Perinatal derivatives: How to best characterize their multimodal functions in vitro. Part C: Inflammation, angiogenesis, and wound healing
- 1Regenerative Medicine Group, Research Institute Hospital 12 de Octubre (imas12), Madrid, Spain
- 2Center for Advanced Studies and Technology (CAST), Department of Medical, Oral and Biotechnological Sciences, University G. d’Annunzio Chieti-Pescara, StemTech Group, Chieti, Italy
- 3University of Ljubljana, Faculty of Medicine, Institute of Cell Biology, Ljubljana, Slovenia
- 4Laboratorio de Regeneración, Oncología Molecular y TGF-β, IMIB-Arrixaca, Murcia, Spain
- 5Service de Chirurgie Maxillo-Faciale, Stomatologie et Odontologie Hospitalière, CHU Besançon, Besançon, France
- 6Laboratoire de Nanomédecine, Imagerie, Thérapeutique EA 466, Université Bourgogne Franche-Comté, Besançon, France
- 7Institute for Transfusion Medicine, University Hospital Essen, University of Duisburg-Essen, Essen, Germany
- 8Department of Women’s and Children’s Health, University of Padova, Padova, Italy and Foundation Institute of Pediatric Research Fondazione Città Della Speranza, Padova, Italy
- 9Centro di Ricerca E. Menni, Brescia, Italy
- 10Department of Life Science and Public Health, Università Cattolica del Sacro Cuore, Rome, Italy
- 11Fondazione Policlinico Universitario “Agostino Gemelli” IRCCS, Rome, Italy
- 12Systems Biology Ireland, School of Medicine, Conway Institute, University College Dublin, Dublin, Ireland
- 13Division of Cell Biology, Histology and Embryology, Gottfried Schatz Research Center, Medical University of Graz, Graz, Austria
Perinatal derivatives (PnD) are birth-associated tissues, such as placenta, umbilical cord, amniotic and chorionic membrane, and thereof-derived cells as well as secretomes. PnD play an increasing therapeutic role with beneficial effects on the treatment of various diseases. The aim of this review is to elucidate the modes of action of non-hematopoietic PnD on inflammation, angiogenesis and wound healing. We describe the source and type of PnD with a special focus on their effects on inflammation and immune response, on vascular function as well as on cutaneous and oral wound healing, which is a complex process that comprises hemostasis, inflammation, proliferation (including epithelialization, angiogenesis), and remodeling. We further evaluate the different in vitro assays currently used for assessing selected functional and therapeutic PnD properties. This review is a joint effort from the COST SPRINT Action (CA17116) with the intention to promote PnD into the clinics. It is part of a quadrinomial series on functional assays for validation of PnD, spanning biological functions, such as immunomodulation, anti-microbial/anti-cancer activities, anti-inflammation, wound healing, angiogenesis, and regeneration.
1 Introduction
Stem and progenitor cells from various tissues are increasingly being used in regenerative medicine and immunotherapies. For the expansion of these strategies, many researchers are putting effort on developing methods and technologies based on perinatal derivatives (PnD), which comprise perinatal tissues and thereof derived cells and secretomes. Besides perinatal mesenchymal stromal cells (MSC), also other perinatal tissues and cells, such as human amniotic membrane epithelial cells (hAEC), human amniotic fluid cells (hAFC), human parietal decidua (hPD) cells, or processed membranes (human amniotic membrane (hAM) or human amnio-chorionic membrane (hACM)) were already used or are under investigation for therapeutical treatment. Perinatal endothelial cells (EC) are predominantly isolated from the human umbilical vein (HUVEC), due to easier obtention and higher yield (Silini et al., 2020). Consequently, research using HUVEC is gaining momentum in the PnD field for the development of technologies useful for the vascular component regeneration and as an in vitro platform to validate the functional role of other PnD in the context of inflammation and angiogenesis (Pipino et al., 2022).
PnD portrait important advantages over products obtained from adults regarding their naïvity, availability and accessibility. This review aims to address the most widely studied PnD with a special focus on their functional in vitro validation in the field of inflammation, angiogenesis and cutaneous as well as oral wound healing to support their use in specific pre-clinical and clinical settings.
The anti-inflammatory properties of PnD make them highly attractive for treating inflammatory, autoimmune, and degenerative diseases (Silini et al., 2013; Cargnoni et al., 2021; Yang et al., 2021). Inflammation is the body’s defense mechanism to harmful stimuli, such as pathogens or damaged tissues. When the immune system is activated, inflammatory cells transmigrate from the vessels into damaged tissues (Medzhitov, 2008). There are two types of inflammation, acute inflammation characterized by a rapid response and chronic inflammation, which is a persistent but slowly evolving response. Immune cells from both, innate and adaptive response, play important roles in the pathogenesis of inflammatory diseases (Marshall et al., 2018). Chemokines as produced by damaged tissue recruit different immune cells, including eosinophils, macrophages, neutrophils, and T lymphocytes, contributing to inflammation. The resolution of inflammation is carried out by acting at different levels, such as through a decrease in the proliferation and maturation of immune cells, an increase in phagocytosis and apoptosis of immune cells, and an inhibition of the secretion of proinflammatory mediators. To behave as an anti-inflammatory agent, PnD should sense the inflammatory conditions, express and secrete anti-inflammatory molecules, and interact with immune cells. Accumulating evidence from preclinical and clinical trials indicates that PnD exert anti-inflammatory therapeutic effects in numerous autoimmune and inflammatory diseases such as graft versus host disease (GVHD), rheumatoid arthritis, multiple sclerosis, systemic lupus erythematosus, and respiratory diseases (Ringden et al., 2018; Yang et al., 2021; Ma et al., 2022).
Inflammation and angiogenesis, the formation of new blood vessels, are critical steps in the complex process of wound healing. Hemostasis, the first phase of wound healing, leads to vasoconstriction and the formation of a blot clot that stops bleeding. The following inflammatory phase leads to the accumulation of neutrophils and macrophages at the wound to defend bacteria and remove foreign substances, respectively. In addition, the adaptive immune system is activated. In the subsequent proliferative phase, fibroblasts multiply and deposit extracellular matrix, and angiogenesis, a critical component of acute wound healing, occurs. Re-epithelialization takes place as epithelial cells migrate from the periphery to the center of the wound. Further extracellular matrix deposition leads to a transition of the inflammatory state to a growth state, and a cross-linking of collagen, as well as scar maturation, finally leads to remodeling (Diegelmann and Evans, 2004; Dash et al., 2018). Similar processes have also been described in mucosal wound healing. Delayed wound healing is often due to insufficient blood supply based on impaired wound revascularization (Demidova-Rice et al., 2012). Further, chronic, non-healing wounds are detained in a self-perpetuating inflammatory stage that hinders progression to proliferation (Stojadinovic et al., 2008). Thus, chronic wounds are still a challenge to treat, although already more than a hundred years ago the hAM was used as a biological dressing for treating burns and skin ulcerations. Since then, while its use for the treatment of e.g. ocular ulcers is popular worldwide, its suitability for the management of skin ulcers is less well-recognized (Castellanos et al., 2017).
Improved techniques for tissue preservation, as well as advances in isolation and culture procedures for PnD cells facilitate the way for early phase clinical trials in diverse indications, such as the application of PLacental-eXpanded (PLX-PAD) stromal cells for the muscle recovery after hip arthroplasty (Winkler et al., 2022), multiple sclerosis (Lublin et al., 2014), pulmonary fibrosis (Chambers et al., 2014), and also COVID-19 (Hashemian et al., 2021; Sadeghi et al., 2021).
The focus of this review lies in the functional in vitro testing of PnD, which should be carried out before their application in animal models and subsequent clinical studies, to produce a treatment which is safe, effective and available for patients.
2 Mechanism of action
2.1 PnD effects on inflammation and the immune response
PnD can downregulate inflammation by acting on several key players in innate and adaptative immunity. PnD reduce inflammatory conditions in vitro by suppressing the proliferation, secretion of inflammatory cytokines, and cytotoxic activity of different immune cell subpopulations, as well as by inducing T cells and monocytes to acquire anti-inflammatory functions. These anti-inflammatory effects can be measured in vitro in cell-to-cell contact studies between PnD and immune cells (Erkers et al., 2013; Vellasamy et al., 2013), in non-contact transwell studies via secretion of soluble factors or by using conditioned medium (CM) of PnD cultures (Magatti et al., 2008; Gu et al., 2013). It is also important to understand that PnD can act constitutively (Magatti et al., 2008; Rossi et al., 2012; Pianta et al., 2015), and they can react to local inflammatory stimuli. To functionally assess this, an in vitro inflammatory environment created by the addition of Interferon γ (IFNγ) and/or Tumour Necrosis Factor (TNF), Interleukin (IL)1β, IL6, granulocyte-macrophage- colony stimulating factor (GM-CSF) or a mixture of all of these can be used to see if the cell morphology, size, immunophenotype and proliferation capabilities of PnD are altered (Mebarki et al., 2021). Furthermore, this simulated pro-inflammatory environment can be used to study the inhibitory capacity of PnD on T cell proliferation (Mebarki et al., 2021).
2.1.1 Assessment of PnD effects on the innate immune response
Innate immune cells, such as macrophages, neutrophils, dendritic cells, and natural killer cells can be regulated by PnD (Abumaree et al., 2017; De La Torre et al., 2018; Edinger et al., 2018; Torre and Flores, 2020). Anti-inflammatory assays are focused on the evaluation of cell viability, maturation and/or activation of immune cells.
Macrophages play an important role in the initiation, preservation, and cessation of inflammation through production of several cytokines and growth factors. Generally based on their cytokine profile and cell surface markers (see below) macrophages show two different phenotypes: the predominantly pro-inflammatory M1 phenotype and the generally anti-inflammatory M2 phenotype The macrophage activity switches from pro-inflammatory (M1) to anti-inflammatory (M2) during the inflammatory process, and an imbalance of this change at the end of an inflammatory process is associated with a range of inflammatory diseases (Fujiwara and Kobayashi, 2005; Lee, 2018). Some studies have reported that PnD can induce anti-inflammatory effects by regulating macrophage functions. To study whether direct or indirect contact of PnD with macrophages induces a switch to an M2-like anti-inflammatory phenotype, a transwell chamber membrane culture system can be used. The effects of adding CM of unstimulated PnD to differentiated monocytes can also be evaluated. Firstly, CD14+ monocytes are isolated from peripheral blood mononuclear cells (PBMC) and differentiated into M1 macrophages by the addition of GM-CSF or lipopolysaccharide (LPS). Secondly, macrophages and PnD are co-cultured in the transwell chamber system and the M2 phenotype can be characterized by flow cytometric analyses of morphological changes and the expression of M2 cell surface markers (CD14, CD36, CD86, CD163, CD204, CD206, B7-H4 and CD11 b), the co-stimulatory molecules (CD40, CD80 and CD86) and the co-inhibitory molecules (CD273, CD274 and B7-H4), and major histocompatibility complex (MHC-II) molecules (Abumaree et al., 2013; Huang et al., 2019). The anti-inflammatory phenotype in M2-like macrophages can also be evaluated by qRT-PCR looking for an increased expression of the M2 cell surface markers, and by Enzyme-Linked Immunosorbent Assay (ELISA) examining increased secretion of IL-10 and arginase (Arg)-1, and decreased secretion of IL-1β, IL-12 (p70) and MIP-1α, TNF, inducible nitric oxide synthase (iNOS), and IL-6 (Abumaree et al., 2013; Huang et al., 2019). Furthermore, since the clearance of apoptotic cells is critical for the resolution of inflammation, it is possible to assess whether PnD increase the phagocytic activity of M2 macrophages. Phagocytosis of apoptotic cells is then evaluated by fluorescence microscopy (Abumaree et al., 2013) or the ability of M2 macrophages to take up zymosan particles as measured with the CytoSelect™ Phagocytosis Kit (Abumaree et al., 2013). The immortalized human monocyte/macrophage cell line THP-1 is another useful cell model system to study the effect of PnD on activated macrophages (He et al., 2012). THP-1 cells are pre-treated with phorbol-12-myristate-13-acetate (PMA) to induce macrophage differentiation and then activated with LPS (Shu et al., 2015). After direct coculture with PnD or their CM, Western blot can be used to detect diminished levels of the proinflammatory cytokines TNF and IL-1β, and the inhibition of the mitogen-activated protein kinase (MAPK)/NF-κB signaling pathway (Hu et al., 2013). Macrophages can induce changes in the phenotype of PnD, and these changes can be evaluated by flow cytometry as an induction in secretion of the inflammatory proteins IL8, IL-12, and Monocyte Chemoattractant Protein 1 (MCP-1), and of anti-inflammatory proteins IL-10, indoleamine-pyrrole-2,3-oxygenase (IDO) and B7H4 (Abumaree et al., 2013).
