of α-Fe2O3(0001) has been studied by a number of theoretical groups [for details see the review by Parkinson, 2016] (Wang et al., 1998; Bergermayer et al., 2004; Trainor et al., 2004; Nguyen et al., 2014; Lewandowski et al., 2016; Ovcharenko et al., 2016).
In the crystal structure of Fe3O4 oxygen anions are aligned in a cubic closed packing of equal spheres, where Fe3+ cations are located at tetrahedrally coordinated interstitial sites. Other cations are located at octahedrally coordinated lattice positions, among which 50% exhibit the valence state +3 while the other 50% are in the +2 oxidation state. This chemical composition is typical for inverse spinel bulk structures and can be written as Fe3+[Fe3+Fe2+]O4 to illustrate that the first type is tetrahedrally coordinated (A-type), whereas the parenthesized Fe2+ and Fe3+ ions occupy octahedrally coordinated sites (B-type). At low-pressure and low-temperature (CO measurement conditions), Fe3O4 consists of a crystal structure with monoclinic symmetry (Wright et al., 2002; Bengtson et al., 2013). Above 120 K, known as the Verwey temperature (Verwey, 1939), the octahedrally coordinated iron atoms in magnetite have an average oxidation state of Fe +2.5 due to electron hopping between equal numbers of Fe2+ and Fe3+ ions. Such electron movement is frozen in Fe3O4 below 120 K and therefore accompanied by a significant decrease in the electrical conductivity.
For Fe3O4(111) surfaces, six terminations may occur when cleaving the (111) stacking sequence (Figure 1B). The different terminations exhibit Fe atoms on octahedral (Feoct1 or Feoct2) and tetrahedral (Fetet1 or Fetet2) lattice sites, as well as O layers (O1 or O2). The surface structure of Fe3O4(111) has been the subject of numerous experimental and theoretical investigations. Quantum chemical calculations have predicted that the Feoct2-termination is energetically favored on Fe3O4(111) under oxygen-deficient conditions, followed closely by the Fetet1 termination (Ahdjoudj et al., 1999; Zhu et al., 2006; Grillo et al., 2008; Noh et al., 2015). Recent work combining IRRAS and DFT (Li et al., 2018) revealed that the regular Fe3O4(111) thin film is Fetet1-terminated over close-packed oxygen layer, in line with the LEED IV results (Ritter and Weiss, 1999; Sala et al., 2012). STM studies provided further evidence for the Fetet1 termination on the pristine Fe3O4(111) surface, while the Feoct2 termination was observed under oxygen-poor conditions (Lennie et al., 1996; Shimizu et al., 2010). In addition, a long-range ordered biphase structure was reported for reduced Fe3O4(111) in the form of both thin films (Shaikhutdinov et al., 1999) and single-crystals (Condon et al., 1997; Paul et al., 2007). The STM and LEED data suggested the coexistence of Fe3O4(111) and Fe1−xO(111) domains.
From the ideal Fe3O4(001) model, only two surface terminations are possible, namely Fetet and FeoctO layers (Figure 1C). Again, the surface structure of Fe3O4(001) depends strongly on the preparation parameters (Tarrach et al., 1993; Parkinson et al., 2011; Bartelt et al., 2013). The polar Fe3O4(001) surface is well-known to be stabilized by a (√2 × √2)R45° reconstruction. The reconstructed surface was first studied using STM by Wiesendanger et al. (1992) and later by other groups [see e.g., Chambers and Joyce, 1999; Rustad et al., 1999; Stanka et al., 2000; Mariotto et al., 2002]. Recently, Parkinson et al. (Bliem et al., 2014) proposed a subsurface cation vacancy (SCV) model by using STM in conjunction with LEED IV analysis and theory. In this model, the reconstructed surface features a FeoctO termination (B-layer) and is slightly distorted by a rearrangement of the cations in the subsurface layers.
Overall, due to the great complexity, an unambiguous experimental determination of the surface atomic structure for these iron oxides under different conditions is still a challenging task.
Experimental Details
The IR measurements were carried out in an UHV multi-chamber system (Prevac). The base pressure of the entire chambers is in 10−10 mbar range. The IR spectra were recorded in reflection absorption mode using a state-of-the-art Fourier-Transform (FT)-IR spectrometer (Bruker Vertex 80v) that is directly coupled to the UHV chamber via differentially pumped KBr-windows. The IR-spectrometer contains an internal polarizer module, which allows to carry out polarization-resolved IRRAS experiments. All CO adsorption measurements reported here were conducted for the respective iron oxide substrate mounted in a cryo-pumped IR-chamber at low temperatures. With liquid-He cooling, we could achieve sample temperatures of 65 K, which was sufficiently low to yield CO adsorption on all substrates studied here. Exposures to CO were carried out by using a leak-valve based directional doser. Gas dosages are quoted in Langmuir (1 L = 1.33·10−6 mbar·s). The IR spectra were recorded after saturating the iron oxide surfaces with CO (1 L). The polarization-dependent IRRAS measurements were conducted with p- and/or s-polarized light at grazing incidence, with the angle of 80° with respect to the surface normal. The IRRA spectra are shown in absorbance mode. The resolution of the IR spectrometer was set to 4 cm−1 and 2,048 scans were accumulated for a single spectrum.
Iron oxide single crystals were mounted on a sample holder dedicated for e-beam heating. Temperatures were measured with a NiAl-NiCr thermocouple, that is spot-welded at a position between heating plate and backside of the sample. Surface quality and cleanliness were checked by XPS and LEED. Supplementary XPS measurements on the oxidation states of iron atoms for differently prepared iron oxide surfaces were conducted at the HESGM-beamline of the BESSY II storage ring, which is a part of the Helmholtz-Center Berlin.
The iron oxide single crystals used in this study were cut from minerals and polished (SurfaceNet GmbH). The α-Fe2O3(0001) substrate was cleaned by repeated cycles of annealing with stepwise heating from 850 K to 950 K in 10−5 mbar O2 (Supporting information, Figures S2A–C) (Lübbe and Moritz, 2009; Schöttner et al., 2019). The slightly reduced Fe2O3(0001) was formed after over-annealing the sample by gradual heating from 950 K to 1,050 K in 10−5 mbar O2. A highly reduced surface was prepared by exposing the clean and stoichiometric α-Fe2O3(0001) to atomic hydrogen followed by post-annealing at 1,000 K. Then the reduced α-Fe2O3(0001) surface was prepared by repeated cycles of sputtering with 1.0 keV Ar+ for 15 min and subsequent annealing at 950 K in 10−6 mbar oxygen for 20 min until a LEED pattern gets visible.
The Fe3O4(111) single crystal surface was prepared by sputtering with 1.0 keV Ar+ for 15 min and subsequent annealing at 920 K in 10−6 mbar oxygen for 20 min. The surface of Fe3O4(001) was cleaned by Ar+-sputtering at 1.0 keV for 15 min and annealing at 870 K in a background of oxygen at 10−6 mbar for 20 min (Figure S3) (Nie et al., 2013).
Results And Discussion
CO Adsorption on the Pristine α-Fe2O3(0001) Surface
In Figure 2 we display polarization-resolved IRRAS data recorded after a saturating exposure of the pristine stoichiometric α-Fe2O3(0001) single crystal surface to CO at 65 K. For p-polarized light, we observe a single, symmetric band at 2,169 cm−1 which is characteristic for CO bound to the coordinatively unsaturated surface Fe3+ cations (Zecchina et al., 1988), indicating the presence of a well-defined surface with only one adsorption site. As mentioned above, the surface termination of iron oxides depends strongly on the preparation conditions (Kuhlenbeck et al., 2013; Parkinson, 2016). For example, the ferryl termination was observed for α-Fe2O3(0001) thin films after oxidation with O2 partial pressures of up to 1 mbar, showing a typical IR band at 2,185 cm−1 (Lemire et al., 2005), which is not seen in the present IR spectra (Figure 2). Our IRRAS data provides direct spectroscopic evidence that the pristine α-Fe2O3(0001) surface is single Fe3+-terminated.
