Hox-Positive Adult Mesenchymal Stromal Cells: Beyond Positional Identity
- 1Department of Biochemistry and Molecular Medicine, Faculty of Medicine, Lomonosov Moscow State University, Moscow, Russia
- 2Laboratory of Gene and Cell Therapy, Institute for Regenerative Medicine, Lomonosov Moscow State University, Moscow, Russia
Homeotic genes (Hox) are universal regulators of the body patterning process in embryogenesis of metazoans. The Hox gene expression pattern (Hox code) retains in adult tissues and serves as a cellular positional identity marker. Despite previously existing notions that the Hox code is inherent in all stroma mesenchymal cells as a whole, recent studies have shown that the Hox code may be an attribute of a distinct subpopulation of adult resident mesenchymal stromal cells (MSC). Recent evidence allows suggesting a “non-canonical” role for Hox gene expression which is associated with renewal and regeneration in postnatal organs after damage. In tissues with high regenerative capacity, it has been shown that a special cell population is critical for these processes, a distinctive feature of which is the persistent expression of tissue-specific Hox genes. We believe that in the postnatal period Hox-positive subpopulation of resident MSC may serve as a unique regenerative reserve. These cells coordinate creation and maintenance of the correct structure of the stroma through a tissue-specific combination of mechanisms. In this article, we summarize data on the role of resident MSC with a tissue-specific pattern of Hox gene expression as regulators of correct tissue reconstruction after injury.
Introduction
Homeotic genes (designated as Hox in mice and HOX in humans) is a family of homeotic genes encoding transcription factors known to function as master regulators of cell identity and fate during embryonic development of vertebrates. In mammals, Hox genes regulate patterning of the embryo along the body axis ranging from the anterior boundary of hindbrain till the tail end of the body. Hox genes are highly conserved among species and encode a set of proteins that share a high degree of structural similarity. In mammals, 39 Hox gene family members are organized into four clusters labeled A, B, C, and D – each cluster located on a separate chromosome – namely 7, 17, 12, and 2, respectively.
Each complex occupies a similar position in the harboring chromosome and its genes are numbered ranging from 1 to 13 according to their location from 3′ to 5′ terminus. However, not each group has all 13 genes, – some numbers may be missing (e.g., Hoxd subgroup has nine genes). Genes of the same number (e.g., Hoxa9, Hoxb9,Hoxc9, and Hoxd9) represent paralogues and demonstrate two unique features of Hox genes:
• Spatial collinearity which means that in the embryo their expression is activated in anteroposterior direction in the same order they are located on the chromosome, starting from 3′. For example, genes of paralogous group 3 are expressed posteriorly to genes of group 2 and all the way further to group 13.
• Temporal collinearity which means that during development 3′ genes are expressed earlier than 5′ genes. In vertebrates limb development is determined by posterior Hox genes (Hox9-13) reflecting the principle of collinearity – indeed, the development of distal body parts correlates with expression of “late-numbered” Hox genes. E.g., within a developing limb Hox9 genes are expressed in the most proximal portion while Hox13 genes – in the most distal.
In adult organism cells can retain expression of Hox genes to form the distinct Hox expression pattern known as “Hox code” that strictly matches the Hox spectrum this part of the body demonstrated during embryonic development (Kmita and Duboule, 2003; Lappin et al., 2006; Svingen and Tonissen, 2006). Hox expression patterns are formed at the stage of mesoderm segmentation and subsequently remain unchanged reflecting positional affiliation of cells (Chang et al., 2002; Ackema and Charité, 2008; Picchi et al., 2013). Hox code is maintained throughout life in both – stromal and parenchymal cells, but the functional role of postnatal Hox gene expression in stroma remains highly enigmatic.
Further, we shall present an overview that will guide to a putative role of postnatal Hox expression in subpopulations of stromal cells as a feature required for tissue repair after damage in mammals (including human).
Redundancy of Hox Genes and Structural Anomalies Caused by Their Disruption
From the functional point of view, Hox proteins are DNA-binding transcription factors that can act as an activator for certain target genes and a repressor for others. The specificity of Hox transcription factors binding to a DNA sequence is determined by protein cofactors from other families (Moens and Selleri, 2006). The main targets of Hox are proteins involved in chromatin remodeling and transcription factors which in summary suggests a major role of Hox in the regulation of genome expression through epigenetic and transcriptional control.
Thus, it is no surprise that a critical role of Hox-encoded proteins has been established in a great variety of cellular functions: cell growth, differentiation, migration, invasion, adhesion, etc. (Seifert et al., 2015). At the same time known regulators of Hox gene expression are scarce yet recent data suggests that Hox expression during embryogenesis is regulated by BMP, Wnt, and retinoic acid pathways. In the adult organism, several Hox regulators are found including vitamin D and steroid hormones which shall be discussed in detail below (Seifert et al., 2015; Du and Taylor, 2015).
Despite Hox genes’ pivotal role in embryo patterning, loss of function in a single Hox gene does not always lead to body structural malformation. This matches the existence of paralogous groups mentioned above although the molecular functions of genes among paralogous groups do not fully overlap. In other words, Hox genes are characterized by functional redundancy.
To date, several dozen mouse strains have been created carrying knockouts of different Hox genes. Many of these strains demonstrate profound inborn defects of organs and tissues while some (e.g., with mutant Hoxc5 or Hoxa7) do not display any developmental anomalies. A comprehensive analysis of deviations characteristic for these knockout mice strains can be found in the review by Quinonez and Innis (2014).
In humans, a dozen of developmental anomalies caused by mutations in HOX family genes have been described (Quinonez and Innis, 2014). For example, patients with a HOXA2 gene mutation have microtia, a shortened and narrowed auditory tunnel and cleft palate (Alasti et al., 2008); hereditary hand-food-genital syndrome is a result of HOXA13 mutations as well (Mortlock and Innis, 1997; Goodman et al., 2000).
Postnatal Expression of Hox in Stromal Cells: Memorizing Location and Supporting Regenerative Potency
Stromal cells of mesodermal origin, such as mesenchymal stromal cells (MSC), fibroblasts, smooth muscle cells (Chi et al., 2007), and preadipocytes (Gesta et al., 2006), have a mechanism to memorize their topographic location in the form of their Hox code.