Neutrophils along with macrophages provide the innate cell-mediated immunity and inflammatory responses at sites of injury. Indeed, cytokines secreted by macrophages attract neutrophils to the injured area to initiate the inflammatory response that in turn will recruit additional innate immunity cells and molecules. To assess the role of PnD in neutrophil and macrophage migration in vitro, their chemotactic activity towards recombinant macrophage inflammatory protein (MIP)-2 could be tested in the presence of CM of PnD using migration assay chambers (Li et al., 2005). To study whether PnD induce neutrophil-like N2-type polarization in an inflammatory environment, a trans-well system is used under stimulation of neutrophils with pro-inflammatory LPS. In these in vitro systems, two different cell models of neutrophils can be used, neutrophils freshly isolated from human blood, and the human leukemia cell line (HL-60) that is an incomplete differentiated cell line that must undergo differentiation to become functional neutrophils (Babatunde et al., 2021), HL-60 cells differentiate into neutrophils with DMSO, and subsequent stimulation with LPS induces a polarized N1 phenotype. qPCR and Western blot are used to determine the neutrophil surface molecules associated with a polarized N2-phenotype as well as the levels of secreted pro-inflammatory and anti-inflammatory factors (Wang et al., 2020). Furthermore, PnD also have direct effects on major functions of neutrophils. Co-culture of freshly isolated human blood neutrophils with PnD can serve to demonstrate the increased phagocytic capacity of neutrophils and, at the same time, their decreased oxidative burst capacity as another method to validate the anti-inflammatory function of PnD (Magatti et al., 2018; Alipour et al., 2020). However, there are not many studies addressing the anti-inflammatory role of PnD on neutrophils despite the increasing evidence revealing unsuspected roles of neutrophils in many physiological and pathological processes (Nicolas-Avila et al., 2017), and more studies are needed.
As part of the anti-inflammatory effects of PnD, the inhibition of the differentiation and maturation of monocytes to dendritic cells (DC) has been reported. Monocytes isolated from peripheral blood are differentiated to immature dendritic cells (iDC) in the presence of IL-4 and GM-CSF, and iDCs develop into mature dendritic cells (mDC) in the presence of LPS. Both processes are inhibited by PnD, by direct or indirect contact with the DC using a transwell culture system, or by the addition of CM of PnD (Magatti et al., 2009; Croxatto et al., 2014; Abomaray et al., 2015). Furthermore, PnD induce the switch to an anti-inflammatory phenotype in both iDC and mDC. This change can be measured by flow cytometry, observing a decrease in the expression of co-stimulatory molecules (CD40, CD80, CD83 and CD86) and an increase in the expression of co-inhibitory molecules (B7H3, B7H4, CD273, CD274), and of the immunosuppressive enzyme IDO (Abomaray et al., 2015). Likewise, PnD block the production of pro-inflammatory cytokines by iDC and mDC such as TNF, IL-6, IL-12 and IL-23, IFNγ, C-X-C motif chemokine ligand 10 (CXCL10), CXCL9 and chemokine C-C motif ligand (CCL5) while the secretion of IL-10 is increased, as determined in cell culture supernatants using the ELISA assay (Magatti et al., 2009; Abomaray et al., 2015; Magatti et al., 2015). Phagocytic activity of iDC is essential for the elimination of the cellular components released after a tissue injury, which -if accumulated-would cause inflammation. Phagocytic activity of iDC is induced by PnD and can be determined by CytoSelect™ phagocytosis functional assay (Abomaray et al., 2015). DC are antigen presenting cells capable of inducing an efficient T cell response to specific antigens, being an important mediator of the innate and adaptive immune response. To directly determine the impact of DC co-cultured with PnD on T-cell proliferation, a mixed lymphocyte reaction (MLR) is used, and T cell proliferation can be quantified by 3H thymidine uptake (Croxatto et al., 2014; Magatti et al., 2015; Talwadekar et al., 2015). Then, PnD induce an anti-inflammatory phenotype on DC which will down-regulate the activity and proliferation of T cells, presenting in vitro evidence of their role in modulating the immune response in immunological diseases.
In most of these studies, monocytes are isolated from human PBMC, and only few of them used an established cell line model. Although it is well known that the composition of human PBMC depend on the donor’s physiological status and its use supposes a high variability across donors (Kleiveland, 2015), they are the gold standard tool for isolating monocytes to investigate the role of immune cells in inflammatory diseases. However, although monocyte-like cells do not fully replicate the genotypic and phenotypic properties of human peripheral blood monocytes (Riddy et al., 2018), they represent a simplified surrogate and readily available cell model, especially when human blood is not available, which makes them a valuable and convenient model for repeated testing.
Natural killer cells (NK cells) are large granular lymphocytes with cytotoxic activity against injured cells, and their activation results in the secretion of pro-inflammatory cytokines, suggesting that NK cells can either drive inflammation or restrain adaptive immune responses to prevent excessive inflammation or even autoimmunity (Brandstadter and Yang, 2011; Zitti and Bryceson, 2018; Moloudizargari et al., 2021). Analysis of cellular crosstalk between PnD and NK cells has yielded contradictory results probably due to either the different origin of PnD and the different microenvironmental conditions to which the cells are exposed during pregnancy (Abumaree et al., 2019) or due to different PnD:NK and NK/tumor cells ratios used in each study (Moloudizargari et al., 2021), or to the different cell type used for cytotoxicity testing (Boissel et al., 2008). Despite this, it is valuable to know how PnD interact with NK cells in culture. It is possible to determine whether the coculture modulates NK cell proliferation, NK-activated receptor expression (NKp30, NKp44, NKp46, NKG2D, and CD69) and/or NK cell cytotoxicity on tumor cell lines (Chatterjee et al., 2014; Croxatto et al., 2014; Li et al., 2015). Furthermore, the profile of cytokine production by NK cells may be affected by coculture with PnD resulting in changes in the expression of pro-inflammatory molecules that can be measured by RT-PCR and/or ELISA assay (Li et al., 2015; Abumaree et al., 2019). Furthermore, the suppressive activity of PnD can be measured by their release of anti-inflammatory molecules such as IL-10 and prostaglandin E2 (PGE2) when cocultured with NK cells (Chatterjee et al., 2014; Li et al., 2015). Interestingly, IL-2 preactivated NK cells exert cytotoxic effects on PnD despite expressing high levels of HLA-I, whereas nonactivated NK cells did not lyse the PnD, suggesting that an inflammatory environment is necessary for the NK cytolytic activity against PnD (Abumaree et al., 2018; Abumaree et al., 2019).
2.1.2 Assessment of PnD effects on the adaptative immune response
PnD have anti-inflammatory properties acting on the adaptive response and these are measured as their ability to inhibit the proliferation and cytokine production of T lymphocytes as well as their ability to modulate T cell differentiation. The anti-inflammatory properties of PnD can be studied using in vitro models of inflammatory diseases, such as ocular allergic inflammation (Solomon et al., 2005), inflammation of human middle ear epithelial cells (HMEEC) by airway pollutants (Kim et al., 2019; Kim et al., 2020), atopic dermatitis (Kim et al., 2020) or demyelinating diseases (Bravo et al., 2016). The anti-inflammatory effects of PnD after co-culture can be analyzed by fibroblast proliferation measured with the [3H]-thymidine incorporation assay (Solomon et al., 2005) or T cell proliferation measured by the MTT assay (Bravo et al., 2016). The inhibitory effects can be analyzed by RT-PCR and ELISA showing the down-regulation of inflammatory cytokines released by fibroblasts, such Trasnforming Growth Factor (TGF)-β1, GM-CSF, IL-8, IL6, TNF, IL1β, and thymus as well as activation-regulated chemokines (TARC) (Solomon et al., 2005; Kim et al., 2019; Kim et al., 2020) or down-regulation of the inflammatory cytokine IL17 released by T cells (Bravo et al., 2016). In addition, the levels of PnD-expressed anti-inflammatory genes in these inflammatory systems, such as PGE2, TGFβ, and Vascular Endothelial Growth Factor (VEGF), can also be measured (Kim et al., 2019).
As described throughout this chapter, the anti-inflammatory potential of PnD is primarily assessed by determining self-secreted cytokines, as well as the production of pro- and anti-inflammatory cytokines by immune cells. ELISA and flow cytometry are the most widely used and best validated methods to measure cytokines and other inflammatory mediators. However, these methods are time-consuming, require a long sample preparation time, and do not allow the measurements of multiple cytokines at the same time in the same sample and in real-time. Recently, multiplex arrays (Luminex-based) have been developed from traditional ELISAs which allows the measurement of multiple cytokines in the same sample at the same time, and using only a small volume (Leng et al., 2008). Other commonly used immunoassays include Meso Scale Discovery (MSD), cytometric bead array (CBA), time-resolved fluorescence resonance energy transfer (TR-FRET), AlphaLISA, and FirePlex which have different sensitivities and multiplexing capabilities (Platchek et al., 2020). These technologies could represent more reliable and simpler strategies to assess the effect of PnD on immune cells.
2.2 PnD effects on inflammation and the vascular function
Among PnD, endothelial cells derived from the placenta (hP-EC) and umbilical cord, such as HUVEC represent a precious easy access ex vivo model of the human vasculature (Silini et al., 2020). HUVEC applications range from cardiovascular to metabolic as well as wound healing and angiogenesis-related diseases (Medina-Leyte et al., 2020). In addition, Gestational Diabetes (GD)-HUVEC exposed in vivo even transiently (during pregnancy) to hyperglycemia, exhibit some epigenetics modifications leading to a durable pro-inflammatory phenotype (Di Fulvio et al., 2014; Di Pietrantonio et al., 2021). HUVEC have been used to study the pro- and anti-atherogenic effect of several molecules in the early stages of atherosclerosis (Di Tomo et al., 2015; Di Pietro et al., 2020). Therefore, they represent a valuable in vitro model to assess the role of PnD in the above diseases through the following functional assays.
Due to a pro-oxidant state of Gestational Diabetes (GD)-HUVEC can be stimulated with an oxidative stress agent and compared to control HUVEC. O2- production and intracellular accumulation of reactive oxygen species (ROS) are measured to assess the antioxidant effect of PnD. While these methods allow to assess the general oxidative status, more integrative approaches evaluating potential antioxidant effects with higher sensitivity as well as the identification of permanent markers of oxidative stress are required (Pipino et al., 2020). Therefore, the levels of DNA/RNA damage, lipid peroxidation, and protein oxidation/nitration such as nitrotyrosine expression associated with impaired vascular nitric oxide (NO) secretion and availability may give a more reliable scenario regarding alteration of the oxidative balance (Di Fulvio et al., 2014; Alshabibi et al., 2018; Di Pietrantonio et al., 2021).
In endothelial dysfunction, the early stage of atherogenesis, there is a close interaction between oxidative stress and inflammation. Increased monocyte adhesion to the endothelium is among the mechanisms predisposing to endothelial dysfunction, the early predictor of plaque formation and atherosclerosis. The Monocyte-HUVEC Adhesion Assay can be performed in C- and GD-HUVEC in the basal state and after incubation with PnD before stimulation with an inflammatory stimulus such as low doses of TNF. Subsequently, cells from the monocytic cell line U937 are added to evaluate their adhesion to HUVEC monolayers (Di Tomo et al., 2015). Moreover, by qPCR and western blot/flow cytometry any inflammation-related gene and protein, such as Vascular Cell Adhesion Molecule 1 (VCAM-1) and Intercellular Adhesion Molecule 1 (ICAM-1), can be evaluated. Indeed, it is noteworthy that the level of these adhesion molecules increases during inflammation. More importantly, VCAM-1 and ICAM-1 membrane exposure, the main mechanism behind the interaction between monocytes and endothelial cells, can be analyzed by flow cytometry (Di Tomo et al., 2015).
