Hypoxia in Bone and Oxygen Releasing Biomaterials in Fracture Treatments Using Mesenchymal Stem Cell Therapy: A Review
- 1Department of Medical Microbiology, Faculty of Medicine and Health Sciences, Universiti Putra Malaysia, Serdang, Malaysia
- 2Department of Biomedical Sciences, Faculty of Medicine and Health Sciences, Universiti Putra Malaysia, Serdang, Malaysia
- 3Department of Technology, Research Center for Hua-Da Precision Medicine of Inner Mongolia Autonomous Region, Hohhot, China
- 4School of Bioscience, Faculty of Medicine, Bioscience and Nursing, MAHSA University, Jenjarom, Malaysia
- 5Department of Orthopedics, Faculty of Medicine and Health Sciences, Universiti Putra Malaysia, Serdang, Malaysia
- 6Center for Materials Engineering and Regenerative Medicine, Bharath Institute of Higher Education and Research, Bharath University, Chennai, India
Bone fractures have a high degree of severity. This is usually a result of the physical trauma of diseases that affect bone tissues, such as osteoporosis. Due to its highly vascular nature, the bone is in a constant state of remodeling. Although those of younger ages possess bones with high regenerative potential, the impact of a disrupted vasculature can severely affect the recovery process and cause osteonecrosis. This is commonly seen in the neck of femur, scaphoid, and talus bone. In recent years, mesenchymal stem cell (MSC) therapy has been used to aid in the regeneration of afflicted bone. However, the cut-off in blood supply due to bone fractures can lead to hypoxia-induced changes in engrafted MSCs. Researchers have designed several oxygen-generating biomaterials and yielded varying degrees of success in enhancing tissue salvage and preserving cellular metabolism under ischemia. These can be utilized to further improve stem cell therapy for bone repair. In this review, we touch on the pathophysiology of these bone fractures and review the application of oxygen-generating biomaterials to further enhance MSC-mediated repair of fractures in the three aforementioned parts of the bone.
Bone Ischemia
This review is mainly focused on the use of oxygen releasing biomaterials with mesenchymal stem cell therapy to address hypoxia in the treatment of bone fractures (Figure 1). Bone fracture cases are commonly presented in varying degrees of severity and are usually a manifestation of direct physical injury or bone-related diseases such as osteoporosis (Burge et al., 2007). With gradual improvements in healthcare and living, an increase in these cases is predictable because of the longer life expectancies (Rommens, 2019). Although the bone is seen as a rigid structure, it is classified as a connective tissue that constantly remodels itself throughout a human’s lifespan in a highly vascularized environment (Boskey and Coleman, 2010; Cowan and Kahai, 2018). Despite having modest regenerative potential, especially in children, a loss of blood supply in the bone can impede cellular repair and potentially lead to osteonecrosis (Shah et al., 2015). This cut-off in blood circulation can be due to fractures or dislocations that physically cause an interruption. This pathophysiology is commonly observed in the neck of femur (Shah et al., 2015), scaphoid (Hayat and Varacallo, 2018), and talus bone (Matthews and Stitson, 2018).
The femur is a piece of bone in the upper leg that articulates with the acetabulum of the pelvic bone and the tibia of the lower leg. A fracture of the femur neck, which connects the femur head to the shaft, has a high risk of cutting off the blood supply by damaging the ascending arteries and causing necrosis (Gumustas et al., 2018). This avascularity in the femur head was found to arise in about 72% of patients who suffered from femur neck fractures, and the incidence increases with severity (Han et al., 2019). One example is a displaced femoral neck fracture (Figure 2A). Fractures of the scaphoid (Figure 2B) are common in the carpals, and are often observed in young, working individuals (Hayat and Varacallo, 2018). Similar to the femur neck, blood vessel interruption in this wrist bone can lead to avascularity and even arthritis if treatment is inadequate (Dias et al., 2016).