Indeed, fibroblasts isolated from topographically different body regions are characterized by a stable Hox code reflecting initial localization (Chang et al., 2002; Ackema and Charité, 2008). Moreover, the pattern of Hox gene expression in the postnatal period resembles that during development. For example, Hox codes in fibroblasts from different tissues coincide with Hox codes of mesoangioblasts of respective somites during embryogenesis (Ackema and Charité, 2008).
Lineage tracing data shows that in the postnatal period certain Hox-positive MSC (for example, expressing Hoxa11) do not arise from Hox-negative ones, but originate from pre-existed mesoangioblasts (Pineault et al., 2019). Thus, the expression of Hox genes in tissue-resident MSC does not seem to “turn on” in the postnatal period but rather remains unrepressed in some cells after birth. This is consistent with a confirmed postulate that in embryonic development Hox genes are repressed irreversibly and recapitulation of their expression seems impossible under physiological conditions (Wang et al., 2009). In this case, MSC inhere Hox code of their progenitors and retain it throughout life. This data supports a predisposition that in the postnatal period, tissues may contain subpopulations of Hox-positive MSC originating from embryonic progenitors and retaining their Hox code.
The Hox code of MSC reflects not only their location and harbor within the body but also serves as a “fingerprint” of tissue from which the cells were isolated. Hox-positive status is a very stable characteristic of a cell: it persists in vitro after prolonged culture, during differentiation, and in presence of soluble factors secreted by cells with another Hox code (Ackema and Charité, 2008). Expression of Hox in MSC is strongly resistant to the influence of exogenous factors (soluble molecules, hypoxia, stress, cell-to-cell contacts, etc.).
It was shown (Leucht et al., 2008) that the Hox code in stem cells is preserved even after transplantation to a Hox-negative organ or tissue within the host. In contrast, Hox-negative cells can adopt the Hox-code from the Hox-positive environment and this may occur after their heterotopic transplantation, as well as in vitro after co-culture with Hox-positive cells. Human unrestricted somatic stem cells (USSC) isolated from cord blood are characterized by the absence of HOX expression yet they readily acquire a HOX code when co-cultured with Hox-positive MSC (Liedtke et al., 2013).
Pronounced stability of the Hox code in the postnatal period may be important for proper healing and tissue regeneration. Hox gene expression is locally enhanced at the site of healing cutaneous wound (Uyeno et al., 2001) or bone fracture (Rux et al., 2017), supporting the proposed importance of Hox gene expression in these processes. Indeed, Hox gene upregulation in the site of injury strongly correlates with the regeneration outcome. Recent work by Qu et al. (2020) on murine digit tip regeneration demonstrated that only the successful regeneration is accompanied by temporary upregulation of Hoxa13 and Hoxd13 genes – ones that regulate digit development in embryogenesis. Furthermore, Hox gene expression is critical for fibroblast-dependent mechanisms of wound healing. It was demonstrated by Hansen et al. (2003) using transgenic diabetic mice that exhibit diminished wound healing. Exogenous delivery of Hoxd3 gene to wound bed by plasmid injections accelerated wound closure in these mice which was mediated by robust increase of collagen production by fibroblasts.
Data from in vivo experiments suggest that a mismatch between expression patterns of Hox genes in the graft and its surroundings may lead to decreased graft survival (Dani et al., 2017). This was accurately demonstrated in a model of bone regeneration after heterologous transplantation (Leucht et al., 2008). In this study, fates of Hoxa11-positive MSC from the tibia and Hox-negative neural crest stem cells from the mandibula were investigated after transplantation either to a Hox-positive (tibia) or Hox-negative environment (mandibula). It was established that transplantation of Hox-negative stem cells to a Hoxa11-positive region led to the acquisition of Hoxa11-positive status by transplanted cells followed by successful healing. In contrast, transplantation of Hox11-positive cells to a Hox-negative microenvironment resulted in a drastic reduction of bone tissue regeneration.
Thus, the contribution of Hox-code in resident stromal cells to tissue repair becomes a basis for further assumptions on potential objects and mechanisms to be investigated in this field. We should notice that the role of Hox gene expression in regeneration/repair is expected to be the most prominent in tissues with strong regeneration capacity which will be discussed below.
Among MSCs, There Are Subpopulations Expressing Hox, and They Differ in Properties From Those That Do Not Express Hox
There is evidence that Hox-positive and Hox-negative subpopulations of stem or stromal cells may reside within the same tissue/organ. It was shown that these subpopulations, although residing in the same compartment, show distinct patterns of properties, for instance, specific in vitro differentiation abilities. Particularly, it has been shown that cord blood stem cells contain a subpopulation of USSC that do not express Hox and a pool of bone marrow-derived MSC that express Hox genes (HOXA9, HOXB7, HOXC10). This finding was concordant with unrestricted potency of USSC that can differentiate into cells of all three germ layers while cord blood MSC are limited to tissues of mesodermal origin (Liedtke et al., 2010). Vice versa bone marrow (HOX-positive) MSC, but not USSC (HOX-negative), readily undergo adipogenic differentiation in routine laboratory tests (Kögler et al., 2009).
Thus, it seems that the role of Hox gene expression in adult stromal and stem cells is not limited to storing positional information. Since Hox genes are master regulators of many processes, Hox code affects cell phenotype and, therefore, functional characteristics of a cell which allows claiming that Hox-positive and Hox-negative cells represent two distinct subpopulations. This is consistent with results (Bradaschia-Correa et al., 2019) that Hox status accurately defines transcriptomic differences and differentiation potential between periosteal MSC obtained from Hox-positive and Hox-negative anatomic sites. Recent findings by Rux et al. (2016) highlight a subpopulation of periosteal Hoxa11-positive MSC that was characterized as a progenitor-enriched subpopulation. The role of Hox11 genes in bone repair will be discussed below supporting this remarkable finding by animal tests in a model of bone fracture.