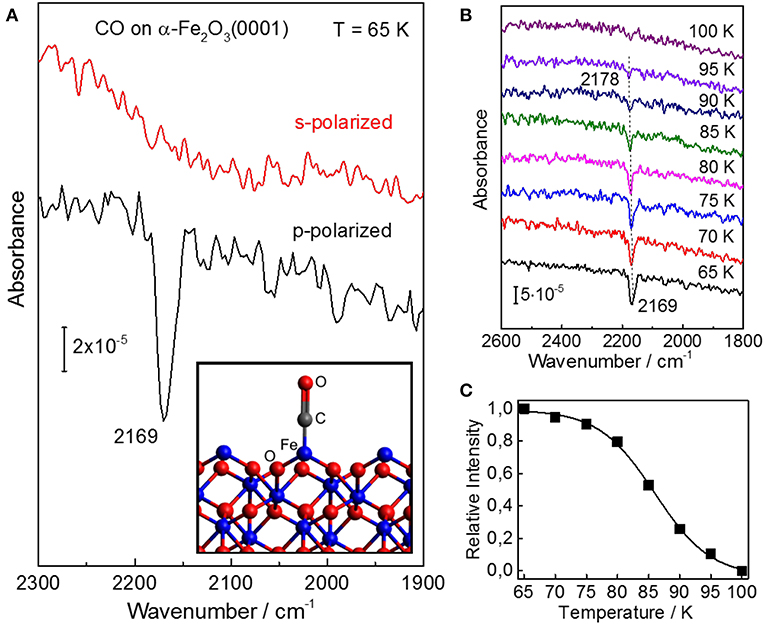
Figure 2. IRRAS results for CO adsorption on the well-ordered stoichiometric α-Fe2O3(0001) surface. (A) Polarization-dependent IRRAS data recorded after exposing the clean surface of α-Fe2O3(0001) to 1 L CO at 65 K. The adsorption model is shown as inset. (B) Temperature-dependent IRRAS data starting from a saturated CO coverage at 65 K. Here, all spectra were recorded at an incidence angle of 80° with p-polarized light. (C) The relative CO band-intensity is shown as a function of the sample temperature.
The difference between p- and s-polarizations can be understood by considering the transition dipole moment (TDM) describing the vibrational excitation of CO. The intensity and sign of an IR peak is given by the interaction of the TDM with the vector of the E-field. Note that for metal surfaces including metal-supported oxide thin films, IRRAS data can only be obtained for IR light polarized perpendicular to the surface according to the so-called surface selection rule. In the case of dielectric bulk materials (e.g., metal oxide single crystals), however, both s- and p-polarized components can interact with adsorbate vibrations being highly dependent on the orientation of TDM, thus allowing for a determination of the adsorption geometry (Wang and Wöll, 2017). The absorbance bands excited by Es are always negative, while the vibrations excited by p-polarized components (normal Ep, n and tangential Ep, t, showing always opposite signs) can be negative or positive depending on the incidence angle Θ and the refractive index n of the substrate (Brunner et al., 1997).
For the adsorption of a full CO monolayer on α-Fe2O3(0001) at 65 K, a single IR band with negative sign was observed at 2,169 cm−1 for p-polarized light but not for s-polarized light (Figure 2A). Since the TDM is oriented parallel to the CO molecular axis, these polarization-dependent IRRAS results indicate that CO is bound to surface Fe3+ ions in an upright geometry. Furthermore, we observed a coverage-dependent frequency shift (Figure S4), in line with IR results reported for CO adsorbed on α-Fe2O3 powder samples (Zecchina et al., 1988). This coverage-induced frequency shift can be explained in terms of lateral adsorbate-adsorbate interactions including both dynamic and substrate-mediated static effects. For CO adsorption on oxide surfaces, the substrate mediated interactions (static shift) play a predominant role, whereas the contribution from dynamic interactions originating from the dipole-dipole coupling between CO adsorbates is rather small (Mahan and Lucas, 1978; Hollins and Pritchard, 1980).
The coverage-dependent frequency shift in CO stretch frequency was also observed after heating the sample to higher temperatures, i.e., with decreasing the CO coverage (temperature-induced CO desorption). The intensity of the CO vibrational band remains stable on α-Fe2O3(0001) up to 70 K (Figure 2B) but strongly decreases upon further heating. The IR band has disappeared at 100 K, indicating complete desorption of CO. The thermal desorption of CO is accompanied by a blue-shift in frequency from 2,169 to 2,178 cm−1. A quantitative analysis of the temperature-dependent IRRAS results (CO coverage as a function of temperature, see Figure 2C) (Gottfried et al., 2003) yields an activation energy for CO desorption on α-Fe2O3(0001) of 24 kJ·mol−1 (using a frequency factor of 1013 s−1), demonstrating that CO binds only weakly to the surface Fe3+ ions.
CO Adsorption on Reduced α-Fe2O3(0001)
In the next step the stoichiometric α-Fe2O3(0001) was subjected to annealing in 10−5 mbar oxygen at higher temperatures increased stepwise from 850 K to 1050 K. The corresponding structure evolution was tracked by measuring the vibrational frequency of adsorbed CO. Upon annealing to 950 K, the IRRA spectrum shows only a single CO band at 2,169 cm−1 (Figure 3), assigned to CO adsorbed at the 3-fold coordinated (Fe-O3) Fe3+ sites of the stoichiometric α-Fe2O3(0001) surface, as discussed above. A new CO band at 2,163 cm−1 appears and becomes the dominating one when increasing the sample temperature to 950–1000 K under O-poor conditions. After heating the sample to 1,000–1,050 K, only the 2,163 cm−1 band is detected, while the Fe3+-related CO vibration at 2,169 cm−1 observed for the fully oxidized surface disappears completely (see Figure 3). Note that in the fitting process, the half width for all CO peaks was set to be equal.
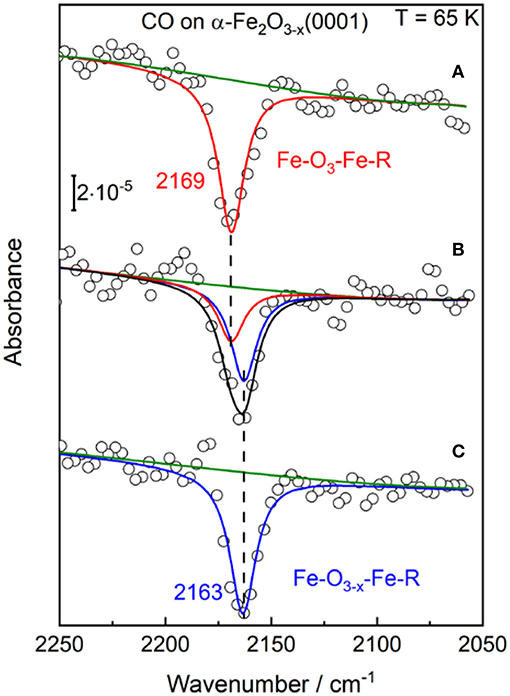
Figure 3. The p-polarized IRRA spectra obtained after saturation adsorption of CO (1 L) on α-Fe2O3(0001) at 65 K. The α-Fe2O3(0001) samples were pretreated in 10−5 mbar O2 at different temperatures: (A) 850–950 K; (B) 950–1,000 K; (C) 1,000–1,050 K.