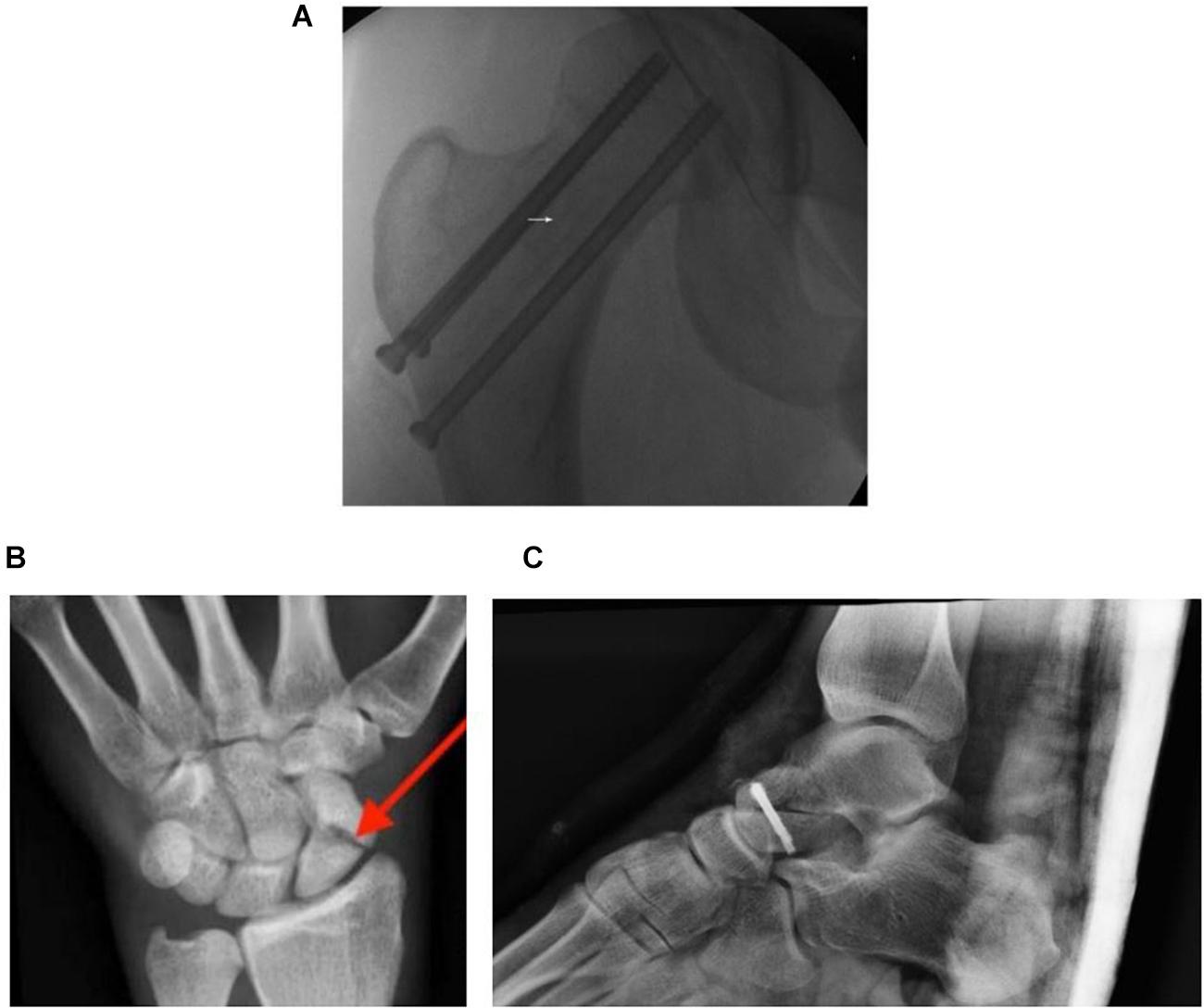
Figure 2. (A) An anteroposterior fluorograph of a displaced femoral neck fracture fixed with cannulated screws. The femoral neck (region around white arrow) connects the femoral head to the rest of the femur. (B) Plain radiograph of the left anteroposterior carpals of a young adult presented with a full thickness scaphoid fracture (red arrow). (C) Post-operative plain radiograph of a talus presented with a fracture. Adapted from Patel (2012), Li et al. (2013), and Kim and Yanuck (2018).
Much like how the carpal bones are important for wrist movement, the talus (Figure 2C) is a bone in the lower foot that is crucial for movement. Due to its unique retrograde blood supply, as well as having no direct attachments to any other bones, physical trauma such as from a heavy fall can displace the talus and disrupt vascular supply (Clare and Maloney, 2019). This was found to have a high association with the occurrence of avascular necrosis (Lindvall et al., 2004).
Hypoxia Limits Orthopedic Treatment Efficiency
Clinical treatment in these cases depends on the severity of the fracture and typically involves an immediate fixation or application of a cast to promote bone union (Matthews and Stitson, 2018). However, the risk of osteonecrosis remains. A more severe injury in the talus, for example, gives rise to more complications regardless of the timing of treatment (Vallier et al., 2004). Even with surgical or non-surgical treatments, bone union and cessation of osteonecrosis are not guaranteed, including the aforementioned bones (Sanders et al., 2004; Dias and Singh, 2011; Gumustas et al., 2018). In recent years, mesenchymal stem cell (MSC) therapy has been used to aid in the regeneration of bone afflicted with osteonecrosis, such as in the femur head (Wang et al., 2019). Since MSCs are multipotent, these stem cells are able to differentiate into osteocytes as the cells are derived from the mesoderm (Fafián-Labora et al., 2019). However, the cut-off in blood supply due to bone fractures can lead to hypoxia-induced changes in engrafted MSCs. Hypoxia is known to affect the proliferation, differentiation, metabolism, and viability of MSCs in vitro (Ejtehadifar et al., 2015). Exposure to hypoxia leads to increased cell death, but over time there is an upregulation of stemness genes such as Oct-4, Sox2, and Nanog, as the cells persist in an undifferentiated state (Samal et al., 2021). Interestingly, high oxygen tensions also affect MSCs by reducing proliferative capacity (Krinner et al., 2009).