Another example of the Hox gene that serves as a discerning marker within one anatomic region is HOXC10. The placenta contains both decidua-derived MSC in which HOXC10 is highly expressed and amnion-derived MSC that lack its expression (Hwang et al., 2009). These two cell types demonstrate the dramatic difference in their potency and secretome composition (Kögler et al., 2004, 2005) supporting that presence or absence of a specific Hox may be used as a marker to discern between subpopulations of functionally different cells. Indeed, both MSC – from decidua and amnion – show a typical CD73/90/105 immunophenotype and fully comply with other criteria of MSC.
We have mentioned above that fibroblasts isolated from various regions of the body are characterized by different Hox codes reflecting their origin. This characteristic of cultured cells retains in vitro for a substantial period of time. However, this data was obtained by evaluation of Hox expression in a fibroblast culture after several passages (or after a 2-week colony formation assay), which raises a concern on whether a true physiological pattern of Hox expression can be reproduced in such kind of experiment. Isolation of MSC is a stress factor similar to tissue damage that affects the status of isolated cells including expression of key transcription factors. It was demonstrated that the phenotype of cultured MSC does not fully reflect the heterogeneity (including functional) of the cell population that existed in situ prior to isolation (Sacchetti et al., 2016). Indeed, selective conditions of culture medium may result in a proliferation of one subpopulation and loss of minor pools of cells or ones that fail to adapt to culture medium composition. Thus, over several passages, the composition and ratio of cellular subpopulations may change dramatically and where one may gain advantage another may be marginally eliminated. In addition, we have also mentioned that Hox-negative cells are able to “adopt” Hox expression patterns from surrounding cells both – in vivo and in vitro via an unestablished mechanism (Leucht et al., 2008; Liedtke et al., 2013). Therefore, it is possible that the entire primary culture, previously heterogeneous in Hox expression, may acquire a Hox-positive status over time in vitro.
Resident Hox-Positive MSC Determine the Structure and Organization of Stroma
Bone
Recent studies have revealed an important role of Hox11-positive stromal cells subpopulation in limb bone regeneration. During embryogenesis, Hox11 paralogues regulate the development of bones in the forearms and lower legs (zeugopod). In the postnatal period, a subset of Hoxa11-positive MSC resides in these parts of the skeleton. After a fracture, the expression of Hoxa11 drastically increases at the site of injury. It has been established that Hox11 genes are necessary for successful fracture healing both in early stages when Hox11 function is essential for maturation of chondrocytes and in later healing periods when remodeling of the extracellular matrix has been shown to be Hox11-dependent (Rux et al., 2016, 2017). Hox11 genes play an important role not only in fracture healing but also in normal bone turnover: Hox11-expressing MSC regulate osteocyte renewal, promoting maturation of osteoblasts and maintaining natural spatial organization of collagen fibrils in the bone (Song et al., 2020).
Loss-of-function experiments show that bone MSC lacking expression of Hox11 genes fail to completely differentiate to the osteogenic and chondrogenic lineages in vitro (Rux et al., 2017; Song et al., 2020). Bone marrow MSC isolated from different parts of the skeleton and therefore having a different Hox code also differ by the efficiency of adipogenic and osteogenic differentiation (Ackema and Charité, 2008). Thus, in MSC their Hox code does not only carry information about positional identity but also determines specific “bias” of multipotency in cells from different anatomical regions.
In mouse tibia, Hoxa11 paralogues are expressed exclusively in a subpopulation of periosteal MSC with a PDGFRα+/CD51+/LepR+ immunophenotype (Rux et al., 2016) and it was established that these Hoxa11-positive MSC are essential for normal fracture healing (Rux et al., 2017) supporting that this subpopulation is functionally distinct from Hoxa11-negative MSC of the tibia.
Thus, Hox-positive MSC are necessary for both normal bone renewal and fracture healing. Moreover, we may speculate that during fracture healing Hox-positive MSC may temporarily induce Hox expression in other cell subpopulations as these characteristics of Hox-positive-to-Hox-negative cells crosstalk were described in vitro in multiple types of stromal cells from other tissue.
Spleen
In recent study, Ueno et al. (2019) characterized a Hoxa11-positive subpopulation of MSC in neonatal mouse spleen. Experiments with ectopic transplantation of an embryonic spleen showed that MSC of this subpopulation differentiate into all three types of splenic stromal cells. After stroma formation, it undergoes repopulation by hematopoietic cells of the host finalizing ectopic spleen to a fully operational organ.
In humans, structural elements of the spleen also show a vivid potential to form heterotopically. There are reported cases of splenosis – a condition when autologous heterotransplantation of splenic cells occurs after the rupture of the organ’s capsule. Eventually, it results in ectopic formation of spleen tissue – typically in the abdominal cavity (Fremont and Rice, 2007). It is likely that HOXA11-positive MSC of the spleen may be crucial for the development of splenosis in humans via creating an ectopic stromal harbor for other cell types to build organ ex situ. This claim is supported by data in mice that Hoxa11-positive MSC can give rise to all types of spleen stromal cells.
Thus, Hoxa11 gene expression may be a marker of MSC that function as splenic stroma organizers, and their potency to rebuild spleen’s stromal portion is a strong intrinsic feature realized via a specific Hox-dependent gene expression profile.
Endometrium
Besides spleen, the human body has another structure that can “take root,” rebuild and function after autologous heterotransplantation – endometrium, the inner layer of the uterus.
Endometrium undergoes deep desquamation during every menstrual cycle and regenerates with a remarkable rapidity (within several days). This extraordinary ability of endometrium results in up to 200–300 cycles of complete regeneration over a woman’s lifespan and is mediated by specific properties of its stromal cells (endometrial MSC).
The capability of human endometrium to grow outside the uterus also underlies a serious condition known as endometriosis, in which endometrial tissue is ectopically formed in the abdominal cavity, on the surface of ovaries or even umbilicus. Endometriosis leads to hormonal disorders, infertility, and bleedings since ectopic tissue may undergo desquamation during the menstrual cycle (Vercellini et al., 2013).
Data on the potential role of Hox genes in endometrial renewal and ectopy are accumulated during recent decades and deserve an overview within the scope of this communication.