Overall, the present IRRAS data indicate the presence of surface oxygen vacancies (VO) created by annealing to elevated temperatures. Formation of VO defects have been found to induce a red-shift of CO frequencies on other oxides [e.g., rutile TiO2(110) surface; Xu et al., 2012]. Here, the slightly reduced α-Fe2O3(0001) surface (Fe-O3−x-Fe-R) is characterized by a CO band position of 2,163 cm−1, which we assign to CO bound to 2-fold coordinated (Fe-O2) Fe3+ ions adjacent to O-vacancy sites. Importantly, the rather small red shift of 6 cm−1 stands for an only little electronic structure modification of surface iron cations, suggesting that the excess electrons are not trapped at the 2-fold coordinated surface Fe3+ ions. This finding is in excellent agreement with the DFT calculations made on the reduced α-Fe2O3(0001) surface (Ovcharenko et al., 2016). Along with the removal of a surface oxygen atom, two excess electrons were predicted to occupy the easily available Fe 3d states on two neighboring subsurface iron cations (5-fold coordinated).
We turn our attention to the experimental results for the highly reduced surface states of hematite, which were created by subjecting the stoichiometric Fe2O3(0001) to atomic hydrogen exposure followed by Ar+-sputtering and annealing cycles. Again, the changes in surface structure were monitored by measuring the CO vibrational frequency. Our IRRAS data allow to gain detailed insight into the surface structure of highly reduced α-Fe2O3(0001).
After CO adsorption at 65 K, the p-polarized IRRAS data show a number of CO bands in the regions between 2,175–2,135 cm−1 and 2,125–2,025 cm−1 (Figure 4A), which are related respectively to CO species adsorbed on surface Fe3+ and Fe2+ sites, revealing substantial changes of the surface structure. To achieve a thorough analysis of the data, we subjected the IR spectra to a peak-fitting procedure starting with Lorentzian curves, which were centered at local maxima in the spectra. In the deconvoluted spectrum (Figure 4A), four distinct CO stretch bands are resolved at 2,166, 2,150, 2,104, and 2,085 cm−1 for highly reduced α-Fe2O3(0001), in full agreement with those obtained after CO adsorption on the reduced Fe3O4(111) surface [see section CO Adsorption on Fe3O4(111) and Fe3O4(001)].
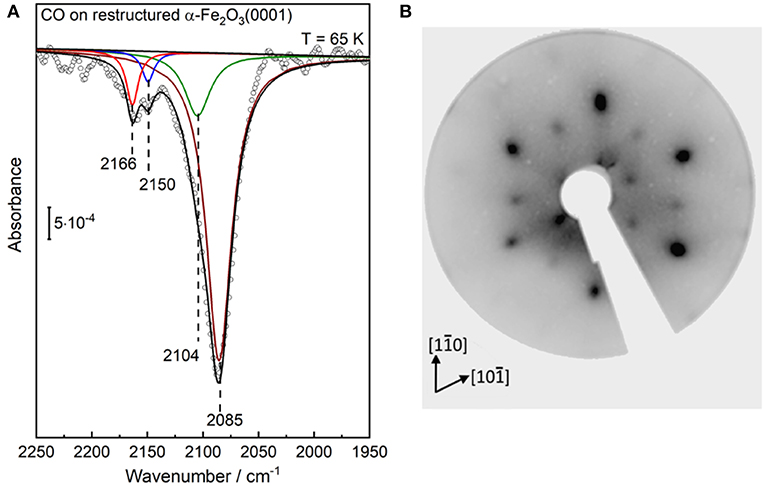
Figure 4. (A) IRRA spectrum (p-polarized) obtained after saturation adsorption of CO (1 L) at 65 K on a highly reduced α-Fe2O3(0001) surface created via atomic hydrogen treatment at RT followed by Ar+-sputtering and post-annealing at 950 K in 10−6 mbar oxygen. (B) LEED pattern of the corresponding highly reduced α-Fe2O3(0001) surfaces recorded at 90 eV.
Interestingly, Figure 4A shows that the intensity of the Fe2+-related CO bands is much stronger than that of the Fe3+-related CO vibrations at 2,166/2,150 cm−1. A higher population of CO species adsorbed to Fe2+ sites could account for this observation. However, the substantial intensity difference should be also related to a larger transition dipole moment for CO-Fe2+, resulting from the enhanced electron backdonation compared to CO/Fe3+, as confirmed by the large red-shift in frequency of CO species bound to Fe2+ cations. A similar phenomenon has been reported for CO adsorption on differently charged (Cu+ and Cu2+) copper ions, where the CO stretch intensity was calculated to be more than a factor of five higher for the CO-Cu+ than for the CO-Cu2+ complex (St. Petkov et al., 2012).
Our IRRAS data strongly suggests that the highly reduced α-Fe2O3(0001) surface reconstructs. This conclusion is further supported by the corresponding LEED pattern (Figure 4B), which reveal that the α-Fe2O3(0001) surface undergoes a complete structural conversion from α-Fe2O3(0001) to Fe3O4(111) termination, in agreement with literature (Condon et al., 1994, 1998; Camillone et al., 2002). Furthermore, the outer hexagon spots become much broader and more intense, which could be attributed to the presence of additional, ordered Fe1−xO(111) domains [further detailed explanations are given in section CO Adsorption on Fe3O4(111) and Fe3O4(001)]. The surface structures of α-Fe2O3(0001), Fe3O4(111) and Fe1−xO(111) are illustrated in Figure 5. The LEED results indicate the formation of a Fe3O4(111)/Fe1−xO(111)-biphase structure on highly reduced α-Fe2O3(0001). The approach using non-destructive IR spectroscopy and CO as a probe molecule allows to provide more reliable information on the surface termination and chemical environments because CO only interacts with the outermost surface (Wang and Wöll, 2017; Chen et al., 2019). The CO vibrations at 2,166/2,104 cm−1 and 2,150 cm−1 could be attributed to octahedral Fe3+/Fe2+ sites as well as to tetrahedral Fe3+ sites within Fe3O4(111) domains, while the CO band the 2,085 cm−1 implies the coexistence of reduced Fe1−xO(111) islands terminated by octahedral Fe2+ cations. These assignments are corroborated by reference experiments where CO was adsorbed on Fe3O4(111) single-crystal surfaces, as discussed below in section CO adsorption on Fe3O4(111) and Fe3O4(001). Further evidence for this massive surface restructuring of the highly reduced α-Fe2O3(0001) is provided by photoelectron spectroscopy, where Fe2+ and Fe3+ species are clearly identified in both Fe 2p (Figure S5A) and valence band regions (Figure S5B) (Liu et al., 2012).
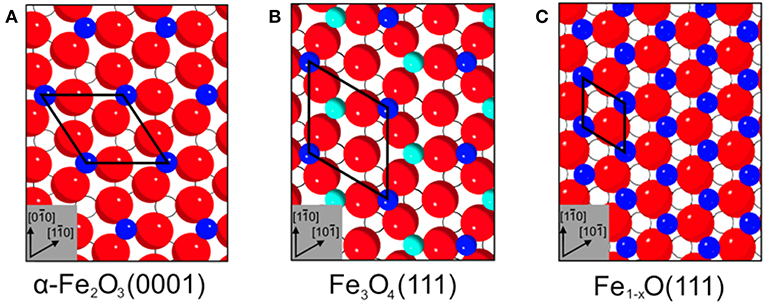
Figure 5. Top view surface structures of (A) α-Fe2O3(0001), (B) Fe3O4(111), and (C) Fe1−xO(111). The surface unit cells are (A) 5.04 Å, (B) 5.94 Å, and (C) 3.04 Å. Surface atoms are shown inked; subsurface atoms below the first oxide layer are depicted hollow. Red spheres correspond to oxide lattice ions; dark blue spheres and cyan spheres represent iron ions on octahedral and tetrahedral lattice sites, respectively.