Besides lowering cell viability, hypoxia has been shown to inhibit metabolic switch and osteogenesis of MSCs (Hsu et al., 2013). The inhibitory effects were demonstrated by suppressed osteogenic markers expression and mineralization of MSCs during osteogenic differentiation (He et al., 2010). Kim et al. (2016) has reported downregulation of calcification, and osteonectin and osteopontin gene expression, implying suppression of osteogenesis in adipose-derived stem cells (ADSCs). It was described that the suppression of osteogenic differentiation involves the generation of reactive oxygen species under oxygen deficiency, which activates Mitogen-Activated Protein Kinase (MAPK) and Phosphatidylinositol 3-Kinase/Akt (PI3K/Akt) signaling pathways and upregulates intracellular Insulin-like Growth Factor-Binding Protein 3 (IGFBP3) level. Osteogenic differentiation in bone marrow-derived mesenchymal stem cells (BMSCs) was also suppressed under low oxygen condition. Hypoxia of 2% oxygen decreased mineralization and alkaline phosphatase (ALP) activity during osteogenesis (Huang et al., 2012). As such, hypoxia results in a reduction of therapeutic efficacy for bone regeneration due to reduced osteogenesis. Oxygen supplementation can therefore reverse this, and promote bone regeneration by introducing normoxic conditions for osteogenic MSCs. However, this requires careful optimization as too much oxygen supply can lead to hyperoxia and subsequently cell death due to the presence of reactive oxygen species, e.g., hydroxyl radicals (OH-) (Northup and Cassidy, 2008).
Ischemia represents a critical issue during engraftment procedure, especially in the regions without intact vasculature or vascular supply, such as femur, talus, and scaphoid. Persistent ischemia can cause cell death and tissue necrosis (Blaisdell, 2002). Supplementation of oxygen to hypoxic tissue lack of vascularization can ameliorate hypoxia-induced cell death by improving cell rescue and viability. The provision of oxygen is beneficial for tissue regeneration. Supplemental oxygenation of the engineered tissue scaffold can eliminate the hindrance of insufficient oxygen in hypoxic tissues, thus reducing dying or dysfunctional cells in the tissue-engineered graft. Continuous and localized oxygen supply can also delay the onset of necrosis in tissue experiencing hypoxia, resulting in extended tissue viability and improved wound repair. However, the supply of oxygen to hypoxic tissue during engineered tissue engraftment remains the main medical challenge. In the effort to avert ischemia-related cells death, numerous approaches have been studied for the delivery of oxygen to various tissues under hypoxia or ischemia. Creating arteriovenous loops (AV) is one method. In a rat AV loop model, an increase in vascularization was correlated with the increase in Hypoxia Inducible Factor-1 alpha (HIF-1α) rate (Yuan et al., 2018). Another method is to pre-vascularized grafts before transplantation. In a rabbit model, pre-vascularized synthetic bone grafts lead to neovascularization and enhanced bone regeneration (Vidal et al., 2020). Apart from these, the use of scaffolds with oxygen-releasing biomaterials is particularly promising. These can be produced by incorporating the compounds on a 2D scaffold surface or as a 3D capsule. Examples include solid peroxides, liquid peroxides, and fluorinated compounds that function to provide oxygen to cells (Suvarnapathaki et al., 2019).
Oxygen Releasing Biomaterials
Researchers have designed several oxygen-generating biomaterials and yielded varying degrees of success in enhancing tissue salvage and preserving cellular metabolism under ischemia. Delivering a sustained source of oxygen would represent a major therapeutic advancement in tissue restoration and regeneration following acute trauma and bone defect. Harrison et al. (2007) have reported sustained release of oxygen by Poly(D,L-lactide-co-glycolic acid) (PLGA) films integrated with sodium percarbonate (SPO). The implantable oxygen-rich compound SPO can delay tissue death in the hypoxic milieu. The SPO contains sodium bicarbonate and hydrogen peroxide, which readily convert to oxygen upon contact with water as shown in the following chemical equation.