The development of the female reproductive system from the Mullerian duct is controlled by HOXA9-HOXA13 genes. In particular, morphogenesis of the uterus is strongly regulated by HOXA10 and HOXA11. In mice, impaired function of any of these genes leads to defects in a part of the reproductive system which they control during development (Taylor, 2000). In human development dysregulation of HOXA10 expression may occur under the influence of a nonsteroidal estrogen medication – diethylstilbestrol (DES) and xenoestrogens, such as methoxychlor and bisphenol A. Both latter substances were widely spread for common purposes like protection of livestock from insect parasites (methoxychlor) or plasticware manufacture (bisphenol A). Influence of these xenobiotics on the developing embryo leads to abnormalities of the reproductive system mediated by persistent impairment of Hox gene expression (Ma et al., 1998; Fei et al., 2005; Smith and Taylor, 2007).
In the postnatal period HOX expression, specifically HOXA10 and HOXA11, is retained in endometrium and in its resident MSC. Furthermore, there is a remarkable feature of endometrial MSC in which expression of HOXA10 and HOXA11 is regulated by steroid sex hormones: estrogen and progesterone.
During the menstrual cycle, plasma concentrations of estrogen and progesterone vary to regulate the switch of its phases. Expression of HOXA10 and HOXA11 in endometrial MSC also changes concordantly with undulations of hormone concentration (Figure 1). Expression of HOXA10 and HOXA11 factors is relatively low in the proliferative phase but increases and reaches its peak in the secretory phase and persists throughout menstruation (Taylor et al., 1998; Tang et al., 2006).
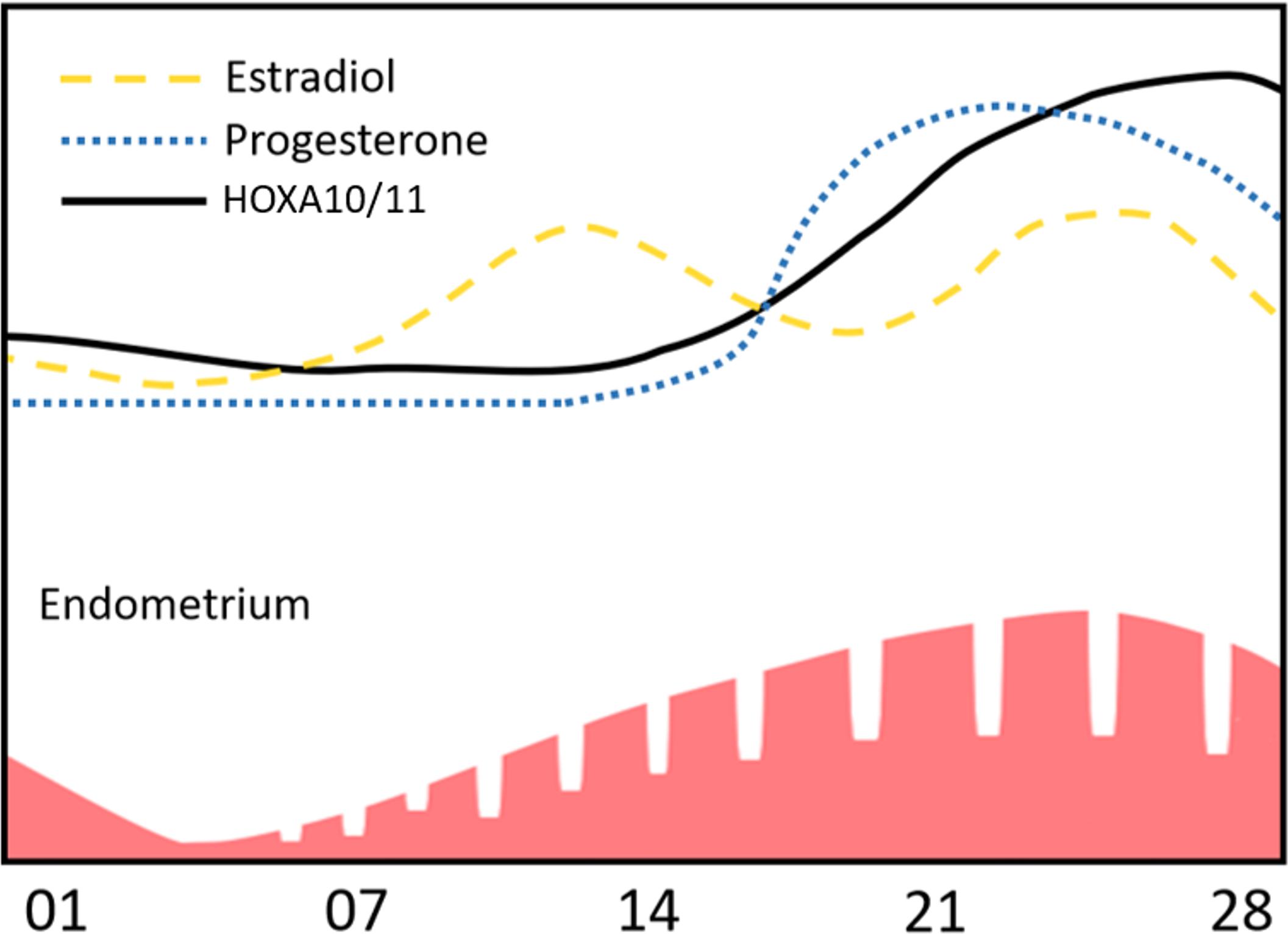
Figure 1. HOXA10 and HOXA11 expression dynamic is concordant to changes of estradiol and progesterone levels during the menstrual cycle.
After menstruation, an extensively vascularized wound surface is formed. Menstrual discharge blood has decreased coagulation leading to the absence of granulations and rapid epithelisation followed by formation of the endometrial stroma. The latter is possible due to the proliferation and differentiation of endometrial MSC accompanied by intensive vascularization of tissue layers. We hypothesize that increased HOXA10 and HOXA11 expression in MSC during menstruation is an evolutionary established response required for quick and efficient regeneration.
Decidualization and embryo implantation that require the adequate function of the endometrium are Hox/HOX-dependent processes as well (Du and Taylor, 2015). Subsequently, most reviews of HOX genes’ role in endometrial function mainly focus on the relation of HOX family to female fertility.
