Blastocyst complementation and interspecies chimeras in gene edited pigs
- 1Lillehei Heart Institute, University of Minnesota, Minneapolis, MN, United States
- 2Cardiovascular Division, Department of Medicine, University of Minnesota, Minneapolis, MN, United States
- 3Stem Cell Institute, University of Minnesota, Minneapolis, MN, United States
- 4Paul and Sheila Wellstone Muscular Dystrophy Center, University of Minnesota, Minneapolis, MN, United States
The only curative therapy for many endstage diseases is allograft organ transplantation. Due to the limited supply of donor organs, relatively few patients are recipients of a transplanted organ. Therefore, new strategies are warranted to address this unmet need. Using gene editing technologies, somatic cell nuclear transfer and human induced pluripotent stem cell technologies, interspecies chimeric organs have been pursued with promising results. In this review, we highlight the overall technical strategy, the successful early results and the hurdles that need to be addressed in order for these approaches to produce a successful organ that could be transplanted in patients with endstage diseases.
Introduction
Endstage organ failure or acute traumatic injuries are associated with considerable morbidity and mortality. The only curative therapy for many of these terminal or devastating diseases is solid organ transplantation (Garry et al., 2005; Virani et al., 2021). Due to the limited number of organ donors, this curative therapy is available for only a subpopulation of the patients that need these therapies. For example, it is estimated that 200,000 to 300,000 American adults would benefit from orthotopic heart transplantation annually, but only about 3000 adults receive a cardiac transplant (Virani et al., 2021). This disparity is what drives the pursuit of alternative therapies. In addition to endstage organ disease such as heart disease, there are traumatic injuries that threaten limbs and ultimately contribute to volumetric muscle loss (Corona et al., 2015; Greising et al., 2016). Currently, there are limited treatments for volumetric muscle loss and thus lead to considerable morbidity, amputations, lifelong disability and loss of life (Greising et al., 2017). These chronic diseases and traumatic injuries warrant new therapies.
Technological advancements such as gene editing (Doudna and Charpentier, 2014; Jinek et al., 2012; Cong et al., 2013) and somatic cell nuclear transfer (SCNT) technologies (Polejaeva et al., 2000; Tian et al., 2003; Galli and Lazzari, 2021) have now allowed for genetic modification of large animals such as the pig (Lai et al., 2002; Yamada et al., 2005; Hisashi et al., 2008; Pierson et al., 2020; Watanabe et al., 2020; Hinrichs et al., 2021; Reichart et al., 2021) (Figure 1). These genetically modified pigs have renewed the interest in xenotransplantation but they also provide a platform for engineering human organs and nonhuman primate cells (interspecies chimeras) in large animals (Wu et al., 2016; Garry and Garry, 2019; Fu et al., 2020; Garry and Garry, 2021). For example, a gene edited pig served as a donor for the first porcine cardiac xenotransplantation which was performed early in 2022 (Graham, 2022; Griffith et al., 2022; Shah and Han, 2022). The recipient of the cardiac xenotransplant survived approximately 2 months and provides a platform for future clinical studies using these gene edited nonhuman organs that would serve as an unlimited organ source for those with terminal diseases (Griffith et al., 2022).
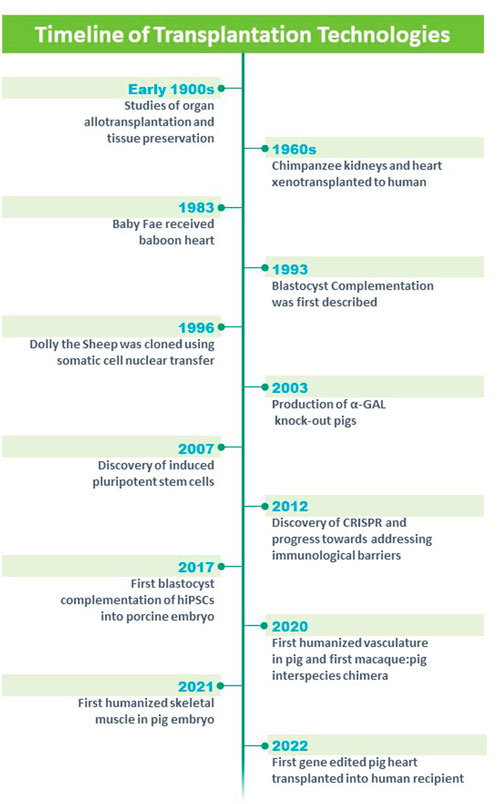
FIGURE 1. Timeline of technologies and advances in the field of solid organ transplantation. Modified from https://blogs.ubc.ca/xenotransplantationandethics/sample-page/.
Here, we provide a review of the studies related to cardiac xenotransplantation and blastocyst complementation in the porcine animal model as potential strategies to engineer a suitable organ for transplantation that will have longterm function and decreased requirements for immunosuppressive agents. Additionally, we will highlight the opportunities and the challenges for each of the strategies with the overall goal of engineering organs for research opportunities and clinical treatments.
Cardiac xenotransplantation: Historical perspective and recent successes
Xenotransplantation has been examined using an array of organs including heart, kidney, vasculature, skin, pancreas, islets and other organs. Alexis Carrel, M.D. was an early pioneer whose research focused on the surgical repair of the vasculature in the early 1900s (Carrel, 1907). Carrel used his knowledge of the vasculature and applied it to the transplant of arteries, veins, kidneys and hearts (heterotopically), limbs and endocrine glands (Carrel and Guthrie, 1905; Carrel, 1912; Carrel, 1914). Further, he focused on tissue preservation and oxygenation of tissues (Carrel, 1907). Collectively, his studies earned him the Nobel Prize in 1912 and these studies provided a platform for allograft transplantation and xenotransplantation (Figure 1).
James D. Hardy undertook the first xenotransplantation using an undersized nonhuman primate heart into a 68 year old male patient who had severe vasculopathy and was supported with mechanical ventilation (Hardy et al., 1964; Cooper, 2012; Cooper et al., 2015) (Figure 1). While the xenotransplanted heart was technically successful, the patient expired in the operating room after 90 min of support, in part, due to the undersized donor organ and rejection.