As mentioned in section Preview on Related Iron Oxide Crystal Structures, the highly reduced α-Fe2O3(0001) surface may also feature an ordered α-Fe2O3(0001)/Fe1−xO(111)-biphase reconstruction which is characterized by floret-like satellite spots, positioned adjacent to the peaks for the pristine (1 × 1)-α-Fe2O3(0001) surface (Condon et al., 1995, 1998). However, this surface reconstruction is not supported by our combined results from IRRAS and LEED (Figure 4). Our observation is in line with the STM studies on α-Fe2O3(0001) single crystal surfaces (Condon et al., 1998), where a Fe3O4(111)-termination was created by annealing the sample at ~1,000 K in 10−6 mbar O2. The structural transformation to a α-Fe2O3(111)/Fe1−xO(111)-biphase occurs only when increasing the temperature to about 1,100 K (Condon et al., 1998). In addition, more recent DFT calculations revealed that the α-Fe2O3(0001) surface can expose O-, Fe-, and ferryl-terminated regions, whose size varies depending on the oxygen chemical potential (Lewandowski et al., 2016). The long-range order was explained in terms of the dipole-dipole interaction between domains with different work functions.
CO Adsorption on Fe3O4(111) and Fe3O4(001)
In order to aid the assignment of the CO bands and to gain a thorough understanding of the structural evolution of the α-Fe2O3(0001) surface during reduction, we have carried out additional reference measurements on Fe3O4(111) and reconstructed Fe3O4(001)-√2 × √2R45° (Figure S3) single crystal surfaces. Figure 6a shows p-polarized IRRAS data recorded after saturating the slightly (two sputter/annealing cycles) and strongly (five sputter/annealing cycles) reduced Fe3O4(111) single-crystal surfaces with CO at 65 K, which is much lower than the Verwey transition temperature of ~120 K (Verwey, 1939). The deconvoluted spectra display four CO stretch bands at 2,166, 2,150, 2,104, and 2,085 cm−1 (Figure 6a), in excellent agreement with the results found for the highly reduced surface of hematite α-Fe2O3(0001) (see Figure 4A).
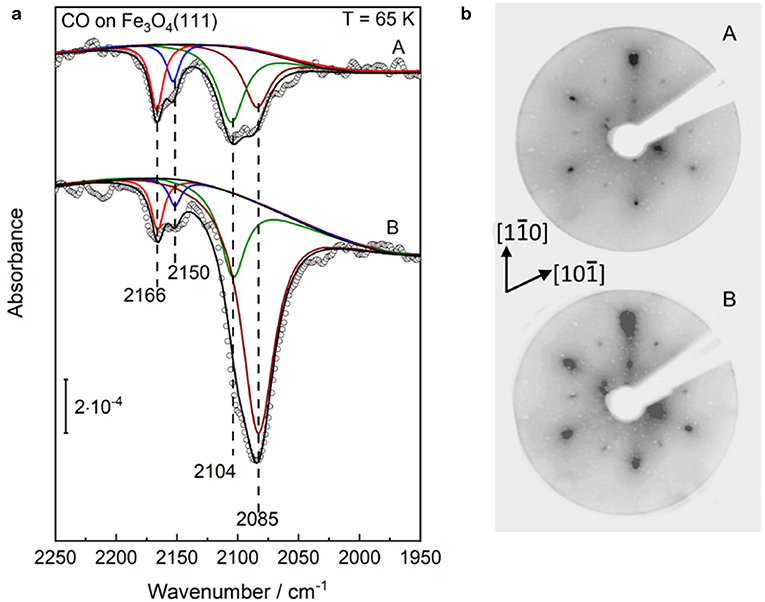
Figure 6. Structural evolution of well-defined Fe3O4(111) single-crystal surfaces after (A) slight and (B) heavy Ar+-sputtering treatment. Afterward such treatment the sample was annealed at 920 K in 10−6 mbar oxygen. (a) The p-polarized IRRAS data of CO adsorption on reduced Fe3O4(111) surfaces. The clean surfaces was saturated with a dose of 1 L CO at 65 K. (b) LEED patterns of the corresponding Fe3O4(111) surfaces recorded at 90 eV.
As discussed in section Preview on Related Iron Oxide Crystal Structures, the surface structure of Fe3O4(111) is extremely sensitive to the preparation parameters (Kuhlenbeck et al., 2013; Parkinson, 2016). The experimental and theoretical studies revealed consistently that the regular Fe3O4(111) surface is Fetet1-terminated over a close-packed oxygen layer, whereas this surface is stabilized by the Feoct2-termination under oxygen-deficient conditions (Lennie et al., 1996; Ahdjoudj et al., 1999; Ritter and Weiss, 1999; Zhu et al., 2006; Grillo et al., 2008; Shimizu et al., 2010; Sala et al., 2012; Noh et al., 2015). The vibrational frequencies at 2,166/2,104 cm−1 and 2,150 cm−1 are assigned to CO species adsorbed to octahedral Fe3+/Fe2+ sites (B type) as well as to tetrahedral Fe3+ sites (A type), respectively, indicating a Feoct2-terminated Fe3O4(111) structure (see Figure 5B).
The assignment of the CO bands is further assisted by additional IRRAS data obtained for CO adsorption on the highly reduced α-Fe2O3(0001) surface created by annealing at 950 K in a higher O2 pressure of 10−4 mbar (see Figure S6). In this condition, the Fe3O4(111) layer within the Fe3O4(111)/Fe1−xO biphase should be dominated by the Fetet1-termination. Indeed, the corresponding IR data shows a single Fe3+-related vibrational band at 2,153 cm−1, which is attributed to CO molecules bound on surface Fetet1 sites (see Figure S6).
Furthermore, we assign the low-lying IR band at 2,085 cm−1 to CO bound to Fe2+ surface sites from reduced Fe1−xO phases (see Figure 5C), as reported in the literature (Benziger and Larson, 1982). Again, the Fe1−xO(111) surface can expose either Fe or O layers according to the preparation conditions. Whereas, the STM investigations suggested the presence of O-terminated Fe1−xO(111) domains formed by oxidation of the Fe3O4(111) layer on α-Fe2O3(0001) (Tang et al., 2013), the DFT calculations predicted a Fe-termination in O-poor environments (Li et al., 2005). This assignment is supported by the observation that the 2,085 cm−1 band becomes more intense along with the Ar+-sputtering/annealing treatment of the Fe3O4(111) single-crystal surface (Figure 6a), indicating the growth of ordered Fe1−xO(111) domains, which coexist with the Feoct2-terminated Fe3O4(111) structures on the reduced Fe3O4(111) surface. The stretch frequencies of all CO species adsorbed on different iron(III/II) oxide single-crystal surfaces studied in the course of this work are summarized in Table 1.
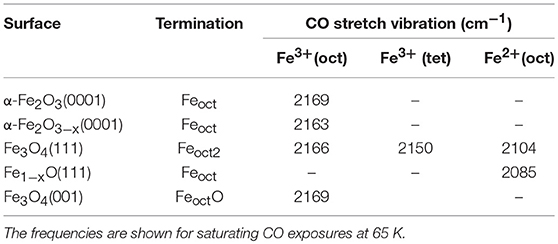
Table 1. CO stretch frequencies collected on regular and restructured hematite (0001) as well as on magnetite (111) and (001) single-crystal surfaces.
Our IR data provide direct spectroscopic evidence for the formation of a Fe3O4(111)/Fe1−xO(111) biphase structure on Fe3O4(111), in line with the results from STM and LEED (Condon et al., 1997; Shaikhutdinov et al., 1999; Paul et al., 2007). Here, the presence of a Fe3O4(111)/Fe1−xO(111) biphase is also supported by the LEED patterns shown in Figure 6B. In addition to the pristine Fe3O4(111) structure (Tarrach et al., 1993), the spots at the outer hexagon become much broader and more intense under reducing conditions, which is also seen for the highly reduced α-Fe2O3(0001) surface (see Figure 4B). This should originate from the presence of ordered Fe1−xO(111) domains. However, we did not see the floreted diffraction patterns as observed for a typical α-Fe2O3(0001)/Fe1−xO(111) biphase. This can be attributed to the rather small lattice mismatch (2%) between Fe3O4(111) and Fe1−xO(111), which makes an unambiguous observation of the floret-like satellite spots extremely difficult (Condon et al., 1997; Paul et al., 2007).