We would like to draw attention to another aspect of HOX gene expression that can be stipulated as a putatively pivotal role of HOX-positive stromal cells in regeneration of the endometrium. It should be noted that the female reproductive system is one of the few regions in the adult organism that is characterized by a relatively high basal HOX gene expression compared to other body parts (Taylor, 2000). We suppose that elevated basal HOXA expression in the endometrium may reflect a specific higher threshold for enhanced control of regeneration and retainment of cell program during multiple cycles of its renewal throughout life.
No genetic disease caused by mutations of the HOXA10/11 genes is known in humans, but there is evidence that a number of diseases of the female reproductive system such as hydrosalpinx, polycystic ovary syndrome and endometriosis are accompanied by decreased expression of HOXA10 and HOXA11 in the endometrium. Remarkably numerous and accurate studies show this for endometriosis (Wu et al., 2005; Du and Taylor, 2015). We want to pay special attention to the relation of impaired expression of HOXA10 and HOXA11 to endometriosis since this disease involves the ability of the endometrial stroma to self-organize ectopically, i.e., to “ignore” conditions of an ectopic environment.
We suggest that decreased expression of HOXA10/HOXA11 in endometrial stromal cells may be a causal factor in the development of endometriosis. According to data on the interaction between a graft and a host with different Hox codes, lack of Hox expression in the transplant allows it to successfully engraft – either in a Hox-positive or Hox-negative host environment. Therefore, decreased expression of HOXA10/HOXA11 genes results in partial loss of HOX code “identity” in endometrial cells facilitating its ectopy. It is of particular interest that the suppression of HOXA10 results in increased autophagy proteins (beclin-1 and LC3-II) expression in endometrial tissue (Zheng et al., 2018). We suppose that it reflects a protective mechanism for clearance of cells with “loss of identity”, and that its failure results in the survival of ectopic endometrial cells with suppressed HOXA10/11. Being moved into the abdominal cavity they may adopt local HOX conditions and by unknown means avoid intrinsically activated autophagy to form endometrial tissue in an ectopic location.
Due to findings on Hoxa11-positive periosteum MSC, it is known that Hox gene functioning in stromal cells is of great importance for maintaining the normal structure of the stroma. In patients with endometriosis, the architecture of eutopic endometrium is impaired, including increased surface epithelium heterogeneity and reduced endometrial thickness (reviewed in Sharpe-Timms, 2001), which can also be caused or mediated by altered HOX expression in stromal cells of the endometrium.
Thus, available data suggests that the expression of HOXA genes in MSC of the endometrium ensures its normal functioning and regeneration during the menstrual cycle. We suggest that a HOXA-positive MSC subpopulation in the endometrium is critical for controlling its physiological regeneration after damage, as well as for maintaining the normal structure.
Conclusion
Homeobox genes are critical during embryonic development of many animals. Expression of Hox is known to persist in many tissues in the postnatal period suggesting the role of these genes not only during development but also for the functioning of tissues throughout life. The tissue-specific pattern of Hox gene expression is inherent in stromal/stem cells of mesenchymal origin whose role in physiological renewal and regeneration is well-established in recent decades (Figure 2).
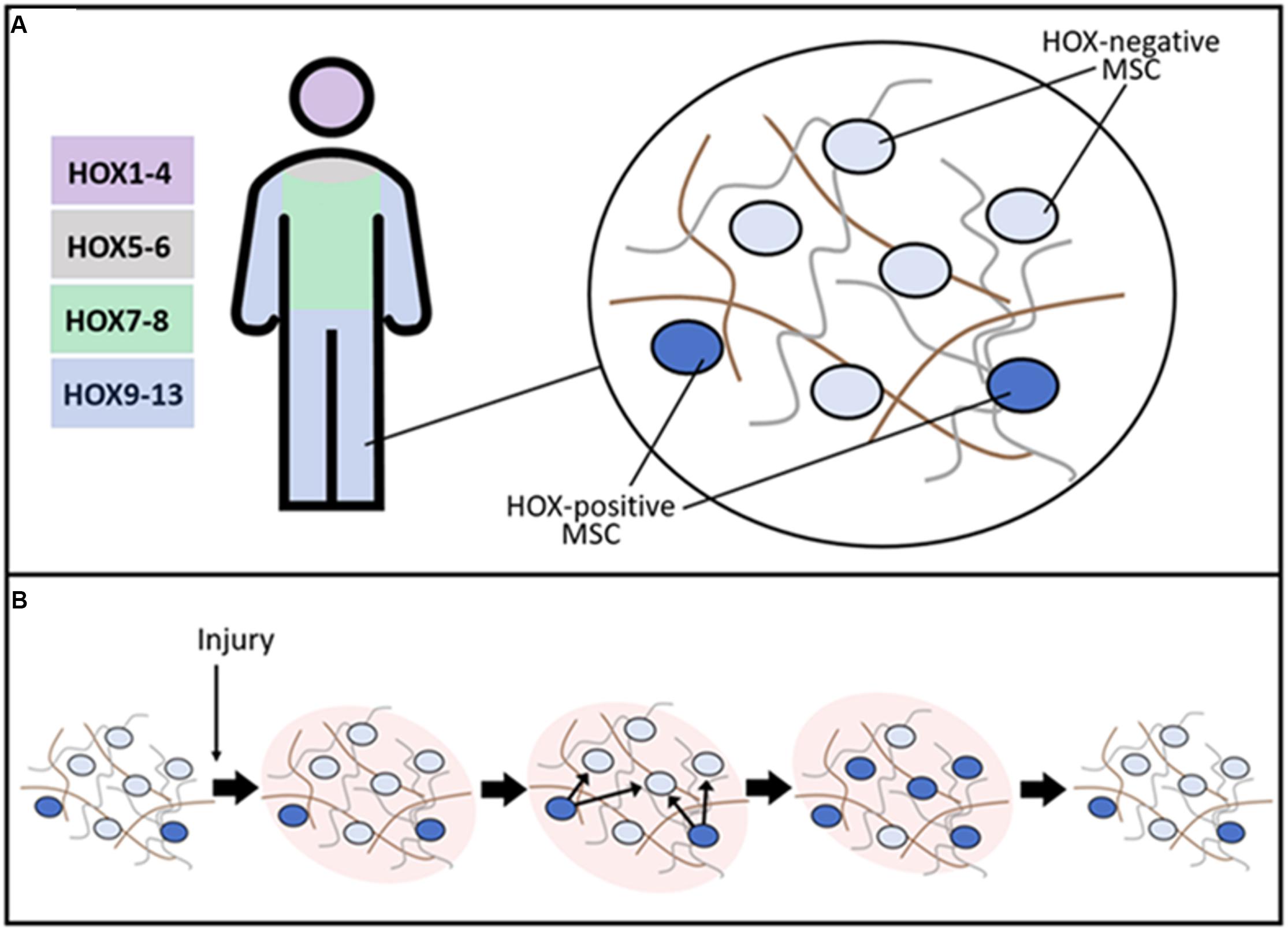
Figure 2. Putative role of resident HOX-positive MSC in the maintenance of stroma tissue-specific structure. (A) After embryogenesis, HOX gene expression is conserved in a distinct subpopulation of MSC. The schematic representation of HOX codes in distinct body parts is present. In normal tissue, a small HOX-positive subpopulation of MSC coordinates matrix turnover processes supporting normal architecture of stroma during physiological tissue renewal. (B) After damage, HOX-positive MSC are activated and induce expression of HOX genes in neighboring cells leading to the restoration of the initial structure of tissue stroma and facilitating regeneration.