There was a considerable hiatus for the field of cardiac xenotransplantation until Dr. Leonard Bailey at Loma Linda Hospital undertook a heroic effort by implanting a baboon heart into the newborn Baby Fae who had hypoplastic left heart syndrome (Bailey et al., 1985; Stafford, 2019) (Figure 1). Baby Fae survived for 21 days and ultimately the graft failure was secondary to xenograft rejection (despite the use of immunosuppression agents). These early studies emphasized the importance of the vasculature which was the source of hyperacute rejection and chronic rejection of the xenograft. Emerging technologies began to revolutionize the field. Keith Campbell and Ian Wilmut’s team at the Roslin Institute successfully cloned Dolly who lived from 5 July 1996 to 14 February 2003 and whose birth and lifespan was a milestone for mammalian cloning technology (Campbell et al., 1996; Wilmut et al., 1997; Wilmut et al., 2007) (Figure 1). Further, the advent of gene editing (Jinek et al., 2012; Cong et al., 2013; Doudna and Charpentier, 2014) and reprogramming technologies (using Yamanaka factors (Takahashi and Yamanaka, 2006; Takahashi et al., 2007); provided important platforms for genetically engineering large animal models such as swine (Figure 1). Using these technologies, investigators genetically modified domestic pigs by deleting genes that contributed to hyperacute rejection of the xenotransplanted organ (α-1,3-galactosyltransferase or GGTA1, β-1,4-N-acetylgalactosaminyltransferase two or B4GalNT2 and Cytidine monophosphate-N-acetylneuraminic acid hydroxylase or CMAH) and Growth hormone receptor to prevent xenograft overgrowth (Kolber-Simonds et al., 2004; Ahrens et al., 2015; Choi et al., 2017; Sykes and Sachs, 2019; Goerlich et al., 2021; Hinrichs et al., 2021; Reichart et al., 2021) (Figure 1). Further genetic modifications included transgenic overexpression of human proteins to decrease cellular injury (CD47 and Hemoxygenase 1) (Petersen et al., 2010; Ahrens et al., 2015; Cooper et al., 2018), complement activation (hCD55 and hCD46) (Fischer et al., 2016) and coagulopathies (human Endothelial Cell Protein C Receptor and human Thrombomodulin) (Oropeza et al., 2009; Petersen et al., 2009). For the most part (with the exception of the Growth hormone receptor knockout), these genetic modifications targeted the porcine vasculature as these endothelial antigens have been shown to promote hyperacute rejection, delayed xenograft rejection, antibody mediated rejection and chronic xenograft rejection. The hearts from these gene edited pigs, were xenotransplanted into baboons in either a heterotopic position (to assess for rejection) (Lin et al., 1998; Hisashi et al., 2008; Iwase et al., 2015; Goerlich et al., 2020) or in the orthotopic position (to assess for functional performance) (Schmoeckel et al., 1998; Waterworth et al., 1998; Goerlich et al., 2021; Litovsky et al., 2022) (Figure 1). These xenografts performed well and these preclinical studies served as a platform for human studies. On 7 January 2022, a patient with endstage heart failure who was supported by extracorporeal membrane oxygenation (ECMO) received FDA approval for compassionate care use of a cardiac xenotransplantation from a gene edited pig (Figure 1) (Griffith et al., 2022; Pelc and Smiley, 2022; Reardon, 2022). The cardiac xenotransplant surgical procedure was successful although the patient had several complications including aortic dissection, acute kidney injury, thrombocytopenia and infections. The patient survived approximately 2 months, was the longest living survivor of cardiac xenotransplantation and was the world’s first human to receive a porcine heart (Griffith et al., 2022). These efforts have provided an important platform for other strategies aimed at engineering organs for transplantation therapies.
The use of miniature swine for the production of human organs
Miniature swine have been utilized extensively as biomedical research models of human disease due to their comparable size to humans, docile behavior, and slower growth curves when compared to domestic swine (Table 1). Various breeds including the Hanford (Ho et al., 2010), Sinclair/Hormel/Minnesota Mini (Misfeldt and Grimm, 1994), Yucatan (Panepinto and Phillips, 1986) and Gottingen (Khan et al., 2018) have been bred and each have distinct advantages and uses (Table 2). The use of miniature swine for the production of human organs is equally attractive for the reasons cited above but also for the relatively short gestational times and the ability to use inbred strains such as MGH defined MHC factors (Sykes and Sachs, 2019) and where isolation and breeding techniques can eliminate zoonotic viruses.
SCNT and blastocyst complementation to produce exogenic organs
Previous studies have demonstrated that the deletion of genes to produce a mouse embryo that lacks an entire lineage or organ can be completely rescued using blastocyst complementation and pluripotent embryonic stem cells from a different species. For example, genetic mouse studies demonstrated that the deletion of Pdx1 (the master regulator for the pancreas) resulted in a mouse that lacked a pancreas and died early following birth (Offield et al., 1996). Using blastocyst complementation, the Pdx1 mutant mouse blastocyst was completely rescued using GFP-labeled rat embryonic stem cells (ESCs) and produced a viable mouse with a GFP-labeled pancreas (reflecting that all the pancreatic cells were derivatives of the GFP-labeled rat ESCs) (Kobayashi et al., 2010). Similarly, blastocyst complementation was undertaken with the Pdx1 null rat blastocyst and the GFP-labeled mouse ESCs (Yamaguchi et al., 2017). The resulting rat was viable and had a GFP-labeled pancreas. These studies demonstrated the importance of the depleted organ niche, the efficiency and feasibility of blastocyst complementation and that the host embryo determined the growth and the size of complemented organ (i.e. the size of the pancreas which was derived from mouse ESCs was equal to the size of a rat pancreas and not the size of a mouse pancreas). These successes provided the rationale for interspecies complementation using larger animal models.
The first challenge for the field was to define an appropriate large animal model. Previously, baboons, sheep, cows, chimpanzees, pigs and others have served as donors or recipients for xenotransplantation (Crick et al., 1998; Cooper, 2012; Cooper et al., 2015; Sachs, 2017). Each of these large animal models have their strengths and limitations. Increasingly, the pig has been used for these types of studies as they have a number of characteristics including: availability, comparable size to humans, large litter size, relatively short gestational periods and they can be housed within barrier facilities and screened for multiple pathogens. Further characteristics associated with the porcine animal model are highlighted in Table 3.
The Nakauchi laboratory generated the first large animal exogenic organ by using blastocyst complementation to deliver allogenic porcine blastomeres to a pancreas disabled porcine (Matsunari et al., 2013). These studies demonstrated the generation of functional pancreata in lineage disabled pigs (Matsurnari et al., 2013). Additionally, the rescue of pigs that were lacking kidneys (Matsunari et al., 2020), livers (Matsunari et al., 2020), vasculature and blood (Das et al., 2020) or pancreas/vasculature/blood (Matsunari et al., 2020) was demonstrated using allogenic blastocyst complementation (Das et al., 2020; Matsunari et al., 2020) or with blastocyst complementation of human cells (Das et al., 2020). These papers provided a platform for the deletion and rescue of the vasculature and blood (either alone or in addition to other lineages) within a chimera which may be essential for the transplantation of an organ without rejection.
The discovery of hiPSCs (Takahashi and Yamanaka, 2006; Takahashi et al., 2007) (Figure 1) facilitated the ability to perform blastocyst complementation using human cells. Accordingly, the Belmonte laboratory delivered various types of hiPSCs in WT porcine parthenotes which were, ultimately, delivered to surrogate animals (Wu et al., 2017). Early stage analysis of these chimeric embryos revealed that the donor stem cells were localized throughout the embryo (using lineage specific antisera) (Wu et al., 2017). The authors concluded that while these technologies were successful, the efficiency of the chimerism was limited.