The temperature-dependent IRRAS data allows to gain deep insight into the interaction of CO molecule with various surface sites. As shown in Figure 7A, the Fe3+-related CO bands at 2,166 and 2,150 cm−1 disappear after annealing the sample to ~100 K, indicating a weak binding of CO to coordinatively unsaturated surface Fe3+ cations. The thermal stability and the frequency (blue-shifted compared to the gas phase value, see Table 1) are in good agreement with those observed on pristine α-Fe2O3(0001) (Figure 2B) and Fe3O4(001) surfaces (Figure 7B, see below), in which both are terminated by single Fe3+ species. In comparison, the Fe2+-related CO species at 2,104 and 2,085 cm−1 are thermodynamic more stable and desorb completely only upon annealing to temperatures above 165 K (Figure 7A). Again, the stronger binding of CO to Fe2+ is attributed to the enhanced electron back-donation to the CO 2π* antibonding orbital, thus strengthening the CO-substrate interaction and weakening the internal C–O bond (redshift in frequency).
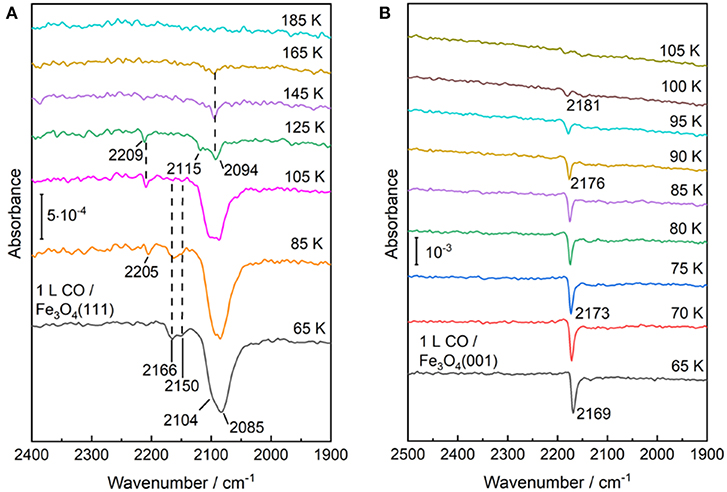
Figure 7. Temperature-dependent IRRA spectra starting from CO saturated surfaces of (A) Fe3O4(111) and (B) Fe3O4(001) at 65 K and subsequently heating to indicated temperatures. All the spectra were recorded at an incidence angle of 80° with p-polarized light at 65 K.
In addition, a new weak IR band at 2,205–2,209 cm−1 is detected after annealing to temperatures above 85 K. The origin of this band is not clear, but a similar CO species has been reported for ZnO and Au/ZnO systems and is assigned to CO coadsorbed with carbonate species that increase the Lewis acidity of adjacent Zn2+ cations (Wang et al., 2007; Noei et al., 2012). Carbonate could be produced via the oxidation of adsorbed CO with surface O atoms on Fe3O4(111) (Tinkle and Dumesic, 1987; Huang et al., 2006). In a recent study on Fe3O4(111) thin films grown on Pt(111) (Li et al., 2018), a CO vibration at 2,204 cm−1 was also observed. According to the DFT calculations, this band was ascribed to CO adsorbed at F ions located at step edges (defect sites). However, the Fe3O4(111) thin film, prepared under oxidation conditions, features a F-termination over close-packed oxygen layer, which is quite different from the present work focusing on the structural evolution of iron oxides under reducing conditions.
We now turn to the experimental results obtained on the reconstructed Fe3O4(001)-(√2 × √2)R45° surface. After adsorption of CO on the Fe3O4(001) surface at 65 K only one single sharp CO band at 2,169 cm−1 is observed (Figure 7B), which is typical for CO bound to Fe3+ sites. As discussed in section Preview on Related Iron Oxide Crystal Structures, the (√2 × √2)R45°-reconstructed Fe3O4(001) surface has been studied extensively by numerous groups (Wiesendanger et al., 1992; Tarrach et al., 1993; Chambers and Joyce, 1999; Rustad et al., 1999; Stanka et al., 2000; Mariotto et al., 2002; Parkinson et al., 2011; Bartelt et al., 2013; Bliem et al., 2014). Recently, Parkinson et al. (Bliem et al., 2014) presented a thorough study combining STM, LEED IV analysis and theory, in which a subsurface cation vacancy (SCV) model was developed. According to this model, the reconstructed surface is Feoct-O terminated, which is accompanied by a rearrangement of the cations in the subsurface layers (Bliem et al., 2014).
Our IRRAS results are fully consistent with the SCV structure in that the single IR band at 2,169 cm−1 is characteristic for CO bound to surface F sites. The assignment to Fe3+ is further supported by a temperature-programmed desorption measurement, where the IRRAS data reveal a complete desorption of CO species after heating to 105 K, in excellent agreement with the TPD results (Hulva et al., 2018). This finding demonstrates again a weak interaction between CO and surface Fe3+ cations as observed for CO adsorption on the Fe3+ sites of α-Fe2O3(0001) and Fe3O4(001) surfaces. In addition, the thermal desorption of CO leads to a blue shift in frequency from 2,169 to 2,181 cm−1 (Figure 7B), which is attributed to the lateral adsorbate-adsorbate interactions [for a detailed discussion see section CO Adsorption on the Pristine α-Fe2O3(0001) Surface].
Overall, the IRRAS results, acquired for CO adsorption on the macroscopic Fe3O4(111) and Fe3O4(001) surfaces, provide a solid basis for a reliable assignment of the CO bands observed for the restructured α-Fe2O3(0001) surface under different reduction conditions (Table 1). Importantly, the comprehensive IR data, together with XPS and LEED analysis, allow for identifying the Fe3O4(111)/Fe1−xO(111) biphase structure on highly reduced α-Fe2O3(0001) surfaces.
Conclusions
In summary, the structural evolution of differently treated iron(III/II) oxide single-crystal surfaces was monitored by polarization-resolved IRRAS using CO as a probe molecule. We presented a comprehensive investigation on stoichiometric and reduced α-Fe2O3(0001) surfaces as well as on Fe3O4(001) and Fe3O4(111) surfaces. The polarization-dependent IRRAS results demonstrate that the CO adsorption is well suited to monitor the atomic structure evolution of iron oxide surfaces under different conditions.
The pristine stoichiometric α-Fe2O3(0001) surface is terminated in a single-Fe3+ configuration as characterized by the single sharp IR band at 2,169 cm−1 (CO on 3-fold coordinated Fe3+). Annealing the hematite (0001) surface to elevated temperatures leads to the formation of surface oxygen vacancies. The reduced α-Fe2O3(0001) surface shows a typical vibrational band at 2,163 cm−1 (CO on Fe3+ at O vacancy). A further reduction of α-Fe2O3(0001) induced by atomic hydrogen treatment followed by Ar+-sputtering and annealing cycles under oxygen poor conditions causes a massive surface restructuring yielding a Fe3O4(111) overlayer, which coexists with Fe1−xO(111) domains. The reconstructed Fe3O4(111) surface is Feoct2-terminated and shows typical CO bands at 2,166/2,150 and 2,104 cm−1. They are assigned to Fe3+(oct)/Fe3+(tet) and Fe2+(oct) sites, respectively. The Fe1−xO(111) domains within the Fe3O4(111)/Fe1−xO(111) biphase are characterized by the intense band at 2,085 cm−1 originating from CO adsorbed on Fe2+(oct) sites. The assignment is further demonstrated by reference IRRAS data acquired for CO adsorption on Fe3O4(111) single-crystal surfaces. The surface of Fe3O4(001) is terminated with F-O in a SCV reconstruction as determined by the single intense IR band of CO at 2,169 cm−1.