We believe that the generally adopted Hox code hypothesis and the role of these genes in supporting postnatal cell identity might lead us to a particularly important direction in the study of human regenerative biology. Key points of this hypothetical direction are summarized below:
1. In adult organisms, resident MSC represent a highly heterogeneous cell population with variable differentiation potency, sensitivity to regulatory stimuli, and genome expression. Recent studies demonstrated the existence of specific subpopulations of MSC necessary for the organization of normal tissue stroma with its tissue-specific features. Presumably, subpopulations of MSC retaining Hox expression may take specialized functions associated with the organization of the tissue-specific structure of the stroma. Our hypothesis relies on the expression of Hox genes as a specific feature of this subpopulation as far as resident MSC include cells of both types – highly expressing Hox genes and cells that lack Hox expression.
2. In humans, HOX-positive MSC subpopulations are crucial for maintaining and reconstructing stroma and have been identified within tissues that demonstrate a remarkable ability for regeneration (bone and spleen). We suggest that resident Hox-positive MSC are leading organizers of stroma renewal and tissue regeneration in other tissues as well and highlight a direction of research focused on the endometrium.
3. The peculiar feature of spleen and endometrium is ectopic growth which indicates a potent ability of cells within these tissues to rebuild a functional environment and give rise to organs (e.g., splenosis). We suggest that Hox expression in a certain subpopulation of MSC in these tissues mediates their ectopic growth and efficient regeneration. Probably, identification of this subpopulation might be within reach in the endometrium as a feasible model object undergoing massive desquamation and reconstruction several hundred times. Data has been accumulated in favor of the assumption that Hox-positive stromal cells of the endometrium are important for its successful regeneration. Study of Hox patterns and their role in endometrial regeneration will allow more detailed insight into the functions of Hox-positive MSC and will expand our understanding of postnatal morphogenesis.
From a methodological point of view identification of crucial Hox genes using knockout or transgenic models may be a complicated mission due to functional redundancy of Hox paralogues. One may fail to exactly define an in vivo role of a given Hox using its knockout. To achieve a “loss of function” status with an obvious morphological outcome, a strain of animals with mixed knockouts and lacking several Hox genes may be required. In addition, conditional loss-of-function models are often obligatory – otherwise, it will be impossible to separate the effects of Hox disruption in embryonic development from defects that occur due to Hox gene suppression in the same structures in the postnatal period.
We expect our communication to trigger a certain amount of discussion and invite other authors and peers to comment on the potential of proposed direction of research and shall endeavor to decipher the role of Hox genes in regeneration using available models within our expertise (Eremichev et al., 2018; Nimiritsky et al., 2019).
Author Contributions
MK: conception and drafting of the manuscript. PM: editing the manuscript, proofing, and funding acquisition. Both authors contributed to the article and approved the submitted version.
Funding
This work has been supported by the Russian Science Foundation grants #19-75-00067 (figure preparation and access to publications) and #19-75-30007 (publisher fee).
Conflict of Interest
The authors declare that the research was conducted in the absence of any commercial or financial relationships that could be construed as a potential conflict of interest.
Acknowledgments
Authors express their gratitude to Dr. Ivan Kulakovskiy for fruitful discussion on bioinformatical approaches that may be used in further studies and to Mr. Mikhail Arbatskiy for assistance in bioinformatics surveys during manuscript revision.