Engineering human hematoendothelial lineages using blastocyst complementation
Our laboratories targeted master regulators and pioneer factors that regulated specific lineages and used gene editing technologies to produce organ depleted porcine embryos that would serve as recipients for blastocyst complementation of donor stem cells. Initially, studies focused on the vasculature as this organ has been shown to harbor cell surface receptors/proteins that promote hyperacute rejection, antibody mediated rejection and chronic rejection (Auchincloss and Sachs, 1998; Sykes and Sachs, 2019). Previous studies using independent screens identified ETV2 and global deletion of this gene resulted in nonviable mouse embryos (E8.5-E9.5) that lacked vasculature and blood (Rasmussen et al., 2012; Rasmussen et al., 2013; Shi et al., 2014; Shi et al., 2015; Garry, 2016; Gong et al., 2017; Koyano-Nakagawa and Garry, 2017; Singh et al., 2020). Additional studies, using zebrafish, xenopus and mice, defined upstream regulators, downstream regulators and further defined roles in the regulation of cell proliferation, cell migration (Chan et al., 2013; Craig et al., 2015; Singh et al., 2017; Gong et al., 2022) and we have identified ETV2 as a pioneer factor for development of the hematoendothelial lineage (Gong et al., 2022). Furthermore, the genetic deletion of the 3.9 kb upstream fragment of the Etv2 gene, which harbors the promoter and enhancer modules, phenocopied the global deletion of Etv2 supporting the notion that this fragment contained all the regulatory modules for Etv2 gene regulation (Koyano-Nakagawa et al., 2015). Deletion of ETV2 in the pig also resulted in the absence of vasculature and blood and the mutant embryos were nonviable by E16/E17 (Das et al., 2020). Having established an organ (vasculature) depleted porcine model, SCNT and blastocyst complementation using GFP-labeled pig stem cells resulted in the complete rescue of the ETV2 null phenotype and viable intraspecies chimeric pigs with GFP-labeled vasculature and blood (Das et al., 2020). These intraspecies chimeric studies represent an important accomplishment for interspecies blastocyst complementation. Two GFP-labeled hiPSCs were delivered into the four to six cell ETV2 null porcine embryo and these interspecies chimeras were transferred to surrogate gilts. Analysis of the chimeric embryos revealed that the hiPSCs rescued the lethal phenotype and differentiated to the endothelial lineage (Figure 1) (Das et al., 2020). Importantly, no hiPSCs or human derivatives were found outside of the hematoendothelial lineages. These studies demonstrated the merit for using an organ depleted strategy (to establish a niche for stem cell homing) in a large animal model. Future studies will be necessary to examine later embryonic development of the interspecies chimeras and the production of a viable human-pig chimera that has a human vasculature.
Engineering human muscle using blastocyst complementation
Previous studies have demonstrated the essential role of the basic helix-loop-helix (bHLH) MYOD family members (MYF5, MYOD, Myogenin and MRF4 also known as MYF6) as master regulators or fundamental determinants for the myogenic lineage (Hasty et al., 1993; Rudnicki et al., 1993; Rawls et al., 1995; Rawls et al., 1998). Elegant studies using the mouse model demonstrated that these myogenic regulatory factors revealed functional redundancy (Rawls et al., 1995; Tajbakhsh et al., 1997; Rawls et al., 1998; Valdez et al., 2000; Kassar-Duchossoy et al., 2004). Using CRISPR/Cas9 gene editing, porcine fibroblasts were genetically modified with MYF5/MYOD/MYF6 multiplex deletions (Maeng et al., 2021) (Figure 2). These mutant porcine embryos were found to lack myogenic progenitors and the skeletal muscle lineage. This mutant phenotype was completely rescued using blastocyst complementation with GFP-labeled pig stem cells (Maeng et al., 2021). These intraspecies chimeras were viable, ambulatory and indistinguishable from the controls. The skeletal muscle from these intraspecies chimeras was fully developed, labeled with GFP (demonstrating that the myofibers were derivatives of the exogenous GFP-labeled porcine stem cells). The chimeric skeletal muscle had a normal fiber type distribution and satellite cell distribution which were resident within the muscle. Furthermore, the physiological response to stimuli were comparable between controls and the intraspecies chimeras (Maeng et al., 2021). Using blastocyst complementation and GFP-labeled hiPSCs as donor cells, the interspecies chimeras were delivered into surrogate gilts and analyzed at two developmental stages. The analyses of these interspecies chimeras used wholemount immunofluorescence, immunohistochemical, sequence analysis (to confirm the presence of human members of the MYOD family and the absence of porcine proteins) and PCR techniques (Maeng et al., 2021). These studies demonstrate that the myotome of the somite was repopulated with human myogenic progenitors that expressed human MYOD and human MYF5. Furthermore, these human myogenic progenitors migrated to the limbs and formed human skeletal muscle. Importantly, a rigorous analysis was performed and demonstrated that no human stem cells or derivatives were located in the brain or the gametes of the chimeric embryos. Collectively, these studies demonstrated the benefits of establishing a muscle depleted embryo as a recipient for engineering the interspecies chimeras (Maeng et al., 2021).
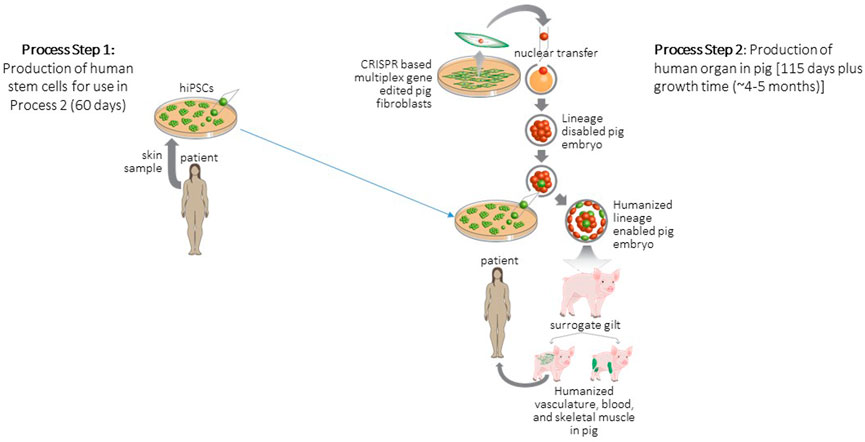
FIGURE 2. Strategy for generation and transplantation of human organ engineered in pig. Process Step 1: Biopsies are collected from patients to derive hiPSCs. Process Step 2: Porcine embryonic fibroblasts are gene edited to delete a lineage following which these fibroblasts are cloned to produce null porcine embryos. Blastocyst complementation is used to deliver patient derived hiPSCs to null embryos following which the complemented embryos are surgically transferred to a surrogate animal. The offspring of the surrogate develops a human organ to replace the deleted lineage. These human organs are then harvested and transplanted to the patient. Figure modified from (Garry and Garry, 2021).