Furthermore, it is accepted that impaired NO synthesis and/or its availability in HUVEC may result in endothelial dysfunction (Pandolfi and De Filippis, 2007). To assess NO bioavailability, the intracellular cGMP level, a biological target of NO activity, is mainly evaluated by using a commercial enzyme immunoassay (EIA) kit (Di Pietro et al., 2020). It has been demonstrated that HUVEC chronically exposed to high glucose and inflammation, as well as treated with the proinflammatory stimulus TNF-α, display decreased levels of cGMP (Ucci et al., 2019) Therefore, this assay is useful to assess the role of PnD in changes of NO bioavailability, which is involved in the modulation of the Nuclear Factor kappa-light-chain-enhancer of activated B cells (NF-κB) nuclear translocation, relevant to further evaluate the potential ability of PnD in inhibiting the inflammatory pathway (Ucci et al., 2019). However, accurate NO detection and quantification are critical to understanding health and disease. Consequently, more than one of the aforementioned assays should be performed for a clear comprehension of anti-inflammatory PnD effects.
Although these assays are widely used for reproducible determinations, the results obtained may be partially affected by the passage number and donor variability of endothelial cells. To overcome these issues, experiments may be performed on multiple endothelial cell strains or immortalized endothelial cells, showing a more uniform response.
Moreover, in some inflammatory diseases, the release of several pro-inflammatory chemokines may inhibit neovascularization. The capability of PnD to regulate the chemokine environment by inhibiting the pro-inflammatory chemokines and/or by increasing the pro-angiogenic ones, may enhance new vessel formation. The most conventional way of assessing PnD’ capacity in decreasing inflammation while improving endothelial cell network-like structures is the Matrigel tube formation assay (Ma et al., 2021; Nensat et al., 2021). This assay, together with the evaluation of cell migration through a scratch assay (Bernabe-Garcia et al., 2017), can be performed to assess the potential therapeutic efficacy of PnD in angiogenesis-related disease, such as diabetic foot ulcers (Castellanos et al., 2017). Besides the rapid and easy well-established method and the comparably easy cell culture and measurement, this assay is only partially representative of real cell environments. Although many laboratories commonly use this method to obtain first evidence of angiogenic and antiangiogenic agents, some limitations may occur such as difficult in vessel quantification through a specific plugin of ImageJ software as well as standardization due to Matrigel lot-to-lot variability (Nowak-Sliwinska et al., 2018). The latter may be prevented by selecting a specific lot with preferred protein and endotoxin concentrations.
Overall, all the HUVEC assays mentioned here were recently published in a study performed to assess the anti-inflammatory and pro-angiogenic role of hAM in GD-HUVEC. The results obtained strongly elucidate the mechanisms through which hAM can affect inflammation, migration, and angiogenesis thus providing additional validation for ongoing clinical trials in diabetic foot ulcer (Pipino et al., 2022).
In addition, to overcome limitations of 2D cell culture assays, HUVEC can be used in vitro to create 3D spheroid structures for functional screening purposes (Kocherova et al., 2019) or blood vessel tissue engineering (Moby et al., 2007; Paternotte et al., 2013). Finally, endothelial cells and the anti-inflammatory influence of PnD can also be analyzed even more physiologically under shear stress conditions in dedicated flow chambers with a computer-guided pump system and video microscopy (Zantl and Horn, 2011; Munir et al., 2015). However, all these methods do not acknowledge the multicellularity in the perivascular microenvironment. An in vitro solution to this problem would be vascularized spheroids or organoids which are currently under investigation.
2.3 PnD effects on cutaneous wound healing
Substantial evidence from several clinical trials shows how the application of hAM or other PnD on wounds and ulcers of diverse etiology has proven to be of benefit (Colocho et al., 1974; Singh et al., 2004; Hasegawa et al., 2007; Mermet et al., 2007; Silini et al., 2015). Additionally, a combination of PnD or even extra-cellular vesicles from PnD have been used for chronic wounds (Bakhtyar et al., 2018; Hashemi et al., 2019). All that evidence makes hAM and derived products the most researched so far in terms of wound healing treatment. Nevertheless, to fully understand the capabilities of hAM and other PnD to promote wound healing, several in vitro systems have been developed. Most chronic wounds show decreased proliferation and migration, mainly affecting keratinocytes, but also fibroblasts and endothelial cells. In consequence, those experimental settings mainly focus on evaluating the pro-proliferative, pro-migratory and pro-angiogenic effects that hAM or other PnD may have.
In addition, in vivo wound healing assays using animal models constitute an integrative benchmark in which PnD′ effects on inflammation and the immune component, such as angiogenesis and dermal as well as epidermal cell migration and proliferation, have to cooperate to show real functional effectiveness. The application of human PnD in animal studies of cutaneous wound healing was extensively reviewed by Pichlsberger et al. (Pichlsberger et al., 2021). In approximately 50% of the included studies (Pichlsberger et al., 2021) the PnD were functionally tested in vitro before they were applied to animal wounds. Herein we focus on the in vitro functional assays used in these studies. The outcome of these in vitro assays, the types and combinations of the PnD applied as well as the references are outlined in detail in Tables 1–5. As naming and abbreviations of the PnD types in the reviewed studies varied due to the authors’ discretion, we harmonized terms according to the recently published consensus nomenclature for PnD to improve the comparability of data (Silini et al., 2020). Among the included studies, the majority of the in vitro tested PnD were cells (38%) mainly MSC isolated from the umbilical cord, amnion, amniotic fluid, placenta, but also cells derived from the amniotic fluid, and the amniotic epithelium. Further functional tests were performed on cellular secretomes (29%), followed by cell-derived small extracellular vesicles (sEVs, 15%) derived from MSC of the umbilical cord or decidua, tissue extracts (13%) and tissue membranes (amnion or amnion/chorion, 6%) (Figure 1A). We did not discriminate between the different subtypes of extracellular vesicles (EV) including exosomes etc., but chose the term sEVs instead, according to the recommendations of the International Society for Extracellular Vesicles to use an operational term for EV subtypes unless their endosomal origin was proven (Van Deun et al., 2017; Thery et al., 2018). Overall, these studies used twenty different functional assays to evaluate PnD in vitro (Figure 1B). The most frequently performed functional assays were: 1) cell proliferation assay, 2) scratch wound assay, 3) chemotaxis assay and 4) angiogenesis assays. These assays are considered the gold standard for the evaluation of wound healing in vitro since they analyze important processes that occur during wound healing, such as proliferation and migration of the cells at the wound edges and the re-epithelialization of the wound surface.
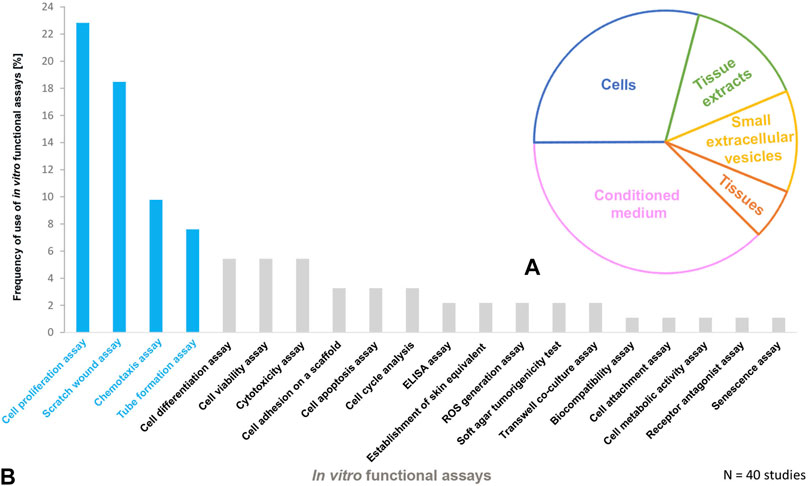
FIGURE 1. Perinatal derivatives and in vitro functional assays used for cutaneous wound healing in animals (A) The schematic presentation of PnD functionally tested in vitro and (B) frequency of use of in vitro functional assays in animal studies of cutaneous wound healing. The most frequently used functional assays are highlighted in blue.
From the pathological point of view, an important limiting factor for wound healing could be the development of an altered keratinocyte behavior (Raja et al., 2007). In that sense, many authors have measured the ability of amnion or other PnD in promoting keratinocyte migration in vitro. Next to the well-known Boyden chamber migration assay (Mcquilling et al., 2019), the wound healing scratch assay is frequently used. By design, based on measuring the gap generated by mechanically tearing a cultured cell monolayer, the scratch assay allows for easy monitoring and quantification of cell migration and wound closure (Liarte et al., 2018). The unique advantage of this assay is the fact that cells at the wound edge can be studied microscopically at any time, allowing for the characterization of distinct morphology and topological changes in living cultures, along with the precise detection of the expression of key factors involved in wound healing on fixed cultures (Bernabe-Garcia et al., 2017). Of note, the wound healing scratch assay can be applied on almost any cell line growing in monolayers. For instance, in the specific case of skin wounds, most of the preclinical experience with amnion and other PnD is based on the effects of well stablished human keratinocyte cell lines, such as the spontaneously transformed aneuploid immortal human cell line HaCaT (Boukamp et al., 1988). However, several reports also apply the scratch method onto human fibroblasts (Bakhtyar et al., 2018; Rahman et al., 2019). In the case of keratinocytes, HaCaT offers unparalleled characteristics by being phenotypically similar to primary keratinocytes, while still allowing routine culture procedures (Boukamp et al., 1988). Numerous papers show that HaCaT keratinocytes had not only allowed to certify the benefits of amnion on migration (Alcaraz et al., 2015; Kitala et al., 2019; Rahman et al., 2019) but also to understand how amnion treatment effects appear to be restricted to the edge of the artificial wound in vitro (Ruiz-Canada et al., 2018). These results support the notion that migration effects of amnion are not systemic, but local. This is in line with clinical observations that the effects of amnion treatment of human patients’ wounds were limited to the wound edge (Insausti et al., 2010). Moreover, the HaCaT scratch assay in combination with immunological methods allows for the precise detection of key proteins participating in the migration machinery, such as Paxilline H, demonstrating that among its effects, amnion treatment triggers focal adhesion molecule rearrangement in cells at the very edge of the wound and is thus promoting local cell migration (Bernabe-Garcia et al., 2017). Altogether, the possibilities provided by the wound healing scratch assay offer uncontested capacity to obtain high quality data in a comprehensive manner, opening opportunity for precise correlations between what is macroscopically observed in the chronic wounds and the behavior of cells observed in vitro, as shown for the HaCaT cells, (Ruiz-Canada et al., 2021).
A proper development of the underlying dermal tissue is a prerequisite for keratinocyte proliferation as an important limiting factor for correct healing and wound closure. Therefore, fibroblast and endothelial cell proliferation also needs to be assessed. Using different methodologies, from image analysis to comparative cell counting, including cell cycle analysis and cell suspension absorbance determination, numerous papers evaluate the effects of amnion treatment and coincide in finding positive effects on keratinocyte and fibroblast proliferation (Alcaraz et al., 2015; Murphy et al., 2017; Kitala et al., 2019; Rahman et al., 2019).
In contrast, comparably little is known about the effects of amnion or other PnD on endothelial cell proliferation. However, studies assessing this component mostly apply methods based on the analysis of in vitro tube formation and branching by endothelial cells in culture (Hu et al., 2018; Bullard et al., 2019). Tube formation assay, an in vitro angiogenesis assay, is often used in wound healing experiment designs since the formation of new vessels significantly contributes to tissue recovery. The 3D-tube formation assay on gelled basement membrane extract is a powerful in vitro technique for evaluating angiogenesis. This assay involves endothelial cell adhesion, migration, protease activity, and tube formation (Arnaoutova and Kleinman, 2010). Also, 2D co-culture models are meaningful assays to evaluate the functionality of the respective PnD type. For example, endothelial cells showed a significantly increased formation of vessel-like structures in direct co-culture with MSC derived from placental blood vessels as compared to human amniotic membrane mesenchymal stromal cells (hAMSC) (Konig et al., 2015). The angiogenic properties of PnD were also evaluated by the chorioallantoic membrane (CAM) assay (Edwards et al., 2014; Rameshbabu et al., 2018; Kaushik and Das, 2019). The CAM assay is an intermediate step between in vitro and the in vivo models. It is minimally invasive to the chick embryo, and it is therefore a potential approach to refinement of animal experimentation (Moreno-Jimenez et al., 2016).