References
Ackema, K. B., and Charité, J. (2008). Mesenchymal stem cells from different organs are characterized by distinct topographic Hox codes. Stem Cells Dev. 17, 979–1091. doi: 10.1089/scd.2007.0220
Alasti, F., Sadeghi, A., Sanati, M. H., Farhadi, M., Stollar, E., Somers, T., et al. (2008). A mutation in HOXA2 is responsible for autosomal-recessive microtia in an Iranian family. Am. J. Hum. Genet. 82, 982–991. doi: 10.1016/j.ajhg.2008.02.015
Bradaschia-Correa, V., Leclerc, K., Josephson, A. M., Lee, S., Palma, L., Litwa, H. P., et al. (2019). Hox gene expression determines cell fate of adult periosteal stem/progenitor cells. Sci. Rep. 9:5043. doi: 10.1038/s41598-019-41639-7
Chang, H. Y., Chi, J.-T., Dudoit, S., Bondre, C., van de Rijn, M., Botstein, D., et al. (2002). Diversity, topographic differentiation, and positional memory in human fibroblasts. Proc. Natl. Acad. Sci. U.S.A. 99, 12877–12882. doi: 10.1073/pnas.162488599
Chi, J.-T., Rodriguez, E. H., Wang, Z., Nuyten, D. S. A., Mukherjee, S., van de Rijn, M., et al. (2007). Gene expression programs of human smooth muscle cells: tissue-specific differentiation and prognostic significance in breast cancers. PLoS Genet. 3:164. doi: 10.1371/journal.pgen.0030164
Dani, C., Foissac, R., Ladoux, A., and Chignon-Sicard, B. (2017). Autologous fat grafts: can we match the donor fat site and the host environment for better postoperative outcomes and safety? Curr. Surg. Rep. 5:14. doi: 10.1007/s40137-017-0178-1
Du, H., and Taylor, H. S. (2015). The role of Hox genes in female reproductive tract development, adult function, and fertility. Cold Spring Harb. Perspect. Med. 6:023002. doi: 10.1101/cshperspect.a023002
Eremichev, R. Y., Makarevich, O. A., Alexandrushkina, N. A., Kulebyakin, K. Y., Dyikanov, D. T., and Makarevich, P. I. (2018). Menstrual-blood serum displays an antifibrotic effect on human endometrial mesenchymal stromal cells. Cell Tiss. Biol. 12, 281–288. doi: 10.1134/S1990519X1804003X
Fei, X., Chung, H., and Taylor, H. S. (2005). Methoxychlor disrupts uterine Hoxa10 gene expression. Endocrinology 146, 3445–3451. doi: 10.1210/en.2005-0341
Fremont, R. D., and Rice, T. W. (2007). Splenosis: a review. South Med. J. 100, 589–593. doi: 10.1097/SMJ.0b013e318038d1f8
Gesta, S., Blüher, M., Yamamoto, Y., Norris, A. W., Berndt, J., Kralisch, S., et al. (2006). Evidence for a role of developmental genes in the origin of obesity and body fat distribution. Proc. Natl. Acad. Sci. U.S.A. 103, 6676–6681. doi: 10.1073/pnas.0601752103
Goodman, F. R., Bacchelli, C., Brady, A. F., Brueton, L. A., Fryns, J. P., Mortlock, D. P., et al. (2000). Novel HOXA13 mutations and the phenotypic spectrum of hand-foot-genital syndrome. Am. J. Hum. Genet. 67, 197–202. doi: 10.1086/302961
Hansen, S. L., Myers, C. A., Charboneau, A., Young, D. M., and Boudreau, N. (2003). HoxD3 accelerates wound healing in diabetic mice. Am. J. Pathol. 163, 2421–2431. doi: 10.1016/S0002-9440(10)63597-3
Hwang, J. H., Seok, O. S., Song, H. R., Jo, J. Y., and Lee, J. K. (2009). HOXC10 as a potential marker for discriminating between amnion- and decidua-derived mesenchymal stem cells. Cloning Stem. Cells 11, 269–279. doi: 10.1089/clo.2008.0068
Kmita, M., and Duboule, D. (2003). Organizing axes in time and space; 25 years of colinear tinkering. Science 301, 331–333. doi: 10.1126/science.1085753
Kögler, G., Critser, P., Trapp, T., and Yoder, M. (2009). Future of cord blood for non-oncology uses. Bone Marrow Transplant. 44, 683–697. doi: 10.1038/bmt.2009.287
Kögler, G., Radke, T. F., Lefort, A., Sensken, S., Fischer, J., Sorg, R. V., et al. (2005). Cytokine production and hematopoiesis supporting activity of cord blood-derived unrestricted somatic stem cells. Exp. Hematol. 33, 573–583. doi: 10.1016/j.exphem.2005.01.012
Kögler, G., Sensken, S., Airey, J. A., Trapp, T., Müschen, M., Feldhahn, N., et al. (2004). A new human somatic stem cell from placental cord blood with intrinsic pluripotent differentiation potential. J. Exp. Med. 200, 123–135. doi: 10.1084/jem.20040440
Lappin, T. R., Grier, D. G., Thompson, A., and Halliday, H. L. (2006). HOX genes: seductive science, mysterious mechanisms. Ulster Med. J. 75, 23–31.
Leucht, P., Kim, J. B., Amasha, R., James, A. W., Girod, S., and Helms, J. A. (2008). Embryonic origin and Hox status determine progenitor cell fate during adult bone regeneration. Development 135, 2845–2854. doi: 10.1242/dev.023788
Liedtke, S., Buchheiser, A., Bosch, J., Bosse, F., Kruse, F., Zhao, X., et al. (2010). The HOX code as a “biological fingerprint” to distinguish functionally distinct stem cell populations derived from cord blood. Stem Cell Res. 5, 40–50. doi: 10.1016/j.scr.2010.03.004
Liedtke, S., Freytag, E. M., Bosch, J., Houben, A. P., Radke, T. F., Deenen, R., et al. (2013). Neonatal mesenchymal-like cells adapt to surrounding cells. Stem Cell Res. 11, 634–646. doi: 10.1016/j.scr.2013.04.001
Ma, L., Benson, G. V., Lim, H., Dey, S. K., and Maas, R. L. (1998). Abdominal B (AbdB) Hoxa genes: regulation in adult uterus by estrogen and progesterone and repression in mullerian duct by the synthetic estrogen diethylstilbestrol (DES). Dev. Biol. 197, 141–154. doi: 10.1006/dbio.1998.8907
Moens, C. B., and Selleri, L. (2006). Hox cofactors in vertebrate development. Dev. Biol. 291, 193–206. doi: 10.1016/j.ydbio.2005.10.032
Mortlock, D. P., and Innis, J. W. (1997). Mutation of HOXA13 in hand-foot-genital syndrome. Nat. Genet. 15, 179–180. doi: 10.1038/ng0297-179
Nimiritsky, P. P., Eremichev, R. Y., Alexandrushkina, N. A., Efimenko, A. Y., Tkachuk, V. A., and Makarevich, P. I. (2019). Unveiling mesenchymal stromal Cells’. organizing function in regeneration. Int. J. Mol. Sci. 20:823. doi: 10.3390/ijms20040823
Picchi, J., Trombi, L., Spugnesi, L., Barachini, S., Maroni, G., Brodano, G. B., et al. (2013). HOX and TALE signatures specify human stromal stem cell populations from different sources. J. Cell Physiol. 228, 879–889. doi: 10.1002/jcp.24239
Pineault, K. M., Song, J. Y., Kozloff, K. M., Lucas, D., and Wellik, D. M. (2019). Hox11 expressing regional skeletal stem cells are progenitors for osteoblasts, chondrocytes and adipocytes throughout life. Nat. Commun. 10:3168. doi: 10.1038/s41467-019-11100-4
Qu, F., Palte, I. C., Gontarz, P. M., Zhang, B., and Guilak, F. (2020). Transcriptomic analysis of bone and fibrous tissue morphogenesis during digit tip regeneration in the adult mouse. FASEB J. 10, doi: 10.1096/fj.202000330R [Epub ahead of print].