Strategies for enhanced efficiencies for the generation of interspecies chimeras
Interspecies chimera production is highly dependent on a number of factors. One factor is the evolutionary distance between the donor cells and the recipient embryo. We know that rat-mouse interspecies chimera formation is relatively efficient and produces viable offspring following blastocyst complementation. The evolutionary distance between rat and mouse species is approximately 25–35 million years (Makalowski and Boguski, 1998). In contrast, the evolutionary distance between humans and mice (rodents) is approximately 95 million years (Makalowski and Boguski, 1998). Likewise, the distance of a common ancestor between humans and pigs is estimated to be more than 90 million years ago (Wei et al., 2018). This divergence reflects the genetic similarity between the respective species. Another factor may be the survival of the donor cells following blastocyst complementation. Studies undertaken in several laboratories have demonstrated the benefit of genetic modification of donor cells to promote cell survival. For example, stem cells that overexpress B-cell lymphoma 2 (BCL2) and/or MYCN have shown increased efficiency of chimera production (Labi et al., 2013; Wang et al., 2018; Das et al., 2020; Zhu et al., 2022). These studies demonstrate that programmed cell death related to evolutionary divergent donor cells is an important factor or barrier. While a clear benefit has been demonstrated, chronic overexpression of BCL2 may be associated with a hypercellular state. Alternatively, strategies have demonstrated that genetic deletion of TP53 or Igfr1 in the donor cell population also results in increased efficiency of interspecies chimera production (Maeng et al., 2021). This strategy increased the proliferation of the donor cell population and may facilitate the reprogramming and integration to the recipient embryo. A third strategy has focused on enhancement of donor cell-recipient cell interconnections and communication, metabolic transition, trophectoderm development and cell surface proteins all of which have been shown to impact the efficiency of interspecies chimeras (Zheng et al., 2021). These latter studies have used single cell RNA-seq to define distinct, species-specific molecular programs at various times during early embryogenesis (Gong et al., 2017; Cutter et al., 2019; Das et al., 2020; Lu et al., 2021; Maeng et al., 2021). These studies further demonstrated the species-specific link between the metabolic state and epigenetic and transcriptional gene regulation in the pre-gastrulation embryo and highlighted targets that may be modified in future experiments in order to achieve increased efficiency of primate-porcine chimeras (Theunissen and Jaenisch, 2017; Lu et al., 2021).
Future studies focused on interspecies chimeras
While initial studies have engineered human-porcine chimeras using an organ-depleted strategy in the porcine recipient, future studies will focus on modifications to enhance the efficiency of interspecies chimeras. Establishment of a developmental niche in the recipient, resulted in successful complementation (Matsunari et al., 2013; Das et al., 2020; Maeng et al., 2021; Nishimura et al., 2021) without the contribution of human stem cells or human derivatives to outside tissues/lineages such as the brain or gametes (Das et al., 2020; Maeng et al., 2021). Future studies using other developmental niche strategies and other human stem cell populations (naïve vs primed vs intermediate hiPSCs) (Wu et al., 2017) will also need to undertake an assessment to examine human cell localization (using immunohistochemistry and PCR) in the embryonic recipient brain and gametes. These quantitative studies will add to the study results that have already been published. Moreover, later stage human-porcine chimeras should be evaluated and characterized using morphological, physiological and molecular (scRNA-seq, ATAC-seq, etc.) techniques to assess developmental progression and the comparison of the chimeric organ to the porcine organ. The immunological status and the response of the recipient animal model to the hiPSCs and their derivatives should also be evaluated. The absence of an immunological response by the porcine recipient following blastocyst complementation using GFP-labeled porcine blastomeres supports the notion that tolerance is established in the resulting chimera (Das et al., 2020; Maeng et al., 2021). Future studies will need to dissect the mechanisms associated with the immunological response or tolerance of the recipient animal model to the interspecies (i.e. human-porcine) chimera. The definition of these mechanisms will be an important advance for the field and serve as a platform for studies that will examine the impact of these chimeric organs as a donor supply for patients with chronic and terminal diseases.
Conclusion
More than 100,000 Americans are awaiting solid organ transplantation and are on the national transplant waiting list. Many more adults could benefit from such curative therapies but due to limited number of donor organs such therapy is not broadly available. Using gene editing technology primarily aimed at the genetic modification of the porcine vasculature, the first porcine cardiac xenotransplant was performed early in 2022. These successes have also paved the path for the generation of human-porcine chimeric organs. Using a gene deletion strategy and blastocyst complementation, human vasculature and human skeletal muscle have been engineered and early chimeric embryos have been examined. Additional studies are warranted that will focus on the developmental progression of the human-porcine chimeric organs, immunological response/tolerance of the recipient animal model, strategies aimed at increased efficiency of interspecies chimeras and the comprehensive characterization of the human chimeras. Collectively, these early studies have generated tremendous enthusiasm and excitement focused on the opportunities to cure endstage diseases and democratize organ transplantation using xenografts and exogenic chimeric organs.
Author contributions
Conceptualization, MG and DG Manuscript preparation and writing: Y-HC, JS, MG, and DG. All authors approved the manuscript.
Funding
This work was supported by the NIH (HL144582 and HL160476), RMM (101617-DS-003) and DOD. The authors acknowledge the efforts of Cynthia Faraday for figure preparation.
Conflict of interest
DG and MG are cofounders of NorthStar Genomics.
The remaining authors declare that the research was conducted in the absence of any commercial or financial relationships that could be construed as a potential conflict of interest.
Publisher’s note
All claims expressed in this article are solely those of the authors and do not necessarily represent those of their affiliated organizations, or those of the publisher, the editors and the reviewers. Any product that may be evaluated in this article, or claim that may be made by its manufacturer, is not guaranteed or endorsed by the publisher.
References
Ahrens, H. E., Petersen, B., Ramackers, W., Petkov, S., Herrmann, D., Hauschild-Quintern, J., et al. (2015). Kidneys from α1, 3-galactosyltransferase knockout/human heme oxygenase-1/human A20 transgenic pigs are protected from rejection during ex vivo perfusion with human blood. Transpl. Direct 1, e23. doi:10.1097/TXD.0000000000000533
Auchincloss, H., and Sachs, D. H. (1998). Xenogeneic transplantation. Annu. Rev. Immunol. 16, 433–470. doi:10.1146/annurev.immunol.16.1.433
Bailey, L. L., Nehlsen-Cannarella, S. L., Concepcion, W., and Jolley, W. B. (1985). Baboon-to-human cardiac xenotransplantation in a neonate. Jama 254, 3321–3329. doi:10.1001/jama.1985.03360230053022
Bouchard, G. F., Brown, L. D., Liu, J., Shoemake, C., Brocksmith, D., Trickey, J., et al. (2019). “Minature swine uses in biomedical research,” in Sinclair bio resources. Editor L. SINCLAIR RESEARCH. Minature Swine Book of Normal Data.