The films were also shown to prolong skin cells survival demonstrated by delayed cells degradation, including apoptosis, lactate accumulation, and skin discoloration. The in situ production of oxygen has shown to reduce cellular apoptosis and tissue necrosis in the ischemic tissue of mouse model. The effect of cell ischemia continues once the oxygen production is exhausted. This technology employed as a skin wound healing agent was able to delay the onset of necrosis up to 3 days (Harrison et al., 2007). The use of oxygen-releasing peroxide on PLGA films for enhanced cell viability was also validated on a hypoxic fibroblast proliferation study in a 3D tissue-engineered constructs (Oh et al., 2009). Researchers also developed an injectable composite system of PLGA encapsulated in calcium peroxide (CaO2)/manganese dioxide (MnO2) microparticles (Hsieh et al., 2020). Under low oxygen tension-induced cultures, they were able to promote the differentiation of pre-osteoblast cells. The enhanced local oxygenation by this composite system was found to possess improved bone regeneration potential.
Hypoxic conditions can cause interruption to skeletal muscle metabolism and function. Biomaterials supplementation of oxygen by active element SPO has been demonstrated to support resting skeletal muscle homeostasis under ischemia (Ward et al., 2013). In the study, oxygen-generating SPO mitigated elevations of muscle cells resting tension following contractile fatigue under normoxic conditions. Under oxygen deficiency, SPO lessens HIF-1α build-up, oxidative stress, and ameliorated intramuscular glycogen depletion. SPO administered into ischemia rat skeletal muscle enhanced in vivo contractility and ameliorated intramuscular glycogen depletion (Ward et al., 2013). The results indicated that SPO can maintain the contractility of skeletal muscle both in vitro and in vivo under ischemia. Hypoxia-induced loss of skeletal muscle viability and metabolic homeostasis can also be prevented in the micro-environment with no functioning vasculature.
Calcium peroxide is one important source of oxygen production via its decomposition by water.
However, the hydrolysis reaction occurs too quickly in the conversion of solid peroxide to oxygen. This causes hyperoxide conditions and leads to the accumulation of reaction intermediate hydrogen peroxide (H2O2). In addition, the reaction increases the possibility of side reactions such as the production of hydroxyl radical (OH-) (Northup and Cassidy, 2008). Previously reported oxygen releasing biomaterials have mutual limitations, which are less controllable reaction kinetics and short-lived due to the PLGA hydrolytic decomposition. Moreover, cytotoxicity caused by H2O2 might occur which can lead to cell death as well as reduced ALP activity and mineralization during the osteogenic differentiation process (Lee et al., 2006). Catalase supplementation is always required to counter H2O2 cytotoxicity by catalyzing its decomposition (Tiedge et al., 1997). Therefore, utilization of such biomaterials can be harmful to transplanted MSCs.
A possible solution for a slower and sustained oxygen release is solid peroxide encapsulation. Calcium peroxide without encapsulation rapidly generates oxygen via hydrolytic conversion, leads to spurts of oxygen that are too transient for cells utilization (Northup and Cassidy, 2008). The bio-stable and hydrophobic (polydimethylsiloxane) PDMS can serve as a diffusional barrier which can decrease the reactivity of the encapsulated calcium peroxide. The capturing of solid peroxide within PDMS were found to regulate oxygen release into the surrounding for over 40 days. Oxygen production by the system is dependent on the water diffusion rate into the PDMS-CaO2 disk and the amount of solid peroxide in the disks (Watson and Baron, 1996). PDMS is highly permeable for the oxygen generated to be diffused efficiently out of the system for cellular usage (Robb, 1968). With the controllable features, the concentration of solid peroxide in the disks, and the disks geometry and dimensions can be optimized to design an oxygen generating system with ideal kinetics of oxygen release. The modulation of reactivity of solid peroxide hydrolysis and rapid clearance of end products ensure the dynamic of the forward reaction, thus eliminating the accumulation of hydrogen peroxide intermediate and side reactions such as hydroxyl radicals. As such, PDMS serves as a suitable biomaterial for maintaining viability and function of transplanted cells under ischemic conditions.
Pedraza et al. (2012) have reported the fabrication of a hydrolytically activated oxygen-producing biomaterial. The designed disk of solid calcium peroxide (CaO2) encapsulated in hydrophobic polydimethylsiloxane (PDMS), PDMS-CaO2 can generate continuous oxygen release for as long as 6 weeks. A single PDMS-CaO2 disk was sufficient to regulate cells function of both β cell line and pancreatic rat islets to their normoxic controls. Hypoxia-induced cell dysfunction and death were ameliorated, where the cellular metabolic function and the production of glucose-dependent insulin were regulated as to that under normoxic conditions. Under experimental ischemia, the viability of the β cell line was improved for almost a month with the sustained supply of oxygen from the PDMS-CaO2 disk (Pedraza et al., 2012). Ischemia-induced cells apoptosis and dysfunction were prevented by suppression of cell stress pathways activation and shifting to anaerobic metabolism (Lee et al., 2006). Additionally, scaffolds have been used with CaO2 to better modulate oxygen release (Touri et al., 2020). These CaO2-coated scaffolds were found to augment bone formation at the regions between the scaffolds and the host’s bone. Osteogenic markers such as osteonectin and osteocalcin were upregulated compared to the bone treated with uncoated scaffolds. Other researchers have also developed methods to directly release molecular oxygen instead of hydrogen peroxide-based methods that rely on decomposition to generate oxygen. One such team was able to create hypoxia-sensitive, oxygen molecule-releasing, microspheres and co-injected them with MSCs into mouse ischemic limbs (Guan et al., 2021). They found significant MSCs survival, proliferation, and angiogenesis without the induction of inflammation.