Quinonez, S. C., and Innis, J. W. (2014). Human HOX gene disorders. Mol. Genet. Metab. 111, 4–15. doi: 10.1016/j.ymgme.2013.10.012
Rux, D. R., Song, J. Y., Pineault, K. M., Mandair, G. S., Swinehart, I. T., Schlientz, A. J., et al. (2017). Hox11 function is required for region-specific fracture repair. J. Bone Miner. Res. 32, 1750–1760. doi: 10.1002/jbmr.3166
Rux, D. R., Song, J. Y., Swinehart, I. T., Pineault, K. M., Schlientz, A. J., Trulik, K. G., et al. (2016). Regionally restricted Hox function in adult bone marrow multipotent mesenchymal stem/stromal cells. Dev. Cell 39, 653–666. doi: 10.1016/j.devcel.2016.11.008
Sacchetti, B., Funari, A., Remoli, C., Giannicola, G., Kögler, G., Liedtke, S., et al. (2016). No identical “mesenchymal stem cells” at different times and sites: human committed progenitors of distinct origin and differentiation potential are incorporated as adventitial cells in microvessels. Stem Cell Rep.orts 6, 897–913. doi: 10.1016/j.stemcr.2016.05.011
Seifert, A., Werheid, D. F., Knapp, S. M., and Tobiasch, E. (2015). Role of Hox genes in stem cell differentiation. World J. Stem Cells 7, 583–595. doi: 10.4252/wjsc.v7.i3.583
Sharpe-Timms, K. L. (2001). Endometrial anomalies in women with endometriosis. Ann. N. Y. Acad. Sci. 943, 131–147. doi: 10.1111/j.1749-6632.2001.tb03797.x
Smith, C., and Taylor, H. S. (2007). Xenoestrogen exposure imprints expression of genes (Hoxa10) required for normal uterine development. FASEB J. 21, 239–246. doi: 10.1096/fj.06-6635com
Song, J. Y., Pineault, K. M., Dones, J. M., Raines, R. T., and Wellik, D. M. (2020). Hox genes maintain critical roles in the adult skeleton. Proc. Natl. Acad. Sci. U.S.A 117, 7296–7304. doi: 10.1073/pnas.1920860117
Svingen, T., and Tonissen, K. F. (2006). Hox transcription factors and their elusive mammalian gene targets. Heredity 97, 88–96. doi: 10.1038/sj.hdy.6800847
Tang, Y., Wang, L., Lv, L., Su, B., Feng, X., Wang, N., et al. (2006). Differential expression of HOXA11 in human endometrial glandular epithelial and stromal cells. Reprod. Contracept. 11.
Taylor, H. S. (2000). The role of HOX genes in the development and function of the female reproductive tract. Semin. Reprod. Med. 18, 81–89. doi: 10.1055/s-2000-13478
Taylor, H. S., Arici, A., Olive, D., and Igarashi, P. (1998). HOXA10 is expressed in response to sex steroids at the time of implantation in the human endometrium. J. Clin. Invest. 101, 1379–1384. doi: 10.1172/JCI1057
Ueno, Y., Fujisaki, K., Hosoda, S., Amemiya, Y., Okazaki, S., Notsu, C., et al. (2019). Transcription factor Tlx1 marks a subset of lymphoid tissue organizer-like mesenchymal progenitor cells in the neonatal spleen. Sci. Rep. 9:20408.
Uyeno, L. A., Newman-Keagle, J. A., Cheung, I., Hunt, T. K., Young, D. M., and Boudreau, N. (2001). Hox D3 expression in normal and impaired wound healing. J. Surg. Res. 100, 46–56. doi: 10.1006/jsre.2001.6174
Vercellini, P., Viganò, P., Somigliana, E., and Fedele, L. (2013). Endometriosis: pathogenesis and treatment. Nat. Rev. Endocrinol. 10, 261–275. doi: 10.1038/nrendo.2013.255
Wang, K., Helms, J., and Chang, H. (2009). Regeneration, repair and remembering identity: the three Rs of Hox gene expression. Trends Cell Biol. 19, 268–275. doi: 10.1016/j.tcb.2009.03.007
Wu, Y., Halverson, G., Basir, Z., Strawn, E., Yan, P., and Guo, S. W. (2005). Aberrant methylation at HOXA10 may be responsible for its aberrant expression in the endometrium of patients with endometriosis. Am. J. Obstet. Gynecol. 193, 371–380. doi: 10.1016/j.ajog.2005.01.034
Keywords: Hox genes, mesenchymal stem/stromal cells, postnatal morphogenesis, regeneration, stroma, tissue renewal
Citation: Kulebyakina M and Makarevich P (2020) Hox-Positive Adult Mesenchymal Stromal Cells: Beyond Positional Identity. Front. Cell Dev. Biol. 8:624. doi: 10.3389/fcell.2020.00624
Received: 04 May 2020; Accepted: 22 June 2020;
Published: 31 July 2020.
Edited by:
Darius Widera, University of Reading, United KingdomReviewed by:
Philippe Bourin, Independent Researcher, Toulouse, FranceAleksandra Borodkina, Institute of Cytology (RAS), Russia
Francesco De Francesco, Azienda Ospedaliero Universitaria Ospedali Riuniti, Italy
Oleg Gusev, RIKEN, Japan
Copyright © 2020 Kulebyakina and Makarevich. This is an open-access article distributed under the terms of the Creative Commons Attribution License (CC BY). The use, distribution or reproduction in other forums is permitted, provided the original author(s) and the copyright owner(s) are credited and that the original publication in this journal is cited, in accordance with accepted academic practice. No use, distribution or reproduction is permitted which does not comply with these terms.
*Correspondence: Pavel Makarevich, pmakarevich@mc.msu.ru