Campbell, K. H., Mcwhir, J., Ritchie, W. A., and Wilmut, I. (1996). Sheep cloned by nuclear transfer from a cultured cell line. Nature 380, 64–66. doi:10.1038/380064a0
Carrel, A., and Guthrie, C. C. (1905). The university of Florida. Funct. a transplanted kidney Sci. 22, 473–474. doi:10.1126/science.22.563.473-a
Carrel, A. (1907). Heterotransplantation of blood vessels preserved in cold storage. J. Exp. Med. 9, 226–228. doi:10.1084/jem.9.2.226
Carrel, A. (1914). Present condition of A strain of connective tissue twenty-eight months old. Exp. operations orifices heart Ann Surg 60, 1–2. doi:10.1084/jem.20.1.1
Carrel, A. (1912). Ultimate results of aortic transplantations. J. Exp. Med. 15, 389–392. doi:10.1084/jem.15.4.389
Chan, S. S., Shi, X., Toyama, A., Arpke, R. W., Dandapat, A., Iacovino, M., et al. (2013). Mesp1 patterns mesoderm into cardiac, hematopoietic, or skeletal myogenic progenitors in a context-dependent manner. Cell Stem Cell 12, 587–601. doi:10.1016/j.stem.2013.03.004
Choi, K., Shim, J., Ko, N., Eom, H., Kim, J., Lee, J.-W., et al. (2017). Production of heterozygous alpha 1, 3-galactosyltransferase (GGTA1) knock-out transgenic miniature pigs expressing human CD39. Transgenic Res. 26, 209–224. doi:10.1007/s11248-016-9996-7
Cong, L., Ran, F. A., Cox, D., Lin, S., Barretto, R., Habib, N., et al. (2013). Multiplex genome engineering using CRISPR/Cas systems. Science 339, 819–823. doi:10.1126/science.1231143
Cooper, D. K. (2012). A brief history of cross-species organ transplantation. Proc.(Bayl Univ. Med. Cent. 25, 49–57. doi:10.1080/08998280.2012.11928783
Cooper, D. K. C., Ekser, B., and Tector, A. J. (2015). A brief history of clinical xenotransplantation. Int. J. Surg. 23, 205–210. doi:10.1016/j.ijsu.2015.06.060
Cooper, D. K. C., Ezzelarab, M., Iwase, H., and Hara, H. (2018). Perspectives on the optimal genetically engineered pig in 2018 for initial clinical trials of kidney or heart xenotransplantation. Transplantation 102, 1974–1982. doi:10.1097/TP.0000000000002443
Corona, B. T., Rivera, J. C., Owens, J. G., Wenke, J. C., and Rathbone, C. R. (2015). Volumetric muscle loss leads to permanent disability following extremity trauma. J. Rehabil. Res. Dev. 52, 785–792. doi:10.1682/JRRD.2014.07.0165
Craig, M. P., Grajevskaja, V., Liao, H. K., Balciuniene, J., Ekker, S. C., Park, J. S., et al. (2015). Etv2 and fli1b function together as key regulators of vasculogenesis and angiogenesis. Arterioscler. Thromb. Vasc. Biol. 35, 865–876. doi:10.1161/ATVBAHA.114.304768
Crick, S. J., Sheppard, M. N., Ho, S. Y., Gebstein, L., and Anderson, R. H. (1998). Anatomy of the pig heart: Comparisons with normal human cardiac structure. J. Anat. 193 (1), 105–119. doi:10.1046/j.1469-7580.1998.19310105.x
Cutter, A. D., Garrett, R. H., Mark, S., Wang, W., and Sun, L. (2019). Molecular evolution across developmental time reveals rapid divergence in early embryogenesis. Evol. Lett. 3, 359–373. doi:10.1002/evl3.122
Das, S., Koyano-Nakagawa, N., Gafni, O., Maeng, G., Singh, B. N., Rasmussen, T., et al. (2020). Generation of human endothelium in pig embryos deficient in ETV2. Nat. Biotechnol. 38, 297–302. doi:10.1038/s41587-019-0373-y
Doudna, J. A., and Charpentier, E. (2014). Genome editing. The new frontier of genome engineering with CRISPR-Cas9. Science 346, 1258096. doi:10.1126/science.1258096
Fischer, K., Kraner-Scheiber, S., Petersen, B., Rieblinger, B., Buermann, A., Flisikowska, T., et al. (2016). Efficient production of multi-modified pigs for xenotransplantation by 'combineering', gene stacking and gene editing. Sci. Rep. 6, 29081. doi:10.1038/srep29081
Fu, R., Yu, D., Ren, J., Li, C., Wang, J., Feng, G., et al. (2020). Domesticated cynomolgus monkey embryonic stem cells allow the generation of neonatal interspecies chimeric pigs. Protein Cell 11, 97–107. doi:10.1007/s13238-019-00676-8
Galli, C., and Lazzari, G. (2021). 25th Anniversary of Cloning by Somatic-Cell Nuclear Transfer: Current applications of SCNT in advanced breeding and genome editing in livestock. Reproduction 162, F23–f32. doi:10.1530/REP-21-0006
Garg, S., Singh, P., Sharma, A., and Gupta, G. (2013). A gross comparative anatomical study of hearts in human cadavers and pigs. IJMDS 2 (2).
Garry, D. J. (2016). Etv2 is a master regulator of hematoendothelial lineages. Trans. Am. Clin. Climatol. Assoc. 127, 212–223.
Garry, D. J., and Garry, M. G. (2019). Interspecies chimeras and the generation of humanized organs. Circ. Res. 124, 23–25. doi:10.1161/CIRCRESAHA.118.314189
Garry, D. J., and Garry, M. G. (2021). Interspecies chimeras as a platform for exogenic organ production and transplantation. Exp. Biol. Med. 246, 1838–1844. doi:10.1177/15353702211024948
Garry, D. J., Goetsch, S. C., Mcgrath, A. J., and Mammen, P. P. (2005). Alternative therapies for orthotopic heart transplantation. Am. J. Med. Sci. 330, 88–101. doi:10.1097/00000441-200508000-00006
Goerlich, C. E., Dichiacchio, L., Zhang, T., Singh, A. K., Lewis, B., Tatarov, I., et al. (2020). Heterotopic porcine cardiac xenotransplantation in the intra-abdominal position in a non-human primate model. Sci. Rep. 10, 10709. doi:10.1038/s41598-020-66430-x
Goerlich, C. E., Griffith, B., Hanna, P., Hong, S. N., Ayares, D., Singh, A. K., et al. (2021). The growth of xenotransplanted hearts can be reduced with growth hormone receptor knockout pig donors. J. Thorac. Cardiovasc. Surg. S0022, 01261. doi:10.1016/j.jtcvs.2021.07.051
Gong, W., Das, S., Sierra-Pagan, J., Skie, E., Dsouza, N., Larson, T., et al. (2022). ETV2 functions as a pioneer factor to regulate and reprogram the endothelial lineage. Nat. Cell Biol. 24, 672–684. In Press. doi:10.1038/s41556-022-00901-3
Gong, W., Rasmussen, T. L., Singh, B. N., Koyano-Nakagawa, N., Pan, W., and Garry, D. J. (2017). Dpath software reveals hierarchical haemato-endothelial lineages of Etv2 progenitors based on single-cell transcriptome analysis. Nat. Commun. 8, 14362. doi:10.1038/ncomms14362
Graham, A. (2022). Blacksburg company raised genetically modified pig for first heart transplant in human. Roanoke, VA: The Roanoke Times.