Another approach was conducted to encapsulate pure H2O2 into a dual-layer matrix to produce clean oxygen for tissue salvage applications (Abdi et al., 2013). The direct usage of encapsulated H2O2 can prevent the generation of by-products including metal cations, and only yield oxygen and water which are non-toxic (Fatokun et al., 2006). Encapsulated raw H2O2 normally diffuses out of the biological matrix at a slow pace, increases its direct contact with the cells and the possibility of exerting cytotoxic effect (Abdi et al., 2011). Therefore, the PLGA matrix was coated with catalase-grafted alginate in the study to serve as a protective layer and promote H2O2 decomposition. In this method, oxygen release can be controlled by manipulating alginate concentration in the microspheres encapsulating H2O2. Sustained oxygen supply from decomposition of encapsulated H2O2 in the fabricated dual layered system has shown to enhance cell survival under ischemia (Abdi et al., 2011). The study also revealed optimum H2O2 concentration to generate efficient amount of oxygen to maintain muscle cells viability under controlled release manner (Abdi et al., 2013). Some researchers have produced a bile acid-based dual-functional prodrug nanoparticle that can scavenge H2O2, promote osteogenesis, and inhibit adipogenesis of MSCs in a bone defect rat model (Arai et al., 2020). They found a significant improvement in bone regeneration as well as potent anti-inflammatory activities in the MSCs. Other than that, catalase enzymes could be grafted onto microspheres such as poly (L-lactic acid) (PLLA) to speed up the conversion of H2O2 (Mohseni-Vadeghani et al., 2021). Additionally, by using PLLA to load CaO2 and allowing mesenchymal stem cells to adhere to the surface, the oxygen release profile of this system was found to be further sustained. This led to the thought of a potential microcarrier with an injectable cell system for improved bone regeneration.
Conclusion
Engineered graft implants face major challenges of tissue necrosis and cellular apoptosis upon contact with the hypoxic micro-environment. Provision of sufficient oxygen to the surgery site is of utmost importance for maximum survival and integration of transplanted cells to the wound. Technology to generate oxygen releasing biomaterials as transplantable graft can provide a sustained infusion of oxygen to the local tissue to accelerate tissue regeneration, thereby represents a viable solution for rescuing hypoxic cells. There are, however, potential limitations to this method. A compromised vasculature in the bone defect that persists over the long term could limit the effectiveness of the oxygen-releasing biomaterial since it is transient. Revascularization as a more general treatment against osteonecrosis may yield better long-term recovery, but this requires in-depth studies. Not only that, the failure of mesenchymal stem cell survival after implantation may be due to glucose shortage rather than hypoxia (Moya et al., 2018). As such, exploring glucose-releasing biomaterials could yield surprising results. Still, the importance of vascularization in transplantation and bone repair should still be investigated.
The concept of supplemental oxygen by biomaterials is particularly attractive in orthopedics tissue salvage application. The early bone graft transplantation stages in femur, talus, and scaphoid are susceptible to failure due to the absence of sufficient oxygenation and vascular infiltration. The introduction of engineered tissue graft further aggravates ischemia due to higher metabolic requirements. Sustained in situ production of supplemental oxygen appears to be promising for tissue repair applications as tissue graft can remain viable during surgery and proliferate as normal in the hypoxic milieu. The biomaterials help to prevent the development of harmful oxygen gradients that might occur in tissue-engineered implants. Furthermore, oxygenation by biomaterials omits the need for multiple operations to refill engineered tissue grafts due to hypoxic-induced cell loss. Therefore, the provision of supplementary oxygen would serve to enhance cellular viability and benefit the wound healing process. Thus, oxygen-producing biomaterials represent an ideal tool for mitigating oxygenation of orthopedics engineered tissue transplant, specifically for femur, talus, and scaphoid.
Author Contributions
SWT and AE-HK composed the manuscript and prepared the figures. JBT, XW, AVS, SR, PLM, and SKS commented on the manuscript. SKS and PLM approved the manuscript. All authors reviewed the manuscript.
Funding
This work was funded by the Ministry of Higher Education under Fundamental Research Grant Scheme (FRGS/2/2013/SKK01/UPM/02/11).