Data Availability
All datasets generated for this study are included in the manuscript and/or the Supplementary Files.
Author Contributions
YW and CW conceived the idea of the experiment. LS and CY carried out the experiments, with additional help by AN and SH. Data analysis was carried out by LS, CY, and YW. The manuscript was written by LS, YW, and CW.
Conflict of Interest Statement
The authors declare that the research was conducted in the absence of any commercial or financial relationships that could be construed as a potential conflict of interest.
Acknowledgments
The Helmholtz-Research-School Energy-related-catalysis is gratefully acknowledged for providing financial support and the donation of a Ph.D.-Scholarship to LS. We acknowledge funding from the Science and Technology of Nanosystems Program (432202). The authors thank Helmholtz-Zentrum Berlin for the allocation of synchrotron radiation beamtime at BESSY II.
Supplementary Material
The Supplementary Material for this article can be found online at: https://www.frontiersin.org/articles/10.3389/fchem.2019.00451/full#supplementary-material
Additional XPS, LEED, and polarization-resolved IRRAS analysis.
References
Ahdjoudj, J., Martinsky, C., Minot, C., Van Hove, M. A., and Somorjai, G. A. (1999). Theoretical study of the termination of the Fe3O4 (111) surface. Surf. Sci. 443, 133–153. doi: 10.1016/S0039-6028(99)01008-0
Bartelt, N. C., Nie, S., Starodub, E., Bernal-Villamil, I., Gallego, S., Vergara, L., et al. (2013). Order-disorder phase transition on the (100) surface of magnetite. Phys. Rev. B 88:235436. doi: 10.1103/PhysRevB.88.235436
Bengtson, A., Morgan, D., and Becker, U. (2013). Spin state of iron in fe3O4 magnetite and h-Fe3O4. Phys. Rev. B 87:155141. doi: 10.1103/PhysRevB.87.155141
Benziger, J. B., and Larson, L. R. (1982). An infrared spectroscopy study of the adsorption of Co on Fe/Mgo. J. Catal. 77, 550–553. doi: 10.1016/0021-9517(82)90195-6
Bergermayer, W., Schweiger, H., and Wimmer, E. (2004). Ab initio thermodynamics of oxide surfaces: O2 on Fe2O3(0001). Phys. Rev. B 69:195409. doi: 10.1103/PhysRevB.69.195409
Bliem, R., Mc Dermott, E., Ferstl, P., Setvin, M., Gamba, O., Pavelec, J., et al. (2014). Subsurface cation vacancy stabilization of the magnetite (001) surface. Science 346, 1215–1218. doi: 10.1126/science.1260556
Brunner, H., Mayer, U., and Hoffmann, H. (1997). External reflection infrared spectroscopy of anisotropic adsorbate layers on dielectric substrates. Appl. Spectrosc. 51, 209–217. doi: 10.1366/0003702971940143
Buchholz, M., Yu, X., Yang, C., Heißler, S., Nefedov, A., Wang, Y., et al. (2016). Ir-spectroscopy of CO adsorption on mixed-terminated ZnO surfaces. Surf. Sci. 652, 247–252. doi: 10.1016/j.susc.2015.12.029
Camillone, N. III., Adib, K., Fitts, J. P., Rim, K. T., Flynn, G. W., Joyce, S. A., et al. (2002). Surface termination dependence of the reactivity of single crystal hematite with CCl4. Surf. Sci. 511, 267–282. doi: 10.1016/S0039-6028(02)01503-0
Chambers, S. A., and Joyce, S. A. (1999). Surface termination, composition and reconstruction of Fe3O4(001) and Γ-Fe2O3(001). Surf. Sci. 420, 111–122. doi: 10.1016/S0039-6028(98)00657-8
Chen, A. L., Yu, X., Zhou, Y., Miao, S., Li, Y., Kuld, S., et al. (2019). Structure of the catalytically active copper–ceria interfacial perimeter. Nat. Catal. 2, 334–341. doi: 10.1038/s41929-019-0226-6
Condon, N. G., Leibsle, F. M., Lennie, A. R., Murray, P. W., Parker, T. M., Vaughan, D. J., et al. (1998). Scanning tunnelling microscopy studies of α-Fe2O3(0001). Surf. Sci. 397, 278–287. doi: 10.1016/S0039-6028(97)00744-9
Condon, N. G., Leibsle, F. M., Lennie, A. R., Murray, P. W., Vaughan, D. J., Thornton, G., et al. (1995). Biphase ordering of iron oxide surfaces. Phys. Rev. Lett. 75, 1961–1964. doi: 10.1103/PhysRevLett.75.1961
Condon, N. G., Leibsle, F. M., Parker, T., Lennie, A. R., Vaughan, D. J., and Thornton, G. (1997). Biphase ordering on Fe3O4(111). Phys. Rev. B 55, 15885–15894. doi: 10.1103/PhysRevB.55.15885
Condon, N. G., Murray, P. W., Leibsle, F. M., Thornton, G., Lennie, A. R., and Vaughan, D. J. (1994). Fe3O4(111) termination of α- Fe3O3 (0001). Surf. Sci. 310, L609–L613. doi: 10.1016/0039-6028(94)91360-9
de Groot, F. M. F., Grioni, M., Fuggle, J. C., Ghijsen, J., Sawatzky, G. A., and Petersen, H. (1989). Oxygen 1s x-ray-absorption edges of transition-metal oxides. Phys. Rev. B 40, 5715–5723. doi: 10.1103/PhysRevB.40.5715
de Smit, E., and Weckhuysen, B. M. (2008). The renaissance of iron-based fischer–tropsch synthesis: on the multifaceted catalyst deactivation behaviour. Chem. Soc. Rev. 37, 2758–2781. doi: 10.1039/b805427d
Freund, H. J., Meijer, G., Scheffler, M., Schlögl, R., and Wolf, M. (2011). Co oxidation as a prototypical reaction for heterogeneous, processes. Angew. Chem. Int. Ed. 50, 10064–10094. doi: 10.1002/anie.201101378
Gottfried, J. M., Schmidt, K. J., Schroeder, S. L. M., and Christmann, K. (2003). Adsorption of Carbon Monoxide on Au(110)-(1 × 2). Surface Science 536, 206–224. doi: 10.1016/S0039-6028(03)00595-8
Grillo, M. E., Finnis, M. W., and Ranke, W. (2008). Surface structure and water adsorption on Fe3O4 (111): spin-density functional theory and on-site coulomb interactions. Phys. Rev. B 77:075407. doi: 10.1103/PhysRevB.77.075407
Hartman, P. (1989). The effect of surface relaxation on crystal habit: cases of corundum (α-Al2O3) and hematite (α-Fe2O3). J. Cryst. Growth 96, 667–672. doi: 10.1016/0022-0248(89)90065-1
Hollins, P., and Pritchard, J. (1980). Dipole coupling effects in the coverage-dependent infrared spectra of co chemisorbed on Cu(111). Chem. Phys. Lett. 75, 378–382.