In the context of wound healing, the cell differentiation assay should also be a reasonable method of choice. However, it was not well represented in our dataset, with only 5% of studies investigating the ability of PnD to promote skin cell differentiation in vitro. The same is true for the cytotoxicity and viability assays, which would also be useful before the application of the PnD in vivo in preclinical and clinical studies.
In general, the PnD treatment showed favorable effects in terms of cutaneous wound healing since it promoted cell proliferation, migration, and differentiation of cells in vitro and showed no cytotoxic effects (Table 1, Table 2, Table 3, Table 4, Table 5). In this regard, the functional assays demonstrated considerable potency, since the results from the preclinical studies also show a promoting effect of PnD on cutaneous wound healing (Pichlsberger et al., 2021). Nevertheless, evaluating the potency of the in vitro functional assays turned out to be delicate as the PnD tested in vitro were often not the same as those applied in vivo. For example, in the same study the PnD-derived CM was functionally tested in vitro, but whole perinatal cells were applied to the animal wound (Kim et al., 2012a; Edwards et al., 2014; Jin et al., 2016). For the development of more effective PnD therapies, this will need to be considered in future studies.
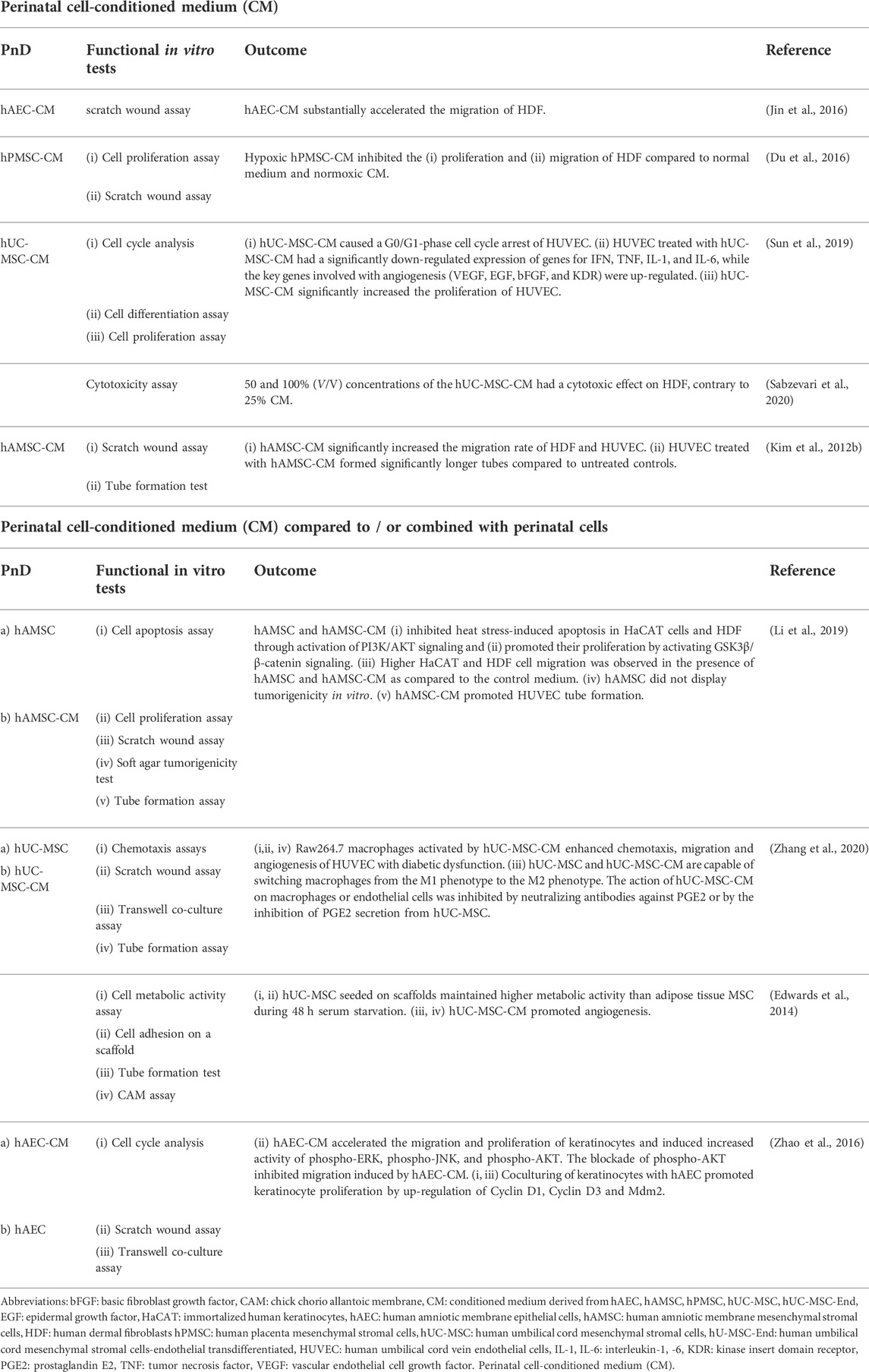
TABLE 2. Functional tests on perinatal cell-conditioned medium (CM) alone or compared to/or combined with perinatal cells.
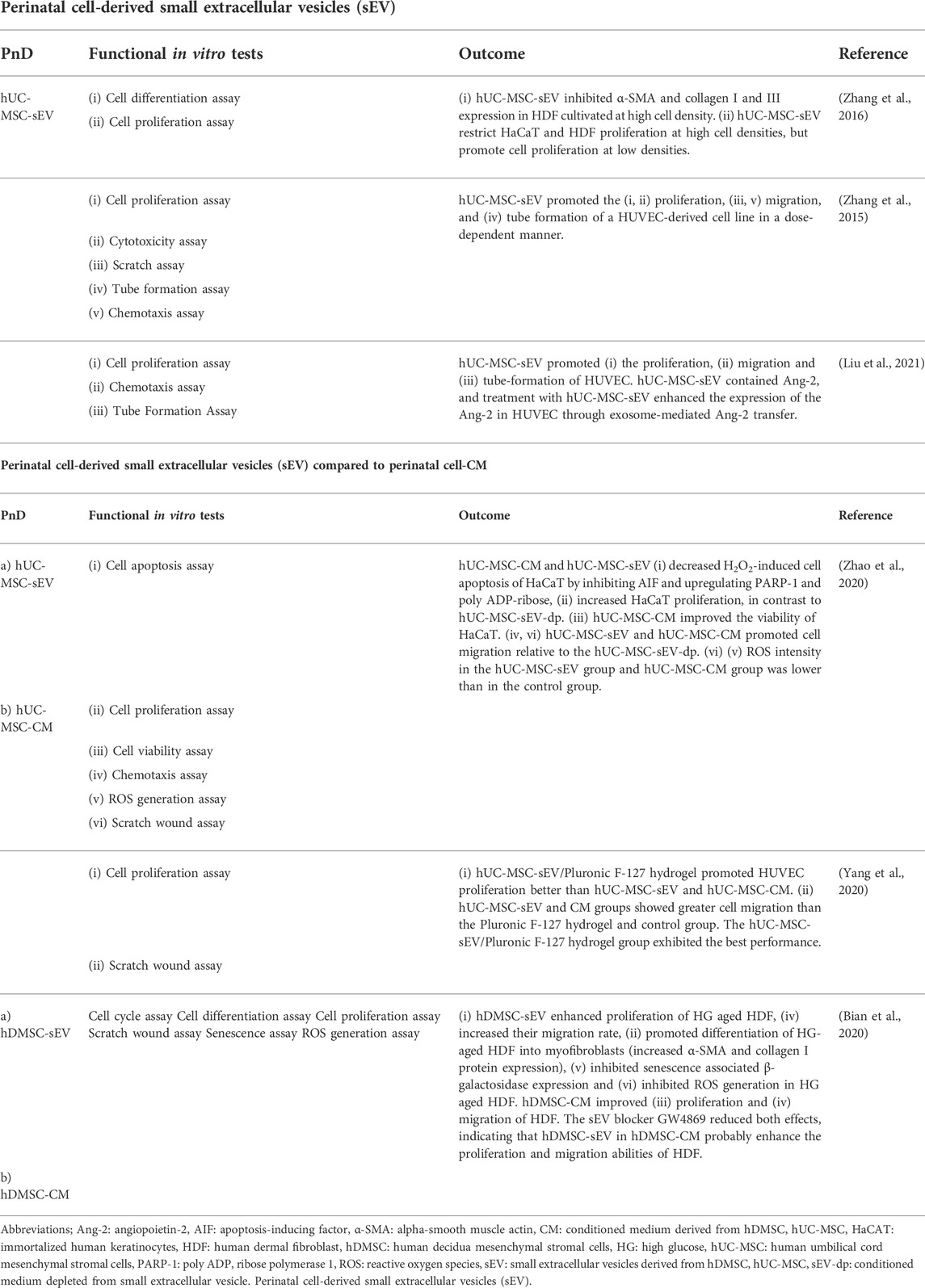
TABLE 3. Functional tests on Perinatal cell-derived small extracellular vesicles (sEV) alone or compared to perinatal cell-conditioned medium (CM).
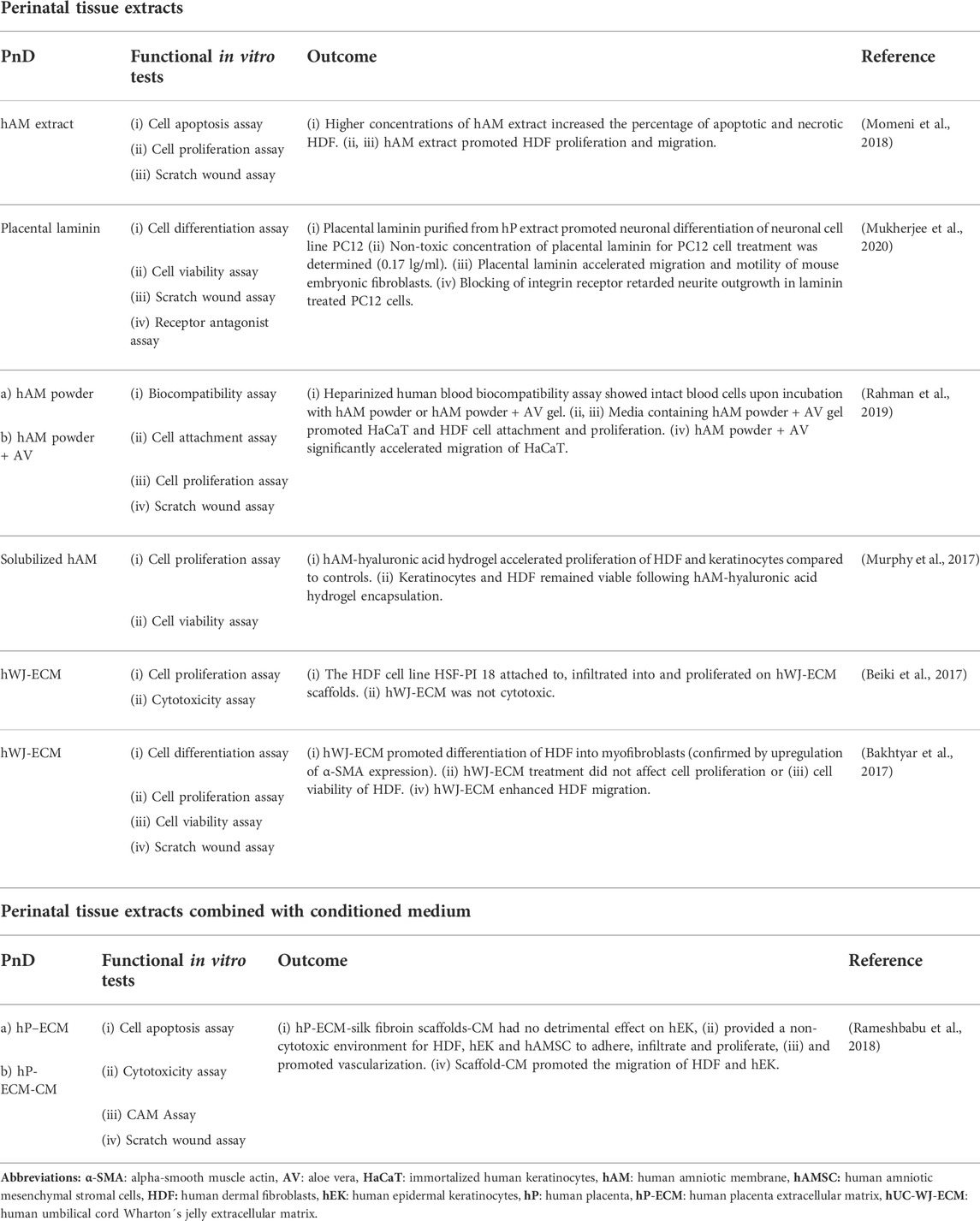
TABLE 5. Functional tests on perinatal tissue extracts alone or combined with conditioned medium (CM).