Conflict of Interest
The authors declare that the research was conducted in the absence of any commercial or financial relationships that could be construed as a potential conflict of interest.
Publisher’s Note
All claims expressed in this article are solely those of the authors and do not necessarily represent those of their affiliated organizations, or those of the publisher, the editors and the reviewers. Any product that may be evaluated in this article, or claim that may be made by its manufacturer, is not guaranteed or endorsed by the publisher.
References
Abdi, S. I., Ng, S. M., and Lim, J. O. (2011). An enzyme-modulated oxygen-producing micro-system for regenerative therapeutics. Int. J. Pharm. 409, 203–205. doi: 10.1016/j.ijpharm.2011.02.041
Abdi, S. I. H., Choi, J. Y., Lau, H. C., and Lim, J. O. (2013). Controlled release of oxygen from PLGA-alginate layered matrix and its in vitro characterization on the viability of muscle cells under hypoxic environment. Tissue Eng. Regen. Med. 10, 131–138. doi: 10.1007/s13770-013-0391-7
Arai, Y., Park, H., Park, S., Kim, D., Baek, I., Jeong, L., et al. (2020). Bile acid-based dual-functional prodrug nanoparticles for bone regeneration through hydrogen peroxide scavenging and osteogenic differentiation of mesenchymal stem cells. J. Control Release 328, 596–607. doi: 10.1016/j.jconrel.2020.09.023
Blaisdell, F. W. (2002). The pathophysiology of skeletal muscle ischemia and the reperfusion syndrome: a review. Cardiovasc. Surg. 10, 620–630. doi: 10.1016/s0967-2109(02)00070-4
Burge, R., Dawson-Hughes, B., Solomon, D. H., Wong, J. B., King, A., and Tosteson, A. (2007). Incidence and economic burden of osteoporosis-related fractures in the United States, 2005-2025. J. Bone. Miner. Res. 22, 465–475. doi: 10.1359/jbmr.061113
Clare, M. P., and Maloney, P. J. (2019). Prevention of avascular necrosis with fractures of the talar neck. Foot Ankle Clin. 24, 47–56. doi: 10.1016/j.fcl.2018.09.003
Dias, J., Brealey, S., Choudhary, S., Cook, L., Costa, M., Fairhurst, C., et al. (2016). Scaphoid waist internal fixation for fractures trial (SWIFFT) protocol: a pragmatic multi-centre randomised controlled trial of cast treatment versus surgical fixation for the treatment of bi-cortical, minimally displaced fractures of the scaphoid waist in adults. BMC Musculoskelet. Disord. 17:248. doi: 10.1186/s12891-016-1107-7
Dias, J. J., and Singh, H. P. (2011). Displaced fracture of the waist of the scaphoid. J. Bone Joint Surg. Br. 93-B, 1433–1439.
Ejtehadifar, M., Shamsasenjan, K., Movassaghpour, A., Akbarzadehlaleh, P., Dehdilani, N., Abbasi, P., et al. (2015). The effect of hypoxia on mesenchymal stem cell biology. Adv. Pharm. Bull. 5, 141–149. doi: 10.15171/apb.2015.021
Fafián-Labora, J. A., Morente-López, M., and Arufe, M. C. (2019). Effect of aging on behaviour of mesenchymal stem cells. World J. Stem Cells 11, 337–346. doi: 10.4252/wjsc.v11.i6.337
Fatokun, A., Stone, T., and Ra Smith, R. A. (2006). Hydrogen peroxide-induced oxidative stress in MC3T3-E1 cells: the effects of glutamate and protection by purines. Bone 39, 542–551. doi: 10.1016/j.bone.2006.02.062
Guan, Y., Gao, N., Niu, H., Dang, Y., and Guan, J. (2021). Oxygen-release microspheres capable of releasing oxygen in response to environmental oxygen level to improve stem cell survival and tissue regeneration in ischemic hindlimbs. J. Control Release 331:376. doi: 10.1016/j.jconrel.2021.01.034
Gumustas, S., Tosun, H. B., Isyar, M., Serbest, S., Oznam, K., and Bulut, G. (2018). Femur neck fracture in young adults, is it really an urgent surgery indication: retrospective clinical study. Pan. Afr. Med. J. 30:112.
Han, Y.-H., Jeong, H.-J., Sohn, M.-H., Yoon, S.-J., and Lim, S. T. (2019). Incidence and severity of femoral head avascularity after femoral neck or intertrochanteric fractures on preoperative bone single photon emission computed tomography/computed tomography. Nucl. Med. Commun. 40, 199–205. doi: 10.1097/mnm.0000000000000963
Harrison, B., Eberli, D., Lee, S., Atala, A., and Yoo, J. J. (2007). Oxygen producing biomaterials for tissue regeneration. Biomaterials 28, 4628–4634. doi: 10.1016/j.biomaterials.2007.07.003
He, J., Genetos, D. C., Yellowley, C. E., and Leach, J. K. (2010). Oxygen tension differentially influences osteogenic differentiation of human adipose stem cells in 2D and 3D cultures. J. Cell. Biochem. 110, 87–96.