Huang, D. M., Cao, D. B., Li, Y. W., and Jiao, H. (2006). Density function theory study of CO adsorption on Fe3O4(111) Surface. J. Phys. Chem. B 110, 13920–13925. doi: 10.1021/jp0568273
Hulva, J., Jakub, Z., Novotny, Z., Johansson, N., Knudsen, J., Schnadt, J., et al. (2018). Adsorption of CO on the Fe3O4 (001) Surface. J. Phys. Chem. B 122, 721–729. doi: 10.1021/acs.jpcb.7b06349
Ketteler, G., Weiss, W., Ranke, W., and Schlögl, R. (2001). Bulk and surface phases of iron oxides in an oxygen and water atmosphere at low pressure. Phys. Chem. Chem. Phys. 3, 1114–1122. doi: 10.1039/b009288f
Kuhlenbeck, H., Shaikhutdinov, S., and Freund, H. J. (2013). Well-ordered transition metal oxide layers in model catalysis – a series of case studies. Chem. Rev. 113, 3986–4034. doi: 10.1021/cr300312n
Kurtz, R. L., and Henrich, V. E. (1983). Geometric structure of the α-Fe2O3(0001) surface: a leed and Xps study. Surf. Sci. 129, 345–354. doi: 10.1016/0039-6028(83)90185-1
Lad, R. J., and Henrich, V. E. (1988). Structure of α-Fe2O3 single crystal surfaces following Ar+ ion bombardment and annealing in O2. Surf. Sci. 193, 81–93. doi: 10.1016/0039-6028(88)90324-X
Lemire, C., Bertarione, S., Zecchina, A., Scarano, D., Chaka, A., Shaikhutdinov, S., et al. (2005). Ferryl (Fe = O) termination of the hematite α-Fe2O3 (0001) Surface. Phys. Rev. Lett. 94:166101. doi: 10.1103/PhysRevLett.94.166101
Lemire, C., Meyer, R., Henrich, V. E., Shaikhutdinov, S., and Freund, H. J. (2004). The surface structure of Fe3O4(111) films as studied by CO adsorption. Surf. Sci. 572, 103–114. doi: 10.1016/j.susc.2004.08.033
Lennie, A. R., Condon, N. G., Leibsle, F. M., Murray, P. W., Thornton, G., and Vaughan, D. J. (1996). Structures of Fe = O4 (111) surfaces observed by scanning tunneling microscopy. Phys. Rev. B 53, 10244–10253. doi: 10.1103/PhysRevB.53.10244
Lewandowski, M., Groot, I. M. N., Qin, Z. H., Ossowski, T., Pabisiak, T., Kiejna, A., et al. (2016). Nanoscale patterns on polar oxide surfaces. Chem. Mater. 28, 7433–7443. doi: 10.1021/acs.chemmater.6b03040
Li, X., Paier, J., Sauer, J., Mirabella, F., Zaki, E., Ivars-Barceló, F., et al. (2018). Surface termination of Fe3O4(111) films studied by CO adsorption revisited. J. Phys. Chem. B 122, 527–533. doi: 10.1021/acs.jpcb.7b04228
Li, Y. L., Yao, K. L., Liu, Z. L., and Gao, G. Y. (2005). First-principles study of the composition, structure, and stability of the Feo (111) surface. Phys. Rev. B 72:155446. doi: 10.1103/PhysRevB.72.155446
Liu, S., Wang, S., Guo, J., and Guo, Q. (2012). Polarity and surface structural evolution of iron oxide films. RSC Adv. 2, 9938–9943. doi: 10.1039/c2ra21892e
Lübbe, M., and Moritz, W. A. (2009). Leed analysis of the clean surfaces of α-Fe2O3(0001) and α-Cr2O3(0001) bulk single crystals. J. Phys. Condens. Matter 21:134010. doi: 10.1088/0953-8984/21/13/134010
Mahan, G. D., and Lucas, A. A. (1978). Collective vibrational modes of adsorbed CO. J. Chem. Phys. 68, 1344–1348. doi: 10.1063/1.435952
Mariotto, G., Murphy, S., and Shvets, I. V. (2002). Charge ordering on the surface of Fe3O4(001). Phys. Rev. B 66:245426. doi: 10.1103/PhysRevB.66.245426
Nguyen, M. T., Seriani, N., and Gebauer, R. (2014). Defective α-Fe2O3 (0001): an ab initio study. ChemPhysChem. 15, 2930–2935. doi: 10.1002/cphc.201402153
Nie, S., Starodub, E., Monti, M., Siegel, D. A., Vergara, L., El Gabaly, F., et al. (2013). Insight into magnetite's redox catalysis from observing surface morphology during oxidation. J. Am. Chem. Soc. 135, 10091–10098. doi: 10.1021/ja402599t
Noei, H., Birkner, A., Merz, K., Muhler, M., and Wang, Y. (2012). Probing the mechanism of low-temperature CO oxidation on Au/Zno catalysts by vibrational spectroscopy. J. Chem. C 116, 11181–11188. doi: 10.1021/jp302723r
Noh, J., Osman, O. I., Aziz, S. G., Winget, P., and Brédas, J. L. (2015). Magnetite Fe3O4 (111) surfaces: impact of defects on structure, stability, and electronic properties. Chem. Mater. 27, 5856–5867. doi: 10.1021/acs.chemmater.5b02885
Ovcharenko, R., Voloshina, E., and Sauer, J. (2016). Water adsorption and O-defect formation on Fe2O3(0001) surfaces. Phys. Chem. Chem. Phys. 18, 25560–25568. doi: 10.1039/C6CP05313K
Parkinson, G. S. (2016). Iron oxide surfaces. Surf. Sci. Rep. 71, 272–365. doi: 10.1016/j.surfrep.2016.02.001
Parkinson, G. S., Novotný, Z., Jacobson, P., Schmid, M., and Diebold, U. (2011). A metastable Fe(A) termination at the Fe3O4(001) surface. Surf. Sci. 605, L42–L45. doi: 10.1016/j.susc.2011.05.018
Paul, M., Sing, M., Claessen, R., Schrupp, D., and Brabers, V. A. M. (2007). Thermodynamic stability and atomic and electronic structure of reduced Fe3O4 (111) single-crystal surfaces. Phys. Rev. B 76:075412. doi: 10.1103/PhysRevB.76.075412
Ritter, M., and Weiss, W. (1999). Fe3O4 (111) surface structure determined by leed crystallography. Surf. Sci. 432, 81–94. doi: 10.1016/S0039-6028(99)00518-X
Rustad, J. R., Wasserman, E., and Felmy, A. R. (1999). A molecular dynamics investigation of surface reconstruction on magnetite (001). Surf. Sci. 432, L583–L588. doi: 10.1016/S0039-6028(99)00581-6
Sakurai, S., Namai, A., Hashimoto, K., and Ohkoshi, S. I. (2009). First observation of phase transformation of all four fe2O3 phases (Γ → E → B → A-Phase). J. Am. Chem. Soc. 131, 18299–18303. doi: 10.1021/ja9046069
Sala, A., Marchetto, H., Qin, Z. H., Shaikhutdinov, S., Schmidt, T., and Freund, H. J. (2012). Defects and inhomogeneities in Fe3O4(111) Thin film growth on Pt(111). Phys. Rev. B 86:155430. doi: 10.1103/PhysRevB.86.155430
Schöttner, L., Ovcharenko, R., Nefedov, A., Voloshina, E., Wang, Y., Sauer, J., et al. (2019). Interaction of Water Molecules with the α-Fe2O3(0001) surface: a combined experimental and computational study. J. Phys. Chem. C 123, 8324–8335. doi: 10.1021/acs.jpcc.8b08819
Shaikhutdinov, S. K., Ritter, M., Wang, X. G., Over, H., and Weiss, W. (1999). Defect structures on epitaxial Fe3O4(111) films. Phys. Rev. B 60, 11062–11069. doi: 10.1103/PhysRevB.60.11062
Shaikhutdinov, S. K., and Weiss, W. (1999). Oxygen pressure dependence of the α-Fe2O3(0001) surface structure. Surf. Sci. 432, L627–L634. doi: 10.1016/S0039-6028(99)00643-3
Shimizu, T. K., Jung, J., Kato, H. S., Kim, Y., and Kawai, M. (2010). Termination and Verwey transition of the (111) surface of magnetite studied by scanning tunneling microscopy and first-principles calculations. Phys. Rev. B 81:235429. doi: 10.1103/PhysRevB.81.235429