Greising, S. M., Dearth, C. L., and Corona, B. T. (2016). Regenerative and rehabilitative medicine: A necessary synergy for functional recovery from volumetric muscle loss injury. Cells Tissues Organs 202, 237–249. doi:10.1159/000444673
Greising, S. M., Rivera, J. C., Goldman, S. M., Watts, A., Aguilar, C. A., and Corona, B. T. (2017). Unwavering pathobiology of volumetric muscle loss injury. Sci. Rep. 7, 13179. doi:10.1038/s41598-017-13306-2
Griffith, B. P., Goerlich, C. E., Singh, A. K., Rothblatt, M., Lau, C. L., Shah, A., et al. (2022). Genetically modified porcine-to-human cardiac xenotransplantation. N. Engl. J. Med. 387, 35–44. doi:10.1056/NEJMoa2201422
Gutierrez, K., Dicks, N., Glanzner, W. G., Agellon, L. B., and Bordignon, V. (2015). Efficacy of the porcine species in biomedical research. Front. Genet. 6, 293. doi:10.3389/fgene.2015.00293
Hardy, J. D., Chavez, C. M., Kurrus, F. D., Neely, W. A., Eraslan, S., Turner, M. D., et al. (1964). Heart transplantation in man: Developmental studies and report of a case. Jama 188, 1132–1140. doi:10.1001/jama.1964.03060390034008
Hasty, P., Bradley, A., Morris, J. H., Edmondson, D. G., Venuti, J. M., Olson, E. N., et al. (1993). Muscle deficiency and neonatal death in mice with a targeted mutation in the myogenin gene. Nature 364, 501–506. doi:10.1038/364501a0
Hinrichs, A., Riedel, E. O., Klymiuk, N., Blutke, A., Kemter, E., Längin, M., et al. (2021). Growth hormone receptor knockout to reduce the size of donor pigs for preclinical xenotransplantation studies. Xenotransplantation 28, e12664. doi:10.1111/xen.12664
Hisashi, Y., Yamada, K., Kuwaki, K., Tseng, Y. L., Dor, F. J. M. F., Houser, S. L., et al. (2008). Rejection of cardiac xenografts transplanted from alpha1, 3-galactosyltransferase gene-knockout (GalT-KO) pigs to baboons. Am. J. Transpl. 8, 2516–2526. doi:10.1111/j.1600-6143.2008.02444.x
Ho, C. S., Martens, G. W., Amoss, M. S., Gomez-Raya, L., Beattie, C. W., and Smith, D. M. (2010). Swine leukocyte antigen (SLA) diversity in Sinclair and Hanford swine. Dev. Comp. Immunol. 34 (3), 250–257. doi:10.1016/j.dci.2009.09.006
Iwase, H., Ekser, B., Satyananda, V., Bhama, J., Hara, H., Ezzelarab, M., et al. (2015). Pig-to-baboon heterotopic heart transplantation–exploratory preliminary experience with pigs transgenic for human thrombomodulin and comparison of three costimulation blockade-based regimens. Xenotransplantation 22, 211–220. doi:10.1111/xen.12167
Jinek, M., Chylinski, K., Fonfara, I., Hauer, M., Doudna, J. A., and Charpentier, E. (2012). A programmable dual-RNA-guided DNA endonuclease in adaptive bacterial immunity. Science 337, 816–821. doi:10.1126/science.1225829
Kassar-Duchossoy, L., Gayraud-Morel, B., Gomes, D., Rocancourt, D., Buckingham, M., Shinin, V., et al. (2004). Mrf4 determines skeletal muscle identity in Myf5:Myod double-mutant mice. Nature 431, 466–471. doi:10.1038/nature02876
Khan, A., Waqar, K., Shafique, A., Irfan, R., and Gul, A. (2018). “Chapter 18 - in vitro and in vivo animal models: The engineering towards understanding human diseases and therapeutic interventions,” in Omics technologies and bio-engineering. Editors D. BARH, and V. AZEVEDO (United States: Academic Press).
Kobayashi, T., Yamaguchi, T., Hamanaka, S., Kato-Itoh, M., Yamazaki, Y., Ibata, M., et al. (2010). Generation of rat pancreas in mouse by interspecific blastocyst injection of pluripotent stem cells. Cell 142, 787–799. doi:10.1016/j.cell.2010.07.039
Kolber-Simonds, D., Lai, L., Watt, S. R., Denaro, M., Arn, S., Augenstein, M. L., et al. (2004). Production of alpha-1, 3-galactosyltransferase null pigs by means of nuclear transfer with fibroblasts bearing loss of heterozygosity mutations. Proc. Natl. Acad. Sci. U. S. A. 101, 7335–7340. doi:10.1073/pnas.0307819101
Koyano-Nakagawa, N., and Garry, D. J. (2017). Etv2 as an essential regulator of mesodermal lineage development. Cardiovasc. Res. 113, 1294–1306. doi:10.1093/cvr/cvx133
Koyano-Nakagawa, N., Shi, X., Rasmussen, T. L., Das, S., Walter, C. A., and Garry, D. J. (2015). Feedback mechanisms regulate ets variant 2 (Etv2) gene expression and hematoendothelial lineages. J. Biol. Chem. 290, 28107–28119. doi:10.1074/jbc.M115.662197
Labi, V., Bertele, D., Woess, C., Tischner, D., Bock, F. J., Schwemmers, S., et al. (2013). Haematopoietic stem cell survival and transplantation efficacy is limited by the BH3-only proteins Bim and Bmf. EMBO Mol. Med. 5, 122–136. doi:10.1002/emmm.201201235
Lai, L., Kolber-Simonds, D., Park, K. W., Cheong, H. T., Greenstein, J. L., Im, G. S., et al. (2002). Production of alpha-1, 3-galactosyltransferase knockout pigs by nuclear transfer cloning. Science 295, 1089–1092. doi:10.1126/science.1068228
Lin, S. S., Weidner, B. C., Byrne, G. W., Diamond, L. E., Lawson, J. H., Hoopes, C. W., et al. (1998). The role of antibodies in acute vascular rejection of pig-to-baboon cardiac transplants. J. Clin. Invest. 101, 1745–1756. doi:10.1172/JCI2134
Litovsky, S. H., Foote, J. B., Jagdale, A., Walcott, G., Iwase, H., Bikhet, M. H., et al. (2022). Cardiac and pulmonary histopathology in baboons following genetically-engineered pig orthotopic heart transplantation. Ann. Transpl. 27, e935338. doi:10.12659/AOT.935338
Lu, X., Zhang, Y., Wang, L., Wang, L., Wang, H., Xu, Q., et al. (2021). Evolutionary epigenomic analyses in mammalian early embryos reveal species-specific innovations and conserved principles of imprinting. Sci. Adv. 7, eabi6178. doi:10.1126/sciadv.abi6178
Lunney, J. K., Van Goor, A., Walker, K. E., Hailstock, T., Franklin, J., and Dai, C. (2021). Importance of the pig as a human biomedical model. Sci. Transl. Med. 13 (621), eabd5758. doi:10.1126/scitranslmed.abd5758
Maeng, G., Das, S., Greising, S. M., Gong, W., Singh, B. N., Kren, S., et al. (2021). Humanized skeletal muscle in MYF5/MYOD/MYF6-null pig embryos. Nat. Biomed. Eng. 5, 805–814. doi:10.1038/s41551-021-00693-1
Makalowski, W., and Boguski, M. S. (1998). Evolutionary parameters of the transcribed mammalian genome: An analysis of 2, 820 orthologous rodent and human sequences. Proc. Natl. Acad. Sci. U. S. A. 95, 9407–9412. doi:10.1073/pnas.95.16.9407
Matsunari, H., Nagashima, H., Watanabe, M., Umeyama, K., Nakano, K., Nagaya, M., et al. (2013). Blastocyst complementation generates exogenic pancreas in vivo in apancreatic cloned pigs. Proc. Natl. Acad. Sci. U. S. A. 110, 4557–4562. doi:10.1073/pnas.1222902110
Matsunari, H., Watanabe, M., Hasegawa, K., Uchikura, A., Nakano, K., Umeyama, K., et al. (2020). Compensation of disabled organogeneses in genetically modified pig fetuses by blastocyst complementation. Stem Cell Rep. 14, 21–33. doi:10.1016/j.stemcr.2019.11.008
Misfeldt, M. L., and Grimm, D. R. (1994). Sinclair miniature swine: An animal model of human melanoma. Vet. Immunol. Immunopathol. 43 (1–3), 167–175. doi:10.1016/0165-2427(94)90133-3
Nishimura, T., Suchy, F. P., Bhadury, J., Igarashi, K. J., Charlesworth, C. T., and Nakauchi, H. (2021). Generation of functional organs using a cell-competitive niche in intra- and inter-species rodent chimeras. Cell Stem Cell 28, 141–149.e3. e3. doi:10.1016/j.stem.2020.11.019
Offield, M. F., Jetton, T. L., Labosky, P. A., Ray, M., Stein, R. W., Magnuson, M. A., et al. (1996). PDX-1 is required for pancreatic outgrowth and differentiation of the rostral duodenum. Development 122, 983–995. doi:10.1242/dev.122.3.983
Oropeza, M., Petersen, B., Carnwath, J. W., Lucas-Hahn, A., Lemme, E., Hassel, P., et al. (2009). Transgenic expression of the human A20 gene in cloned pigs provides protection against apoptotic and inflammatory stimuli. Xenotransplantation 16, 522–534. doi:10.1111/j.1399-3089.2009.00556.x
Panepinto, L. M., and Phillips, R. W. (1986). The Yucatan miniature pig: Characterization and utilization in biomedical research. Lab Anim. Sci. 36 (4), 344–347.