2.4 Oral wound healing versus cutaneous wound healing
Special PnD, i.e. hAM, chorion and human Amnio-Chorionic Membrane (hACM) have been widely used for wound healing in oral reconstruction (Fenelon et al., 2018; Gulameabasse et al., 2020). For instance, hAM as a scaffold enhanced re-epithelialization of the oral cavity and reduced the contracture effects in moderate-sized defects (Lawson, 1985). The hAM has also been proven as useful biodegradable graft material for clinical vestibuloplasty. One week after the surgical intervention, epithelium started to migrate over the graft area from the margins, and the underlying connective tissue showed the formation of granulation tissue. The hAM completely degenerated after 3 weeks. Three months post intervention, epithelial tissue was restored and completely covered the graft area (Samandari et al., 2004). Measurement of blood flow to grafts used in vestibuloplasty revealed an increased blood flow to hAM grafts, whereas palatal grafts had a reduced blood flow during the same time period. Thus, angiogenesis was induced by hAM within 10–15 days, and the blood flow returned to normal by 30 days after surgery (Guler et al., 1997). These favorable effects of the hAM may be due to growth factors present in the amnion, such as basic Fibroblast Growth Factor (bFGF), Epidermal Growth Factor (EGF), TGFβ and IL-1 (Dadkhah Tehrani et al., 2020).
A corresponding animal study revealed that hAM transplanted on rabbit’s gingival wound accelerated the formation of granulation tissue at day 10 by significantly increasing the number of both fibroblasts and blood vessels. Thus, hAM induced rapid epithelialization and both granulation tissue and collagen formation, and suppressed inflammation i. e., the migration of polymorphonuclear cells at the wounded gingival site (Rinastiti et al., 2006).
In oral wound healing, the anti-adhesive properties of hAM in contact with healthy tissue are useful to prevent tissue adhesion in surgical procedures. The hAM transplant may function as an anatomical barrier towards fibrous tissue proliferation (Samandari et al., 2004; Fenelon et al., 2018), but further models are needed to improve understanding of this mechanism of action.
An interesting aspect in the context of wound healing is that oral wounds mainly heal without scarring. The keratinized epidermis of the skin shares some similarities with the oral mucosa, such as the stratified epithelium. In addition, also certain regions of the oral mucosa (gingiva, palate) show signs of keratinization, while the nonkeratinized floor of the mouth and buccal regions do not produce a stratum corneum (Wertz, 2021).
The main difference between skin and the keratinized regions of the oral mucosa is the relatively dry cutaneous surface, which is covered by the secretion products of sweat glands. Hair follicles can be found in most cutaneous regions except the palms and foot soles, and the apical surface is additionally covered by sebum. In contrast, the oral mucosa is constantly moist and well protected by the continuous secretion of mucous glands. The humid milieu may be one of the reasons that the healing of oral mucosal wounds is faster with minimal to no scar formation. There is a smaller inflammatory response with less neutrophils, macrophages, and T-cell infiltration, and the proliferation and migration rate of keratinocytes from oral mucosa is much more rapid as compared to skin keratinocytes (Turabelidze et al., 2014).
During the healing period of adult skin wounds rapid angiogenesis occurs leading to many more capillaries than in normal tissue. Compared to that, healing wounds of oral mucosa have a reduced angiogenic network but are composed of more mature vessels providing better oxygenation. As inflammatory cells produce a variety of proangiogenic factors, it can be assumed that the selective reduction of inflammation and angiogenesis may help to prevent excessive scarring (Dipietro, 2016).
Covering wounds with various types of dressings may help to keep the wound area in a humid milieu that allows controlled regeneration processes without excessive scarring. Most current in vitro assays are performed under fluid conditions, where the cells are exposed to culture medium. Functional assays on cell cultures using air-liquid-interface (ALI) would be helpful to mimic physiologic conditions even more closely.
3 Summary and conclusion
In this review, we addressed studies in which PnD were functionally tested in vitro before being applied to pre-clinical or clinical care with respect to inflammation, angiogenesis and wound healing (Figure 2). We have focussed on the types and combinations of PnD and the functional assays used as well as on the outcomes of the in vitro assays.
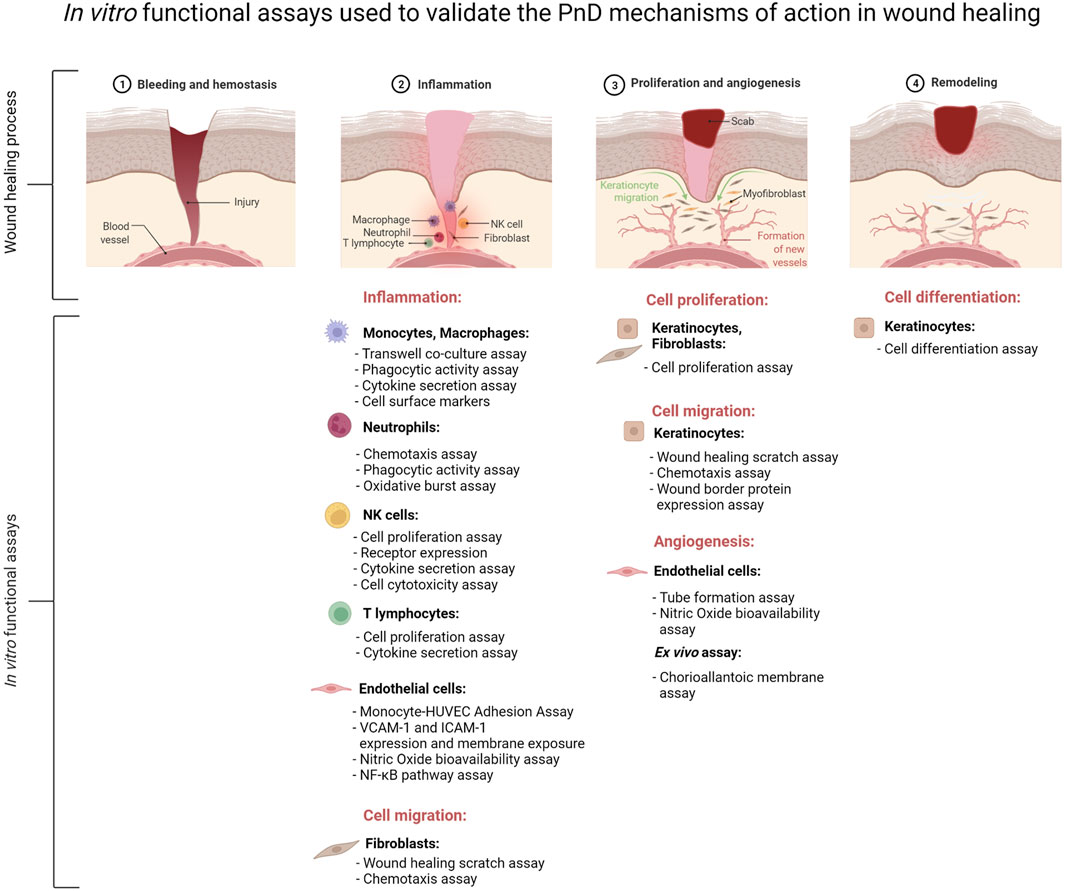
FIGURE 2. Schematic presentation of the major in vitro functional assays performed to validate the mechanisms of action of perinatal derivatives (PnD) in the context of inflammation, angiogenesis and remodeling during the complex process of wound healing. The figure was created using Biorender.com.
Inflammation is a complex physiological mechanism involving different types of immune cells, protein mediators, and metabolites, making it difficult to study the role of PnD in inflammation in vitro. Our analysis indicates that most of the available assays to determine the ability of PnD to control inflammation study the interaction of different types of PnD with a single type of immune cells. These in vitro studies are mainly based on transwell co-cultures of PnD with immune cells, or treatment of immune cells with PnD CM, allowing to differentiate between cell-to-cell contact or paracrine signaling, respectively. The following functional assays are the most frequently used for evaluating immune cell activity: cell proliferation assay, cytokine secretion assay, surface markers expression indicative of acquisition of an anti-inflammatory phenotype, cytotoxicity and phagocytosis assay. Oxidative burst capacity is another assay that has been used to validate the anti-inflammatory role of PnD over neutrophils. All specialized phagocytes i.e., neutrophils and macrophages, have the capacity to generate the respiratory burst (Dahlgren et al., 2007), and this assay could be included as an in vitro functional test of the anti-inflammatory capacity of PnD over these other phagocytic cells. Moreover, the role of PnD on vascular inflammation can be assessed by means of several functional assays using HUVEC as a valuable in vitro model of human vasculature (Pipino et al., 2022).
Among the different functional assays available, the following four assay types are considered as the gold standard for assessing wound healing in vitro: the cell proliferation, the scratch wound, the chemotaxis, and the angiogenesis assay. Of note, in vitro models with characteristics closer to those cells in chronic wounds are needed to attain all the benefits of the application of PnD to non-healing ulcers (Liarte et al., 2020b). Indeed, while chronic wound fluids are known to be rich in proinflammatory cytokines, such as the anti-proliferative TGFß, it has been shown that AM can inhibit TGFß signaling, restoring keratinocyte proliferation and migration (Ruiz-Canada et al., 2018; Ruiz-Canada et al., 2021). Recently, HaCaT cells had been shown to represent a phenotype better resembling the state of keratinocytes in chronic wounds (Liarte et al., 2020a).
Inflammation, angiogenesis, and wound healing are thoroughly regulated processes that play an important role in tissue regeneration. If the balance of these regulatory mechanisms is disturbed, excessive inflammatory reactions, misguided angiogenesis, delayed wound healing or excessive scarring can occur. A promising therapeutic option is treatment with PnD. To understand the mode of action of PnD, various functional assays are carried out. Furthermore, there is a certain risk that the PnD sample may be of varying quality due to donor variability. Thus, functional assays are also important for checking the quality of the PnD compound prior to preclinical and clinical use.
As the gap between cell culture experiments and a complete organism is difficult to bridge, animal models are needed to resolve questions about toxicology or pharmacology. We strongly suggest that functional in vitro testing of PnD is routinely performed before their application to animal models and patients. This helps to minimize animal numbers in animal experimentation in line with the Replacement, Reduction, and Refinement (3R) principles for more ethical use of animals in research (Strech and Dirnagl, 2019) and to ensure the safety and efficacy of the PnD applied to patients.
The present review is in line with the aims of the COST SPRINT Action (CA17116) (Silini et al., 2020) and will contribute to the establishment of guidelines for methods applied to cells and tissues to enable scientifically sound and reproducible research data to promote PnD into clinics.
Author contributions
AF, CP, UJ, SL, FG, MEK, FN, AP, LT and IL-O wrote specific sections of the manuscript. UJ created the figures. IL-O and GE coordinated the work and compiled the manuscript. BG, MP, AP, OP and GE designed the concept of the manuscript. All authors contributed to revising and editing of the manuscript. All authors approved the manuscript in its present form.