Stanka, B., Hebenstreit, W., Diebold, U., and Chambers, S. A. (2000). Surface reconstructions of Fe3O4(001). Surf. Sci. 448, 49–63. doi: 10.1016/S0039-6028(99)01182-6
St. Petkov, P., Vayssilov, G. N., Liu, J., Shekhah, O., Wang, Y., Wöll, C., et al. (2012). Defects in MOFs: a thorough characterization. ChemPhysChem. 13, 2025–2029. doi: 10.1002/cphc.201200222
Tang, Y., Qin, H., Wu, K., Guo, Q., and Guo, J. (2013). The reduction and oxidation of Fe2O3(0001) surface investigated by scanning tunneling microscopy. Surf. Sci. 609, 67–72. doi: 10.1016/j.susc.2012.11.005
Tarrach, G., Bürgler, D., Schaub, T., Wiesendanger, R., and Güntherodt, H. J. (1993). Atomic surface structure of Fe3O4(001) in different preparation stages studied by scanning tunneling microscopy. Surf. Sci. 285, 1–14. doi: 10.1016/0039-6028(93)90908-3
Thevuthasan, S., Kim, Y. J., Yi, S. I., Chambers, S. A., Morais, J., Denecke, R., et al. (1999). Surface structure of MBE-grown a-Fe2O3(0001) by intermediate-energy X-ray photoelectron diffraction. Surf. Sci. 425, 276–286. doi: 10.1016/S0039-6028(99)00200-9
Tinkle, M., and Dumesic, J. A. (1987). Isotopic exchange measurements of the rates of adsorption desorption and interconversion of CO and CO2 over chromia-promoted magnetite - implications for water-gas shift. J. Catal. 103, 65–78. doi: 10.1016/0021-9517(87)90093-5
Trainor, T. P., Chaka, A. M., Eng, P. J., Newville, M., Waychunas, G. A., Catalano, J. G., et al. (2004). Structure and reactivity of the hydrated hematite (0001) surface. Surf. Sci. 573, 204–224. doi: 10.1016/j.susc.2004.09.040
Verwey, E. J. W. (1939). Electronic conduction of magnetite (Fe3O4) and its transition point at low temperatures. Nature 14, 327–328. doi: 10.1038/144327b0
Wang, X. G., Weiss, W., Shaikhutdinov, S. K., Ritter, M., Petersen, M., Wagner, F., et al. (1998). The hematite (α-Fe2O3) (0001) surface: evidence for domains of distinct chemistry. Phys. Rev. Lett. 81, 1038–1041. doi: 10.1103/PhysRevLett.81.1038
Wang, Y., and Wöll, C. (2017). IR spectroscopic investigations of chemical and photochemical reactions on metal oxides: bridging the materials gap. Chem. Soc. Rev. 46, 1875–1932. doi: 10.1039/C6CS00914J
Wang, Y., Xia, X., Urban, A., Qiu, H., Strunk, J., Meyer, B., et al. (2007). Tuning the reactivity of oxide surfaces by charge - accepting adsorbates. Angew. Chem. Int. Ed. 46, 7315–7318. doi: 10.1002/anie.200702815
Wei, X., Shao, B., Zhou, Y., Li, Y., Jin, C., Liu, J., et al. (2018). Geometrical structure of the gold–iron(iii) oxide interfacial perimeter for co oxidation. Angew. Chem. 130, 11459–11463. doi: 10.1002/ange.201805975
Weiss, W., and Ranke, W. (2002). Surface chemistry and catalysis on well-defined epitaxial iron-oxide layers. Prog. Surf. Sci. 70, 1–151. doi: 10.1016/S0079-6816(01)00056-9
Wiesendanger, R., Shvets, I. V., Bürgler, D., Tarrach, G., Güntherodt, H. J., Coey, J. M. D., et al. (1992). Topographic and magnetic-sensitive scanning tunneling microscope study of magnetite. Science 255, 583–586. doi: 10.1126/science.255.5044.583
Wright, J. P., Attfield, J. P., and Radaelli, P. G. (2002). Charge ordered structure of magnetite Fe3O4 below the verwey transition. Phys. Rev. B 66:214422. doi: 10.1103/PhysRevB.66.214422
Xu, M., Noei, H., Fink, K., Muhler, M., Wang, Y., and Wöll, C. (2012). The surface science approach for understanding reactions on oxide powders: the importance of ir spectroscopy. Angew. Chem. Int. Ed. 51, 4731–4734. doi: 10.1002/anie.201200585
Yamamoto, S. (2010). Water adsorption on α-Fe2O3(0001) at near ambient conditions. J. Phys. Chem. C 114, 2256–2266. doi: 10.1021/jp909876t
Yang, C., and Wöll, C. (2017). Ir spectroscopy applied to metal oxide surfaces: adsorbate vibrations and beyond. Adv. Phys. 2, 373–408. doi: 10.1080/23746149.2017.1296372
Yang, C., Yin, L.-L., Bebensee, F., Buchholz, M., Sezen, H., Heissler, S., et al. (2014). Chemical activity of oxygen vacancies on ceria: a combined experimental and theoretical study on Ceo2(111). Phys. Chem. Chem. Phys. 16, 24165–24168. doi: 10.1039/C4CP02372B
Yang, C., Yu, X., Heißler, S., Nefedov, A., Colussi, S., Llorca, J., et al. (2017b). Surface faceting and reconstruction of ceria nanoparticles. Angew. Chem. Int. Ed. 56, 375–379. doi: 10.1002/anie.201609179
Yang, C., Yu, X., Heißler, S., Weidler, P. G., Nefedov, A., Wang, Y., et al. (2017a). O2 activation on ceria catalysts - the importance of substrate crystallographic orientation. Angew. Chem. Int. Ed. 56, 16399–16404. doi: 10.1002/anie.201709199
Yu, X. J., Zhang, Z., Yang, C., and Wöll, C. (2016). Interaction of formaldehyde with the rutile Tio2(110) surface: a combined experimental and theoretical study. J. Phys. Chem. C 120, 12626–12636. doi: 10.1021/acs.jpcc.6b03689
Zecchina, A., Scarano, D., and Reller, A. (1988). Infrared spectra of CO adsorbed on prismatic faces of α-Fe2O3. J. Chem. Soc. Faraday Transac. 1: Phys. Chem. Condens. Phases 84, 2327–2333. doi: 10.1039/f19888402327
Zhu, L., Yao, K. L., and Liu, Z. L. (2006). First-principles study of the polar (111) surface of Fe3O4. Phys. Rev. B 74:035409. doi: 10.1103/PhysRevB.74.035409
Keywords: iron oxide, CO adsorbed infrared spectroscopy, IR spectroscopy, reduced oxides, XPS
Citation: Schöttner L, Nefedov A, Yang C, Heissler S, Wang Y and Wöll C (2019) Structural Evolution of α-Fe2O3(0001) Surfaces Under Reduction Conditions Monitored by Infrared Spectroscopy. Front. Chem. 7:451. doi: 10.3389/fchem.2019.00451
Received: 01 March 2019; Accepted: 05 June 2019;
Published: 25 June 2019.
Edited by:
Maria Veronica Ganduglia-Pirovano, Institute of Catalysis and Petrochemistry (ICP), SpainReviewed by:
Shamil Shaikhutdinov, Fritz-Haber-Institut, GermanyOscar Rodríguez De La Fuente, Complutense University of Madrid, Spain
Geoff Thornton, University College London, United Kingdom
Copyright © 2019 Schöttner, Nefedov, Yang, Heissler, Wang and Wöll. This is an open-access article distributed under the terms of the Creative Commons Attribution License (CC BY). The use, distribution or reproduction in other forums is permitted, provided the original author(s) and the copyright owner(s) are credited and that the original publication in this journal is cited, in accordance with accepted academic practice. No use, distribution or reproduction is permitted which does not comply with these terms.
*Correspondence: Yuemin Wang, yuemin.wang@kit.edu; Christof Wöll, christof.woell@kit.edu