Pelc, C., and Smiley, J. D. (2022). First successful pig-to-human heart transplant may offer new options for patients. Med. News Today. https://www.medicalnewstoday.com/articles/first-successful-pig-to-human-heart-transplant-may-offer-new-options-for-patients
Petersen, B., Lucas-Hahn, A., Lemme, E., Queisser, A. L., Oropeza, M., Herrmann, D., et al. (2010). Generation and characterization of pigs transgenic for human hemeoxygenase-1 (hHO-1). Xenotransplantation 17, 102–103. doi:10.1111/j.1399-3089.2010.00573_7.x
Petersen, B., Ramackers, W., Tiede, A., Lucas-Hahn, A., Herrmann, D., Barg-Kues, B., et al. (2009). Pigs transgenic for human thrombomodulin have elevated production of activated protein C. Xenotransplantation 16, 486–495. doi:10.1111/j.1399-3089.2009.00537.x
Pierson, R. N., 3R. D., Fishman, J. A., Lewis, G. D., D'Alessandro, D. A., Connolly, M. R., Burdorf, L., et al. (2020). Progress toward cardiac xenotransplantation. Circulation 142, 1389–1398. doi:10.1161/CIRCULATIONAHA.120.048186
Polejaeva, I. A., Chen, S. H., Vaught, T. D., Page, R. L., Mullins, J., Ball, S., et al. (2000). Cloned pigs produced by nuclear transfer from adult somatic cells. Nature 407, 86–90. doi:10.1038/35024082
Rasmussen, T. L., Martin, C. M., Walter, C. A., Shi, X., Perlingeiro, R., Koyano-Nakagawa, N., et al. (2013). Etv2 rescues Flk1 mutant embryoid bodies. Genesis 51, 471–480. doi:10.1002/dvg.22396
Rasmussen, T. L., Shi, X., Wallis, A., Kweon, J., Zirbes, K. M., Koyano-Nakagawa, N., et al. (2012). VEGF/Flk1 signaling cascade transactivates Etv2 gene expression. PLoS One 7, e50103. doi:10.1371/journal.pone.0050103
Rawls, A., Morris, J. H., Rudnicki, M., Braun, T., Arnold, H. H., Klein, W. H., et al. (1995). Myogenin's functions do not overlap with those of MyoD or Myf-5 during mouse embryogenesis. Dev. Biol. 172, 37–50. doi:10.1006/dbio.1995.0004
Rawls, A., Valdez, M. R., Zhang, W., Richardson, J., Klein, W. H., and Olson, E. N. (1998). Overlapping functions of the myogenic bHLH genes MRF4 and MyoD revealed in double mutant mice. Development 125, 2349–2358. doi:10.1242/dev.125.13.2349
Reardon, S. (2022). First pig-to-human heart transplant: What can scientists learn? Nature 601, 305–306. doi:10.1038/d41586-022-00111-9
Reichart, B., Längin, M., Denner, J., Schwinzer, R., Cowan, P. J., and Wolf, E. (2021). Pathways to clinical cardiac xenotransplantation. Transplantation 105, 1930–1943. doi:10.1097/TP.0000000000003588
Renner, S., Dobenecker, B., Blutke, A., Zӧls, S., Wanke, R., Ritzmann, M., et al. (2016). Comparative aspects of rodent and nonrodent animal models for mechanistic and translational diabetes research. Theriogenology 86 (1), 406–421. doi:10.1016/j.theriogenology.2016.04.055
Rudnicki, M. A., Schnegelsberg, P. N. J., Stead, R. H., Braun, T., Arnold, H.-H., and Jaenisch, R. (1993). MyoD or Myf-5 is required for the formation of skeletal muscle. Cell 75, 1351–1359. doi:10.1016/0092-8674(93)90621-v
Sachs, D. H. (2017). Immune tolerance, xenografts, and large-animal studies in transplantation. Ann. Am. Thorac. Soc. 14, S220–s225. doi:10.1513/AnnalsATS.201607-534MG
Schmoeckel, M., Bhatti, F. N., Zaidi, A., Cozzi, E., Waterworth, P. D., Tolan, M. J., et al. (1998). Orthotopic heart transplantation in a transgenic pig-to-primate model. Transplantation 65, 1570–1577. doi:10.1097/00007890-199806270-00006
Shah, A. M., and Han, J. J. (2022). First successful porcine to human heart transplantation performed in the United States. Artif. Organs 46, 543–545. doi:10.1111/aor.14203
Shi, X., Richard, J., Zirbes, K. M., Gong, W., Lin, G., Kyba, M., et al. (2014). Cooperative interaction of Etv2 and Gata2 regulates the development of endothelial and hematopoietic lineages. Dev. Biol. 389, 208–218. doi:10.1016/j.ydbio.2014.02.018
Shi, X., Zirbes, K. M., Rasmussen, T. L., Ferdous, A., Garry, M. G., Koyano-Nakagawa, N., et al. (2015). The transcription factor Mesp1 interacts with cAMP-responsive element binding protein 1 (Creb1) and coactivates Ets variant 2 (Etv2) gene expression. J. Biol. Chem. 290, 9614–9625. doi:10.1074/jbc.M114.614628
Singh, B. N., Sierra-Pagan, J. E., Gong, W., Das, S., Theisen, J. W. M., Skie, E., et al. (2020). ETV2 (ets variant transcription factor 2)-rhoj cascade regulates endothelial progenitor cell migration during embryogenesis. Arterioscler. Thromb. Vasc. Biol. 40, 2875–2890. doi:10.1161/ATVBAHA.120.314488
Singh, B. N., Tahara, N., Kawakami, Y., Das, S., Koyano-Nakagawa, N., Gong, W., et al. (2017). Etv2-miR-130a-Jarid2 cascade regulates vascular patterning during embryogenesis. PLoS One 12, e0189010. doi:10.1371/journal.pone.0189010
Stafford, N. (2019). Leonard L Bailey: In 1984 he transplanted a baboon heart into a human infant known as “Baby Fae”. BMJ 366, l4669. doi:10.1136/bmj.l4669
Stricker-Krongrad, A., Shoemake, C., Brocksmith, D., Liu, J., Hamlin, R., and Bouchard, G. (2017). Comparative cardiovascular physiology and pathology in selected lineages of minipigs: Relation to drug safety evaluation. Toxicol. Res. Appl. 1, 239784731769636. doi:10.1177/2397847317696367
Stricker-Krongrad, A., Shoemake, C. R., and Bouchard, G. F. (2016). The miniature swine as a model in experimental and translational medicine. Toxicol. Pathol. 44, 612–623. doi:10.1177/0192623316641784
Swindle, M. M., and Smith, A. C. (2016). Swine in the laboratory: surgery, anesthesia, imaging, and experimental techniques. 3rd Edn, Editor D. D. M. Jr, J. A. Diaz, M. L. Conte, and M. M. Swindle (CRC Press).