Funding
This work contributes to the COST (Cooperation in Science and Technology) Action CA17116 International Network for Translating Research on Perinatal Derivatives into Therapeutic Approaches (SPRINT), funded by the European Commission. This work was also supported by the Instituto de Salud Carlos III (ISCIII), Ministry of Economy, Industry, and Competitiveness, co-funded by the European Regional Development Fund, grant number PI18/01278 (to AIF), by the Slovenian Research Agency (J7-2,594, to MEK). And by the PON-MISE Sustainable Growth Funding-DD 27/09/2018 (grant 557 number: 21, ex 230, to AP) as well as by MIUR - PON AIM “Attraction and International Mobility” Linea 2 (Attrazione dei ricercatori), profilo 2.1 and Ministero della Sanità Programma di Ricerca Finalizzata 2019 (GR-2019-12369702, to CP).
Acknowledgments
This article contributes to the COST Action CA17116 “International Network for Translating Research on Perinatal Derivatives into Therapeutic Approaches (SPRINT)”, supported by COST (European Cooperation in Science and Technology).
Conflict of interest
The authors declare that the research was conducted in the absence of any commercial or financial relationships that could be construed as a potential conflict of interest.
Publisher’s note
All claims expressed in this article are solely those of the authors and do not necessarily represent those of their affiliated organizations, or those of the publisher, the editors and the reviewers. Any product that may be evaluated in this article, or claim that may be made by its manufacturer, is not guaranteed or endorsed by the publisher.
References
Abomaray, F. M., Al Jumah, M. A., Kalionis, B., AlAskar, A. S., Al Harthy, S., Jawdat, D., et al. (2015). Human chorionic villous mesenchymal stem cells modify the functions of human dendritic cells, and induce an anti-inflammatory phenotype in CD1+ dendritic cells. Stem Cell Rev Rep 11, 423–441. doi:10.1007/s12015-014-9562-8
Abumaree, M. H., Abomaray, F. M., Alshabibi, M. A., AlAskar, A. S., and Kalionis, B. (2017). Immunomodulatory properties of human placental mesenchymal stem/stromal cells. Placenta 59, 87–95. doi:10.1016/j.placenta.2017.04.003
Abumaree, M. H., Al Jumah, M. A., Kalionis, B., Jawdat, D., Al Khaldi, A., Abomaray, F. M., et al. (2013). Human placental mesenchymal stem cells (pMSCs) play a role as immune suppressive cells by shifting macrophage differentiation from inflammatory M1 to anti-inflammatory M2 macrophages. Stem Cell Rev Rep 9, 620–641. doi:10.1007/s12015-013-9455-2
Abumaree, M. H., Alshehri, N. A., Almotery, A., Al Subayyil, A. M., Bahattab, E., Abomaray, F. M., et al. (2019). Preconditioning human natural killer cells with chorionic villous mesenchymal stem cells stimulates their expression of inflammatory and anti-tumor molecules. Stem Cell Res. Ther. 10, 50. doi:10.1186/s13287-019-1153-9
Abumaree, M. H., Bahattab, E., Alsadoun, A., Al Dosaimani, A., Abomaray, F. M., Khatlani, T., et al. (2018). Characterization of the interaction between human decidua parietalis mesenchymal stem/stromal cells and natural killer cells. Stem Cell Res. Ther. 9, 102. doi:10.1186/s13287-018-0844-y
Alcaraz, A., Mrowiec, A., Insausti, C. L., Bernabé-García, A., García-Vizcaíno, E. M., López-Martínez, M. C., et al. (2015). Amniotic membrane modifies the genetic program induced by TGFss, stimulating keratinocyte proliferation and migration in chronic wounds. PLoS One 10, e0135324. doi:10.1371/journal.pone.0135324
Alipour, R., Motedayyen, H., Sereshki, N., Rafiee, M., Alsahebfosul, F., and Pourazar, A. (2020). Human amniotic epithelial cells affect the functions of neutrophils. Ijsc 13, 212–220. doi:10.15283/ijsc19155
Alshabibi, M. A., Khatlani, T., Abomaray, F. M., AlAskar, A. S., Kalionis, B., Messaoudi, S. A., et al. (2018). Human decidua basalis mesenchymal stem/stromal cells protect endothelial cell functions from oxidative stress induced by hydrogen peroxide and monocytes. Stem Cell Res. Ther. 9, 275. doi:10.1186/s13287-018-1021-z
Arnaoutova, I., and Kleinman, H. K. (2010). In vitro angiogenesis: Endothelial cell tube formation on gelled basement membrane extract. Nat. Protoc. 5, 628–635. doi:10.1038/nprot.2010.6
Babatunde, K. A., Wang, X., Hopke, A., Lannes, N., Mantel, P. Y., and Irimia, D. (2021). Chemotaxis and swarming in differentiated HL-60 neutrophil-like cells. Sci. Rep. 11, 778. doi:10.1038/s41598-020-78854-6
Bakhtyar, N., Jeschke, M. G., Herer, E., Sheikholeslam, M., and Amini-Nik, S. (2018). Exosomes from acellular Wharton's jelly of the human umbilical cord promotes skin wound healing. Stem Cell Res. Ther. 9, 193. doi:10.1186/s13287-018-0921-2
Bakhtyar, N., Jeschke, M. G., Mainville, L., Herer, E., and Amini-Nik, S. (2017). Acellular gelatinous material of human umbilical cord enhances wound healing: A candidate remedy for deficient wound healing. Front. Physiol. 8, 200. doi:10.3389/fphys.2017.00200
Beiki, B., Zeynali, B., and Seyedjafari, E. (2017). Fabrication of a three dimensional spongy scaffold using human Wharton's jelly derived extra cellular matrix for wound healing. Mater. Sci. Eng. C 78, 627–638. doi:10.1016/j.msec.2017.04.074
Bernabé-García, A., Liarte, S., Moraleda, J. M., Castellanos, G., and Nicolás, F. J. (2017). Amniotic membrane promotes focal adhesion remodeling to stimulate cell migration. Sci. Rep. 7, 15262. doi:10.1038/s41598-017-15509-z
Bian, X., Li, B., Yang, J., Ma, K., Sun, M., Zhang, C., et al. (2020). Regenerative and protective effects of dMSC-sEVs on high-glucose-induced senescent fibroblasts by suppressing RAGE pathway and activating Smad pathway. Stem Cell Res. Ther. 11, 166. doi:10.1186/s13287-020-01681-z
Boissel, L., Tuncer, H. H., Betancur, M., Wolfberg, A., and Klingemann, H. (2008). Umbilical cord mesenchymal stem cells increase expansion of cord blood natural killer cells. Biol. Blood Marrow Transplant. 14, 1031–1038. doi:10.1016/j.bbmt.2008.06.016
Boukamp, P., Petrussevska, R. T., Breitkreutz, D., Hornung, J., Markham, A., and Fusenig, N. E. (1988). Normal keratinization in a spontaneously immortalized aneuploid human keratinocyte cell line. J. Cell Biol. 106, 761–771. doi:10.1083/jcb.106.3.761
Brandstadter, J. D., and Yang, Y. (2011). Natural killer cell responses to viral infection. J. Innate Immun. 3, 274–279. doi:10.1159/000324176
Bravo, B., Gallego, M. I., Flores, A. I., Bornstein, R., Puente-Bedia, A., Hernández, J., et al. (2016). Restrained Th17 response and myeloid cell infiltration into the central nervous system by human decidua-derived mesenchymal stem cells during experimental autoimmune encephalomyelitis. Stem Cell Res. Ther. 7, 43. doi:10.1186/s13287-016-0304-5
Bullard, J. D., Lei, J., Lim, J. J., Massee, M., Fallon, A. M., and Koob, T. J. (2019). Evaluation of dehydrated human umbilical cord biological properties for wound care and soft tissue healing. J. Biomed. Mat. Res. 107, 1035–1046. doi:10.1002/jbm.b.34196
Cargnoni, A., Papait, A., Masserdotti, A., Pasotti, A., Stefani, F. R., Silini, A. R., et al. (2021). Extracellular vesicles from perinatal cells for anti-inflammatory therapy. Front. Bioeng. Biotechnol. 9, 637737. doi:10.3389/fbioe.2021.637737
Castellanos, G., Bernabé-García, A., Moraleda, J. M., and Nicolás, F. J. (2017). Amniotic membrane application for the healing of chronic wounds and ulcers. Placenta 59, 146–153. doi:10.1016/j.placenta.2017.04.005
Chambers, D. C., Enever, D., Ilic, N., Sparks, L., Whitelaw, K., Ayres, J., et al. (2014). A phase 1b study of placenta-derived mesenchymal stromal cells in patients with idiopathic pulmonary fibrosis. Respirology 19, 1013–1018. doi:10.1111/resp.12343
Chatterjee, D., Marquardt, N., Tufa, D. M., Beauclair, G., Low, H. Z., Hatlapatka, T., et al. (2014). Role of gamma-secretase in human umbilical-cord derived mesenchymal stem cell mediated suppression of NK cell cytotoxicity. Cell Commun. Signal 12, 63. doi:10.1186/s12964-014-0063-9
Colocho, G., Graham, W. P., 3R. D., Greene, A. E., Matheson, D. W., and Lynch, D. (1974). Human amniotic membrane as a physiologic wound dressing. Arch. Surg. 109, 370–373. doi:10.1001/archsurg.1974.01360030022006
Croxatto, D., Vacca, P., Canegallo, F., Conte, R., Venturini, P. L., Moretta, L., et al. (2014). Stromal cells from human decidua exert a strong inhibitory effect on NK cell function and dendritic cell differentiation. PLoS One 9, e89006. doi:10.1371/journal.pone.0089006
Dadkhah Tehrani, F., Firouzeh, A., Shabani, I., and Shabani, A. (2020). A review on modifications of amniotic membrane for biomedical applications. Front. Bioeng. Biotechnol. 8, 606982. doi:10.3389/fbioe.2020.606982
Dahlgren, C., Karlsson, A., and Bylund, J. (2007). Measurement of respiratory burst products generated by professional phagocytes. Methods Mol. Biol. 412, 349–363. doi:10.1007/978-1-59745-467-4_23
Dash, B. C., Xu, Z., Lin, L., Koo, A., Ndon, S., Berthiaume, F., et al. (2018). Stem Cells and Engineered Scaffolds for Regenerative Wound Healing. Basel): Bioengineering. 5.
De La Torre, P., Pérez-Lorenzo, , and Flores, A. I. (2018). “Human placenta-derived mesenchymal stromal cells: A review from basic research to clinical applications,” in Stromal cells - structure, function, and therapeutic implications. Editor M. T. VALARMATHI (London: IntechOpen Limited).