Hsieh, T. E., Lin, S. J., Chen, L. C., Chen, C. C., Lai, P. L., and Huang, C. C. (2020). Optimizing an injectable composite oxygen-generating system for relieving tissue hypoxia. Front. Bioeng. Biotechnol. 8:511. doi: 10.3389/fbioe.2020.00511
Hsu, S.-H., Chen, C.-T., and Wei, Y.-H. (2013). Inhibitory effects of hypoxia on metabolic switch and osteogenic differentiation of human mesenchymal stem cells. Stem Cells 31, 2779–2788. doi: 10.1002/stem.1441
Huang, Y.-C., Zhu, H.-M., Cai, J.-Q., Huang, Y.-Z., Xu, J., Zhou, Y., et al. (2012). Hypoxia inhibits the spontaneous calcification of bone marrow-derived mesenchymal stem cells. J. Cell Biochem. 113, 1407–1415. doi: 10.1002/jcb.24014
Kim, E., and Yanuck, J. (2018). Scaphoid fracture. J. Educ. Teach. Emerg. Med. 3:2. doi: 10.21980/J80344
Kim, J., Yoon, S., Song, S., Park, S., Kim, W., and Park, I. (2016). Hypoxia suppresses spontaneous mineralization and osteogenic differentiation of mesenchymal stem cells via IGFBP3 up-regulation. Int. J. Mol. Sci. 17:1389. doi: 10.3390/ijms17091389
Krinner, A., Zscharnack, M., Bader, A., Drasdo, D., and Galle, J. (2009). Impact of oxygen environment on mesenchymal stem cell expansion and chondrogenic differentiation. Cell. Prolif. 42, 471–484. doi: 10.1111/j.1365-2184.2009.00621.x
Lee, D., Lim, B., Lee, Y., and Yang, H. C. (2006). Effects of hydrogen peroxide (H2O2) on alkaline phosphatase activity and matrix mineralization of odontoblast and osteoblast cell lines. Cell Biol. Toxicol. 22, 39–46. doi: 10.1007/s10565-006-0018-z
Li, Z., Chen, W., Su, Y., Zhang, Q., Hou, Z., Pan, J., et al. (2013). The application of closed reduction internal fixation and iliac bone block grafting in the treatment of acute displaced femoral neck fractures. PLoS One 8:e75479. doi: 10.1371/journal.pone.0075479
Lindvall, E. D. O., Haidukewych, G. M. D., DiPasquale, T. D. O., Herscovici, D. D. O., and Sanders, R. M. D. (2004). Open reduction and stable fixation of isolated, displaced talar neck and body fractures. J. Bone Joint Surg. 86, 2229–2234. doi: 10.2106/00004623-200410000-00014
Mohseni-Vadeghani, E., Karimi-Soflou, R., Khorshidi, S., and Karkhaneh, A. (2021). Fabrication of oxygen and calcium releasing microcarriers with different internal structures for bone tissue engineering: solid filled versus hollow microparticles. Colloids Surf. B Biointerf. 197:11376.
Moya, A., Paquet, J., Deschepper, M., Larochette, N., Oudina, K., Denoeud, C., et al. (2018). Human mesenchymal stem cell failure to adapt to glucose shortage and rapidly use intracellular energy reserves through glycolysis explains poor cell survival after implantation. Stem Cells 36, 363–376. doi: 10.1002/stem.2763
Northup, A., and Cassidy, D. (2008). Calcium peroxide (CaO2) for use in modified Fenton chemistry. J. Hazard. Mater. 152, 1164–1170. doi: 10.1016/j.jhazmat.2007.07.096
Oh, S., Ward, C., Atala, A., Yoo, J., and Harrison, B. S. (2009). Oxygen generating scaffolds for enhancing engineered tissue survival. Biomaterials 30, 757–762. doi: 10.1016/j.biomaterials.2008.09.065
Patel, M. (2012). Fracture of Talus. Available online at: Radiopaedia.org (accessed October 3, 2020).