Sykes, M., and Sachs, D. H. (2019). Transplanting organs from pigs to humans. Sci. Immunol. 4, eaau6298. doi:10.1126/sciimmunol.aau6298
Tajbakhsh, S., Rocancourt, D., Cossu, G., and Buckingham, M. (1997). Redefining the genetic hierarchies controlling skeletal myogenesis: Pax-3 and myf-5 act upstream of MyoD. Cell 89, 127–138. doi:10.1016/s0092-8674(00)80189-0
Takahashi, K., Tanabe, K., Ohnuki, M., Narita, M., Ichisaka, T., Tomoda, K., et al. (2007). Induction of pluripotent stem cells from adult human fibroblasts by defined factors. Cell 131, 861–872. doi:10.1016/j.cell.2007.11.019
Takahashi, K., and Yamanaka, S. (2006). Induction of pluripotent stem cells from mouse embryonic and adult fibroblast cultures by defined factors. Cell 126, 663–676. doi:10.1016/j.cell.2006.07.024
Theunissen, T. W., and Jaenisch, R. (2017). Mechanisms of gene regulation in human embryos and pluripotent stem cells. Development 144, 4496–4509. doi:10.1242/dev.157404
Tian, X. C., Kubota, C., Enright, B., and Yang, X. (2003). Cloning animals by somatic cell nuclear transfer-biological factors. Reprod. Biol. Endocrinol. 1, 98. doi:10.1186/1477-7827-1-98
Valdez, M. R., Richardson, J. A., Klein, W. H., and Olson, E. N. (2000). Failure of Myf5 to support myogenic differentiation without myogenin, MyoD, and MRF4. Dev. Biol. 219, 287–298. doi:10.1006/dbio.2000.9621
Virani, S. S., Alonso, A., Aparicio, H. J., Benjamin, E. J., Bittencourt, M. S., Callaway, C. W., et al. (2021). Heart disease and stroke statistics-2021 update: A report from the American heart association. Circulation 143, e254–e743. doi:10.1161/CIR.0000000000000950
Wang, X., Li, T., Cui, T., Yu, D., Liu, C., Jiang, L., et al. (2018). Human embryonic stem cells contribute to embryonic and extraembryonic lineages in mouse embryos upon inhibition of apoptosis. Cell Res. 28, 126–129. doi:10.1038/cr.2017.138
Watanabe, H., Ariyoshi, Y., Pomposelli, T., Takeuchi, K., Ekanayake-Alper, D. K., Boyd, L. K., et al. (2020). Intra-bone bone marrow transplantation from hCD47 transgenic pigs to baboons prolongs chimerism to >60 days and promotes increased porcine lung transplant survival. Xenotransplantation 27, e12552. doi:10.1111/xen.12552
Waterworth, P. D., Dunning, J., Tolan, M., Cozzi, E., Langford, G., Chavez, G., et al. (1998). Life-supporting pig-to-baboon heart xenotransplantation. J. Heart Lung Transpl. 17, 1201–1207.
Wei, K., Zhang, T., and Ma, L. (2018). Divergent and convergent evolution of housekeeping genes in human-pig lineage. PeerJ 6, e4840. doi:10.7717/peerj.4840
Wilmut, I., Schnieke, A. E., Mcwhir, J., Kind, A. J., and Campbell, K. H. (1997). Viable offspring derived from fetal and adult mammalian cells. Nature 385, 810–813. doi:10.1038/385810a0
Wilmut, I., Schnieke, A. E., Mcwhir, J., Kind, A. J., and Campbell, K. H. (2007). Viable offspring derived from fetal and adult mammalian cells. Cloning Stem Cells 9, 3–7. doi:10.1089/clo.2006.0002
Wu, J., Platero Luengo, A., Gil, M. A., Suzuki, K., Cuello, C., Morales Valencia, M., et al. (2016). Generation of human organs in pigs via interspecies blastocyst complementation. Reprod. Domest. Anim. 51, 18–24. doi:10.1111/rda.12796
Wu, J., Platero-Luengo, A., Sakurai, M., Sugawara, A., Gil, M. A., Yamauchi, T., et al. (2017). Interspecies chimerism with mammalian pluripotent stem cells. Cell 168, 473–486. e15. doi:10.1016/j.cell.2016.12.036
Yamada, K., Yazawa, K., Shimizu, A., Iwanaga, T., Hisashi, Y., Nuhn, M., et al. (2005). Marked prolongation of porcine renal xenograft survival in baboons through the use of alpha1, 3-galactosyltransferase gene-knockout donors and the cotransplantation of vascularized thymic tissue. Nat. Med. 11, 32–34. doi:10.1038/nm1172
Yamaguchi, T., Sato, H., Kato-Itoh, M., Goto, T., Hara, H., Sanbo, M., et al. (2017). Interspecies organogenesis generates autologous functional islets. Nature 542, 191–196. doi:10.1038/nature21070
Zheng, C., Hu, Y., Sakurai, M., Pinzon-Arteaga, C. A., Li, J., Wei, Y., et al. (2021). Cell competition constitutes a barrier for interspecies chimerism. Nature 592, 272–276. doi:10.1038/s41586-021-03273-0
Keywords: blastocyst complementation, somatic cell nuclear transfer, pigs, ETV2, MyoD, Myf5, MYF6
Citation: Choe Y-h, Sorensen J, Garry DJ and Garry MG (2022) Blastocyst complementation and interspecies chimeras in gene edited pigs. Front. Cell Dev. Biol. 10:1065536. doi: 10.3389/fcell.2022.1065536
Received: 09 October 2022; Accepted: 17 November 2022;
Published: 08 December 2022.
Edited by:
Jun Wu, University of Texas Southwestern Medical Center, United StatesReviewed by:
Canbin Zheng, The First Affiliated Hospital of Sun Yat-Sen University, ChinaHiromitsu Nakauchi, Stanford University, United States
Copyright © 2022 Choe, Sorensen, Garry and Garry. This is an open-access article distributed under the terms of the Creative Commons Attribution License (CC BY). The use, distribution or reproduction in other forums is permitted, provided the original author(s) and the copyright owner(s) are credited and that the original publication in this journal is cited, in accordance with accepted academic practice. No use, distribution or reproduction is permitted which does not comply with these terms.
*Correspondence: Daniel J. Garry, garry@umn.edu; Mary G. Garry, garry002@umn.edu