Demidova-Rice, T. N., Durham, J. T., and Herman, I. M. (2012). Wound healing angiogenesis: Innovations and challenges in acute and chronic wound healing. Adv. Wound Care 1, 17–22. doi:10.1089/wound.2011.0308
Deng, Q., Huang, S., Wen, J., Jiao, Y., Su, X., Shi, G., et al. (2020). PF-127 hydrogel plus sodium ascorbyl phosphate improves Wharton's jelly mesenchymal stem cell-mediated skin wound healing in mice. Stem Cell Res. Ther. 11, 143. doi:10.1186/s13287-020-01638-2
Di Fulvio, P., Pandolfi, A., Formoso, G., Di Silvestre, S., Di Tomo, P., Giardinelli, A., et al. (2014). Features of endothelial dysfunction in umbilical cord vessels of women with gestational diabetes. Nutr. Metabolism Cardiovasc. Dis. 24, 1337–1345. doi:10.1016/j.numecd.2014.06.005
Di Pietrantonio, N., Palmerini, C., Pipino, C., Baldassarre, M. P. A., Bologna, G., Mohn, A., et al. (2021). Plasma from obese children increases monocyte-endothelial adhesion and affects intracellular insulin signaling in cultured endothelial cells: Potential role of mTORC1-S6K1. Biochimica Biophysica Acta (BBA) - Mol. Basis Dis. 1867, 166076. doi:10.1016/j.bbadis.2021.166076
Di Pietro, N., Baldassarre, M. P. A., Cichelli, A., Pandolfi, A., Formoso, G., and Pipino, C. (2020). Role of polyphenols and carotenoids in endothelial dysfunction: An overview from classic to innovative biomarkers. Oxid. Med. Cell Longev. 2020, 6381380. doi:10.1155/2020/6381380
Di Tomo, P., Di Silvestre, S., Cordone, V. G., Giardinelli, A., Faricelli, B., Pipino, C., et al. (2015). Centella asiatica and lipoic acid, or a combination thereof, inhibit monocyte adhesion to endothelial cells from umbilical cords of gestational diabetic women. Nutr. Metabolism Cardiovasc. Dis. 25, 659–666. doi:10.1016/j.numecd.2015.04.002
Diegelmann, R. F., and Evans, M. C. (2004). Wound healing: An overview of acute, fibrotic and delayed healing. Front. Biosci. 9, 283–289. doi:10.2741/1184
DiPietro, L. A. (2016). Angiogenesis and wound repair: When enough is enough. J. Leukoc. Biol. 100, 979–984. doi:10.1189/jlb.4mr0316-102r
Du, L., Lv, R., Yang, X., Cheng, S., Ma, T., and Xu, J. (2016). Hypoxic conditioned medium of placenta-derived mesenchymal stem cells protects against scar formation. Life Sci. 149, 51–57. doi:10.1016/j.lfs.2016.02.050
Edinger, J., Karasiewicz, K., He, S., Ye, Q., and Hariri, R. (2018). “Placenta-derived mesenchymal stromal cells: Modulation of immunity and inflammation,” in Placenta. Editor R. G. AHMED (London: IntechOpen Limited). doi:10.5772/intechopen.79728
Edwards, S. S., Zavala, G., Prieto, C. P., Elliott, M., Martínez, S., Egaña, J. T., et al. (2014). Functional analysis reveals angiogenic potential of human mesenchymal stem cells from Wharton's jelly in dermal regeneration. Angiogenesis 17, 851–866. doi:10.1007/s10456-014-9432-7
Erkers, T., Nava, S., Yosef, J., Ringdén, O., and Kaipe, H. (2013). Decidual stromal cells promote regulatory T cells and suppress alloreactivity in a cell contact-dependent manner. Stem Cells Dev. 22, 2596–2605. doi:10.1089/scd.2013.0079
Fénelon, M., Catros, S., and Fricain, J. C. (2018). What is the benefit of using amniotic membrane in oral surgery? A comprehensive review of clinical studies. Clin. Oral Invest 22, 1881–1891. doi:10.1007/s00784-018-2457-3
Fujiwara, N., and Kobayashi, K. (2005). Macrophages in inflammation. Cdtia 4, 281–286. doi:10.2174/1568010054022024
Gu, Y. Z., Xue, Q., Chen, Y. J., Yu, G. H., Qing, M. D., Shen, Y., et al. (2013). Different roles of PD-L1 and FasL in immunomodulation mediated by human placenta-derived mesenchymal stem cells. Hum. Immunol. 74, 267–276. doi:10.1016/j.humimm.2012.12.011
Gulameabasse, S., Gindraux, F., Catros, S., Fricain, J. C., and Fenelon, M. (2020). Chorion and amnion/chorion membranes in oral and periodontal surgery: A systematic review. J. Biomed. Mater Res. 109 (8), 1216–1229. doi:10.1002/jbm.b.34783
Güler, R., Ercan, M. T., Ulutunçel, N., Devrim, H., and Uran, N. (1997). Measurement of blood flow by the 133Xe clearance technique to grafts of amnion used in vestibuloplasty. Br. J. Oral Maxillofac. Surg. 35, 280–283. doi:10.1016/s0266-4356(97)90048-6
Han, Y., Sun, T., Han, Y., Lin, L., Liu, C., Liu, J., et al. (2019). Human umbilical cord mesenchymal stem cells implantation accelerates cutaneous wound healing in diabetic rats via the Wnt signaling pathway. Eur. J. Med. Res. 24, 10. doi:10.1186/s40001-019-0366-9
Hasegawa, T., Mizoguchi, M., Haruna, K., Mizuno, Y., Muramatsu, S., Suga, Y., et al. (2007). Amnia for intractable skin ulcers with recessive dystrophic epidermolysis bullosa: Report of three cases. J. Dermatol 34, 328–332. doi:10.1111/j.1346-8138.2007.00281.x
Hashemi, S. S., Mohammadi, A. A., Kabiri, H., Hashempoor, M. R., Mahmoodi, M., Amini, M., et al. (2019). The healing effect of wharton's jelly stem cells seeded on biological scaffold in chronic skin ulcers: A randomized clinical trial. J. Cosmet. Dermatol 18, 1961–1967. doi:10.1111/jocd.12931
Hashemi, S. S., Pourfath, M. R., Derakhshanfar, A., Behzad-Behbahani, A., and Moayedi, J. (2020). The role of labeled cell therapy with and without scaffold in early excision burn wounds in a rat animal model. Iran. J. Basic Med. Sci. 23, 673–679. doi:10.22038/ijbms.2020.34324.8156
Hashemian, S. R., Aliannejad, R., Zarrabi, M., Soleimani, M., Vosough, M., Hosseini, S. E., et al. (2021). Mesenchymal stem cells derived from perinatal tissues for treatment of critically ill COVID-19-induced ARDS patients: A case series. Stem Cell Res. Ther. 12, 91. doi:10.1186/s13287-021-02165-4
He, L., Tu, H. J., He, W. F., Guo, L. L., Yu, S. X., Li, J., et al. (2015). Lentiviral-mediated overexpression of homeobox A4 by human umbilical cord mesenchymal stem cells repairs full-thickness skin defects. Mol. Med. Rep. 11, 3517–3522. doi:10.3892/mmr.2015.3208
He, X., Shu, J., Xu, L., Lu, C., and Lu, A. (2012). Inhibitory effect of Astragalus polysaccharides on lipopolysaccharide-induced TNF-a and IL-1β production in THP-1 cells. Molecules 17, 3155–3164. doi:10.3390/molecules17033155
Hu, K., Yang, Y., Tu, Q., Luo, Y., and Ma, R. (2013). Alpinetin inhibits LPS-induced inflammatory mediator response by activating PPAR-γ in THP-1-derived macrophages. Eur. J. Pharmacol. 721, 96–102. doi:10.1016/j.ejphar.2013.09.049
Hu, Y., Rao, S. S., Wang, Z. X., Cao, J., Tan, Y. J., Luo, J., et al. (2018). Exosomes from human umbilical cord blood accelerate cutaneous wound healing through miR-21-3p-mediated promotion of angiogenesis and fibroblast function. Theranostics 8, 169–184. doi:10.7150/thno.21234
Huang, Q., Yang, Y., Luo, C., Wen, Y., Liu, R., Li, S., et al. (2019). An efficient protocol to generate placental chorionic plate-derived mesenchymal stem cells with superior proliferative and immunomodulatory properties. Stem Cell Res. Ther. 10, 301. doi:10.1186/s13287-019-1405-8
Insausti, C. L., Alcaraz, A., García-Vizcaíno, E. M., Mrowiec, A., López-Martínez, M. C., Blanquer, M., et al. (2010). Amniotic membrane induces epithelialization in massive posttraumatic wounds. Wound Repair Regen. 18, 368–377. doi:10.1111/j.1524-475x.2010.00604.x
Jin, E., Kim, T. H., Han, S., and Kim, S. W. (2016). Amniotic epithelial cells promote wound healing in mice through high epithelialization and engraftment. J. Tissue Eng. Regen. Med. 10, 613–622. doi:10.1002/term.2069
Kaushik, K., and Das, A. (2019). Cycloxygenase-2 inhibition potentiates trans-differentiation of Wharton's jelly-mesenchymal stromal cells into endothelial cells: Transplantation enhances neovascularization-mediated wound repair. Cytotherapy 21, 260–273. doi:10.1016/j.jcyt.2019.01.004
Kim, S. W., Zhang, H. Z., Guo, L., Kim, J. M., and Kim, M. H. (2012a). Amniotic mesenchymal stem cells enhance wound healing in diabetic NOD/SCID mice through high angiogenic and engraftment capabilities. Plos One 7. doi:10.1371/annotation/f6ebe3d3-ef7c-42ce-86fe-d5a661d7f67f
Kim, S. W., Zhang, H. Z., Guo, L., Kim, J. M., and Kim, M. H. (2012b). Amniotic mesenchymal stem cells enhance wound healing in diabetic NOD/SCID mice through high angiogenic and engraftment capabilities. PLoS One 7, e41105. doi:10.1371/journal.pone.0041105
Kim, S. Y., Oh, S. H., Lee, J. H., Suh, M. W., and Park, M. K. (2019). Effects of placenta-derived mesenchymal stem cells on the particulate matter-induced damages in human middle ear epithelial cells. Stem Cells Int. 2019, 4357684. doi:10.1155/2019/4357684
Kim, Y. J., Ahn, H. J., Lee, S. H., Lee, M. H., and Kang, K. S. (2020). Effects of conditioned media from human umbilical cord blood-derived mesenchymal stem cells in the skin immune response. Biomed. Pharmacother. 131, 110789. doi:10.1016/j.biopha.2020.110789
Kitala, D., Klama-Baryła, A., Łabuś, W., Ples, M., Misiuga, M., Kraut, M., et al. (2019). Amniotic cells share clusters of differentiation of fibroblasts and keratinocytes, influencing their ability to proliferate and aid in wound healing while impairing their angiogenesis capability. Eur. J. Pharmacol. 854, 167–178. doi:10.1016/j.ejphar.2019.02.043
Kleiveland, C. R. (2015). “Peripheral blood mononuclear cells,” in The impact of food bioactives on health: In vitro and ex vivo models. Editors K. VERHOECKX, COTTER, P., LOPEZ-EXPOSITO, I., C. KLEIVELAND, T. LEA, A. MACKIE, T. REQUENA, D. SWIATECKAet al. (Cham. (CH). doi:10.1007/978-3-319-16104-4_15
Kocherova, I., Bryja, A., Mozdziak, P., Angelova Volponi, A., Dyszkiewicz-Konwińska, M., Piotrowska-Kempisty, H., et al. (2019). Human umbilical vein endothelial cells (HUVECs) Co-culture with osteogenic cells: From molecular communication to engineering prevascularised bone grafts. J. Clin. Med. 8. doi:10.3390/jcm8101602
König, J., Weiss, G., Rossi, D., Wankhammer, K., Reinisch, A., Kinzer, M., et al. (2015). Placental mesenchymal stromal cells derived from blood vessels or avascular tissues: What is the better choice to support endothelial cell function? Stem Cells Dev. 24, 115–131. doi:10.1089/scd.2014.0115
Koob, T. J., Lim, J. J., Massee, M., Zabek, N., Rennert, R., Gurtner, G., et al. (2014). Angiogenic properties of dehydrated human amnion/chorion allografts: Therapeutic potential for soft tissue repair and regeneration. Vasc. Cell 6, 10. doi:10.1186/2045-824x-6-10
Koob, T. J., Rennert, R., Zabek, N., Massee, M., Lim, J. J., Temenoff, J. S., et al. (2013). Biological properties of dehydrated human amnion/chorion composite graft: Implications for chronic wound healing. Int. Wound J. 10, 493–500. doi:10.1111/iwj.12140
Lawson, V. G. (1985). Oral cavity reconstruction using pectoralis major muscle and amnion. Archives Otolaryngology - Head Neck Surg. 111, 230–233. doi:10.1001/archotol.1985.00800060054006
Lee, C. E. (2018). Macrophages and inflammation. J. Rheum. Dis. 25, 11–18. doi:10.4078/jrd.2018.25.1.11