Pedraza, E., Coronel, M., and Fraker, C. A. (2012). Preventing hypoxia-induced cell death in beta cells and islets via hydrolytically activated, oxygen-generating biomaterials. Proc. Natl. Acad. Sci. U.S.A. 109, 4245–4250. doi: 10.1073/pnas.1113560109
Robb, W. L. (1968). Thin silicone membranes-their permeation properties and some applications. Ann. N. Y. Acad. Sci. 146, 119–137. doi: 10.1111/j.1749-6632.1968.tb20277.x
Rommens, P. M. (2019). Paradigm shift in geriatric fracture treatment. Eur. J. Trauma Emerg. Surg. 45, 181–189. doi: 10.1007/s00068-019-01080-x
Samal, J. R. K., Rangasami, V. K., Samanta, S., Varghese, O. P., and Oommen, O. P. (2021). Discrepancies on the role of oxygen gradient and culture condition on mesenchymal stem cell fate. Adv. Healthcare Mater. 10:2002058. doi: 10.1002/adhm.202002058
Sanders, D. W., Busam, M., Hattwick, E., Edwards, J. R., McAndrew, M. P., and Johnson, K. D. (2004). Functional outcomes following displaced talar neck fractures. J. Orthop. Trauma 18, 265–270. doi: 10.1097/00005131-200405000-00001
Shah, K. N., Racine, J., Jones, L. C., and Aaron, R. K. (2015). Pathophysiology and risk factors for osteonecrosis. Curr. Rev. Musculoskelet. Med. 8, 201–209. doi: 10.1007/s12178-015-9277-8
Suvarnapathaki, S., Wu, X., Lantigua, D., Nguyen, M. A., and Camci-Unal, G. (2019). Breathing Life into Engineered Tissues Using Oxygen-Releasing Biomaterials. Berlin: Nature Publishing Group, 1–18.
Tiedge, M., Lortz, S., Drinkgern, J., and Lenzen, S. (1997). Relation between antioxidant enzyme gene expression and antioxidative defense status of insulin-producing cells. Diabetes Metab. Res. Rev. 46, 1733–1742. doi: 10.2337/diabetes.46.11.1733
Touri, M., Moztarzadeh, F., Abu Osman, N. A., Dehghan, M. M., Brouki Milan, P., Farzad-Mohajeri, S., et al. (2020). Oxygen-releasing scaffolds for accelerated bone regeneration. ACS Biomater. Sci. Eng. 6, 2985–2994. doi: 10.1021/acsbiomaterials.9b01789
Vallier, H. A., Nork, S. E., Barei, D. P., Benirschke, S. K., and Sangeorzan, B. J. (2004). Talar neck fractures: results and outcomes. J. Bone Joint Surg. Am. 1616–1624.
Vidal, L., Brennan, M., Krissian, S., De Lima, J., Hoornaert, A., Rosset, P., et al. (2020). In situ production of pre-vascularized synthetic bone grafts for regenerating critical-sized defects in rabbits. Acta Biomater. 114, 384–394. doi: 10.1016/j.actbio.2020.07.030
Wang, Y., Ma, X., Chai, W., and Tian, J. (2019). Multiscale stem cell technologies for osteonecrosis of the femoral head. Stem Cells Int. 2019, 1–13. doi: 10.1155/2019/8914569
Ward, C. L., Corona, B. T., Yoo, J. J., Harrison, B. S., and Christ, G. J. (2013). Oxygen generating biomaterials preserve skeletal muscle homeostasis under hypoxic and ischemic conditions. PLoS One 8:e72485. doi: 10.1371/journal.pone.0072485
Watson, J., and Baron, M. G. (1996). The behaviour of water in poly (dimethylsiloxane). J. Memb. Sci. 110, 47–57. doi: 10.1016/0376-7388(95)00229-4
Keywords: bone ischemia, bone fracture, hypoxia, stem cells, oxygen-releasing biomaterials
Citation: Teh SW, Koh AE-H, Tong JB, Wu X, Samrot AV, Rampal S, Mok PL and Subbiah SK (2021) Hypoxia in Bone and Oxygen Releasing Biomaterials in Fracture Treatments Using Mesenchymal Stem Cell Therapy: A Review. Front. Cell Dev. Biol. 9:634131. doi: 10.3389/fcell.2021.634131
Received: 27 November 2020; Accepted: 22 July 2021;
Published: 20 August 2021.
Edited by:
Stevo J. Najman, University of Niš, SerbiaReviewed by:
Weihua Huang, New York Medical College, United StatesArnaud Scherberich, University Hospital of Basel, Switzerland
Xinchen Wu, University of Massachusetts Lowell, United States
Copyright © 2021 Teh, Koh, Tong, Wu, Samrot, Rampal, Mok and Subbiah. This is an open-access article distributed under the terms of the Creative Commons Attribution License (CC BY). The use, distribution or reproduction in other forums is permitted, provided the original author(s) and the copyright owner(s) are credited and that the original publication in this journal is cited, in accordance with accepted academic practice. No use, distribution or reproduction is permitted which does not comply with these terms.
*Correspondence: Pooi Ling Mok, rachelmok2005@gmail.com; Suresh Kumar Subbiah, sureshkudsc@gmail.com
†These authors have contributed equally to this work