Leng, S. X., Mcelhaney, J. E., Walston, J. D., Xie, D., Fedarko, N. S., and Kuchel, G. A. (2008). ELISA and multiplex technologies for cytokine measurement in inflammation and aging research. J. Gerontol. A Biol. Sci. Med. Sci. 63, 879–884. doi:10.1093/gerona/63.8.879
Li, H., Niederkorn, J. Y., Neelam, S., Mayhew, E., Word, R. A., Mcculley, J. P., et al. (2005). Immunosuppressive factors secreted by human amniotic epithelial cells. Invest Ophthalmol. Vis. Sci. 46, 900–907. doi:10.1167/iovs.04-0495
Li, J., Koike-Soko, C., Sugimoto, J., Yoshida, T., Okabe, M., and Nikaido, T. (2015). Human amnion-derived stem cells have immunosuppressive properties on NK cells and monocytes. Cell Transpl. 24, 2065–2076. doi:10.3727/096368914x685230
Li, J. Y., Ren, K. K., Zhang, W. J., Xiao, L., Wu, H. Y., Liu, Q. Y., et al. (2019). Human amniotic mesenchymal stem cells and their paracrine factors promote wound healing by inhibiting heat stress-induced skin cell apoptosis and enhancing their proliferation through activating PI3K/AKT signaling pathway. Stem Cell Res. Ther. 10, 247. doi:10.1186/s13287-019-1366-y
Liarte, S., Bernabe-Garcia, A., Armero-Barranco, D., and Nicolas, F. J. (2018). Microscopy based methods for the assessment of epithelial cell migration during in vitro wound healing. J. Vis. Exp. 1, 56799. doi:10.3791/56799
Liarte, S., Bernabe-Garcia, A., and Nicolas, F. J. (2020a). Human skin keratinocytes on sustained TGF-beta stimulation reveal partial EMT features and weaken growth arrest responses. Cells 9, 255. doi:10.3390/cells9010255
Liarte, S., Bernabe-Garcia, A., and Nicolas, F. J. (2020b). Role of TGF-beta in skin chronic wounds: A keratinocyte perspective. Cells 9. doi:10.3390/cells9020306
Liu, J., Yan, Z., Yang, F., Huang, Y., Yu, Y., Zhou, L., et al. (2021). Exosomes derived from human umbilical cord mesenchymal stem cells accelerate cutaneous wound healing by enhancing angiogenesis through delivering angiopoietin-2. Stem Cell Rev. Rep. 17, 305–317. doi:10.1007/s12015-020-09992-7
Lublin, F. D., Bowen, J. D., Huddlestone, J., Kremenchutzky, M., Carpenter, A., Corboy, J. R., et al. (2014). Human placenta-derived cells (PDA-001) for the treatment of adults with multiple sclerosis: A randomized, placebo-controlled, multiple-dose study. Mult. Scler. Relat. Disord. 3, 696–704. doi:10.1016/j.msard.2014.08.002
Ma, H., Jiang, S., DU, L., Liu, J., Xu, X., Lu, X., et al. (2021). Conditioned medium from primary cytotrophoblasts, primary placenta-derived mesenchymal stem cells, or sub-cultured placental tissue promoted HUVEC angiogenesis in vitro. Stem Cell Res. Ther. 12, 141. doi:10.1186/s13287-021-02192-1
Ma, Y., Liu, X., Long, Y., and Chen, Y. (2022). Emerging therapeutic potential of mesenchymal stem cell-derived extracellular vesicles in chronic respiratory diseases: An overview of recent progress. Front. Bioeng. Biotechnol. 10, 845042. doi:10.3389/fbioe.2022.845042
Magatti, M., Caruso, M., DE Munari, S., Vertua, E., De, D., Manuelpillai, U., et al. (2015). Human amniotic membrane-derived mesenchymal and epithelial cells exert different effects on monocyte-derived dendritic cell differentiation and function. Cell Transpl. 24 (9), 1733–1752. doi:10.3727/096368914X684033
Magatti, M., DE Munari, S., Vertua, E., Gibelli, L., Wengler, G. S., and Parolini, O. (2008). Human amnion mesenchyme harbors cells with allogeneic T-cell suppression and stimulation capabilities. Stem Cells 26, 182–192. doi:10.1634/stemcells.2007-0491
Magatti, M., DE Munari, S., Vertua, E., Nassauto, C., Albertini, A., Wengler, G. S., et al. (2009). Amniotic mesenchymal tissue cells inhibit dendritic cell differentiation of peripheral blood and amnion resident monocytes. Cell Transpl. 18, 899–914. doi:10.3727/096368909x471314
Magatti, M., Vertua, E., Cargnoni, A., Silini, A., and Parolini, O. (2018). The immunomodulatory properties of amniotic cells: The two sides of the coin. Cell Transpl. 27, 31–44. doi:10.1177/0963689717742819
Mahmood, R., Mehmood, A., Choudhery, M. S., Awan, S. J., Khan, S. N., and Riazuddin, S. (2019). Human neonatal stem cell-derived skin substitute improves healing of severe burn wounds in a rat model. Cell Biol. Int. 43, 147–157. doi:10.1002/cbin.11072
Marshall, J. S., Warrington, R., Watson, W., and Kim, H. L. (2018). An introduction to immunology and immunopathology. Allergy Asthma Clin. Immunol. 14, 49. doi:10.1186/s13223-018-0278-1
Mcquilling, J. P., Kammer, M., Kimmerling, K. A., and Mowry, K. C. (2019). Characterisation of dehydrated amnion chorion membranes and evaluation of fibroblast and keratinocyte responses in vitro. Int. Wound J. 16, 827–840. doi:10.1111/iwj.13103
Mebarki, M., Iglicki, N., Marigny, C., Abadie, C., Nicolet, C., Churlaud, G., et al. (2021). Development of a human umbilical cord-derived mesenchymal stromal cell-based advanced therapy medicinal product to treat immune and/or inflammatory diseases. Stem Cell Res. Ther. 12, 571. doi:10.1186/s13287-021-02637-7
Medina-Leyte, D. J., Domínguez-Pérez, M., Ingrid Mercado, I., Villarreal-Molina, M. T., and Jacobo-Albavera, L. (2020). Use of human umbilical vein endothelial cells (HUVEC) as a model to study cardiovascular disease. Appl. Sci. 10, 938. doi:10.3390/app10030938
Medzhitov, R. (2008). Origin and physiological roles of inflammation. Nature 454, 428–435. doi:10.1038/nature07201
Mermet, I., Pottier, N., Sainthillier, J. M., Malugani, C., Cairey-Remonnay, S., Maddens, S., et al. (2007). Use of amniotic membrane transplantation in the treatment of venous leg ulcers. Wound Repair Regen. 15, 459–464. doi:10.1111/j.1524-475x.2007.00252.x
Moby, V., Boura, C., Kerdjoudj, H., Voegel, J. C., Marchal, L., Dumas, D., et al. (2007). Poly(styrenesulfonate)/poly(allylamine) multilayers: A route to favor endothelial cell growth on expanded poly(tetrafluoroethylene) vascular grafts. Biomacromolecules 8, 2156–2160. doi:10.1021/bm070348n
Moloudizargari, M., Govahi, A., Fallah, M., Rezvanfar, M. A., Asghari, M. H., and Abdollahi, M. (2021). The mechanisms of cellular crosstalk between mesenchymal stem cells and natural killer cells: Therapeutic implications. J. Cell Physiol. 236, 2413–2429. doi:10.1002/jcp.30038
Momeni, M., Zarehaghighi, M., Hajimiri, M., Khorasani, G., Dinarvand, R., Nekookar, A., et al. (2018). In vitro and in vivo investigation of a novel amniotic-based chitosan dressing for wound healing. Wound Repair Regen. 26, 87–101. doi:10.1111/wrr.12618
Montanucci, P., DI Pasquali, C., Ferri, I., Pescara, T., Pennoni, I., Siccu, P., et al. (2017). Human umbilical cord Wharton jelly-derived adult mesenchymal stem cells, in biohybrid scaffolds, for experimental skin regeneration. Stem Cells Int. 2017, 1472642. doi:10.1155/2017/1472642
Moreno-Jimenez, I., Hulsart-Billstrom, G., Lanham, S. A., Janeczek, A. A., Kontouli, N., Kanczler, J. M., et al. (2016). The chorioallantoic membrane (CAM) assay for the study of human bone regeneration: A refinement animal model for tissue engineering. Sci. Rep. 6, 32168. doi:10.1038/srep32168
Mukherjee, C., Saleem, S., Das, S., Biswas, S. C., and Bhattacharyya, D. (2020). Human placental laminin: Role in neuronal differentiation, cell adhesion and proliferation. J. Biosci. 45. doi:10.1007/s12038-020-00043-4
Munir, H., Rainger, G. E., Nash, G. B., and Mcgettrick, H. (2015). Analyzing the effects of stromal cells on the recruitment of leukocytes from flow. J. Vis. Exp. 1, e52480. doi:10.3791/52480
Murphy, S. V., Skardal, A., Song, L., Sutton, K., Haug, R., Mack, D. L., et al. (2017). Solubilized amnion membrane hyaluronic acid hydrogel accelerates full-thickness wound healing. Stem Cells Transl. Med. 6, 2020–2032. doi:10.1002/sctm.17-0053
Nensat, C., Songjang, W., Tohtong, R., Suthiphongchai, T., Phimsen, S., Rattanasinganchan, P., et al. (2021). Porcine placenta extract improves high-glucose-induced angiogenesis impairment. BMC Complement. Med. Ther. 21, 66. doi:10.1186/s12906-021-03243-z
Nicolas-Avila, J. A., Adrover, J. M., and Hidalgo, A. (2017). Neutrophils in homeostasis, immunity, and cancer. Immunity 46, 15–28. doi:10.1016/j.immuni.2016.12.012
Nowak-Sliwinska, P., Alitalo, K., Allen, E., Anisimov, A., Aplin, A. C., Auerbach, R., et al. (2018). Consensus guidelines for the use and interpretation of angiogenesis assays. Angiogenesis 21, 425–532. doi:10.1007/s10456-018-9613-x
Pandolfi, A., and De Filippis, E. A. (2007). Chronic hyperglicemia and nitric oxide bioavailability play a pivotal role in pro-atherogenic vascular modifications. Genes Nutr. 2, 195–208. doi:10.1007/s12263-007-0050-5
Paternotte, E., Kerdjoudj, H., Kokten, T., Stoltz, J. F., Kearney-Schwartz, A., Voegel, J. C., et al. (2013). Endothelialized and preconditioned natural umbilical arteries with long term patency open the route for future human uses. Clin. Hemorheol. Microcirc. 54, 223–234. doi:10.3233/ch-131728
Pianta, S., Bonassi Signoroni, P., Muradore, I., Rodrigues, M. F., Rossi, D., Silini, A., et al. (2015). Amniotic membrane mesenchymal cells-derived factors skew T cell polarization toward Treg and downregulate Th1 and Th17 cells subsets. Stem Cell Rev. Rep. 11, 394–407. doi:10.1007/s12015-014-9558-4
Pichlsberger, M., Jerman, U. D., Obradović, H., Tratnjek, L., Macedo, A. S., Mendes, F., et al. (2021). Systematic review of the application of perinatal derivatives in animal models on cutaneous wound healing. Front. Bioeng. Biotechnol. 9. doi:10.3389/fbioe.2021.742858
Pipino, C., Bernabe-Garcia, A., Cappellaci, I., Stelling-Ferez, J., DI Tomo, P., Santalucia, M., et al. (2022). Effect of the human amniotic membrane on the umbilical vein endothelial cells of gestational diabetic mothers: New insight on inflammation and angiogenesis. Front. Bioeng. Biotechnol. 10. doi:10.3389/fbioe.2022.854845
Pipino, C., Shah, H., Prudente, S., DI Pietro, N., Zeng, L., Park, K., et al. (2020). Association of the 1q25 diabetes-specific coronary heart disease locus with alterations of the gamma-glutamyl cycle and increased methylglyoxal levels in endothelial cells. Diabetes 69, 2206–2216. doi:10.2337/db20-0475
Platchek, M., Lu, Q., Tran, H., and Xie, W. (2020). Comparative analysis of multiple immunoassays for cytokine profiling in drug Discovery. SLAS Discov. 25, 1197–1213. doi:10.1177/2472555220954389
Rahman, M. S., Islam, R., Rana, M. M., Spitzhorn, L. S., Rahman, M. S., Adjaye, J., et al. (2019). Characterization of burn wound healing gel prepared from human amniotic membrane and Aloe vera extract. BMC Complement. Altern. Med. 19, 115. doi:10.1186/s12906-019-2525-5
Raja, Sivamani, K., Garcia, M. S., and Isseroff, R. R. (2007). Wound re-epithelialization: Modulating keratinocyte migration in wound healing. Front. Biosci. 12, 2849–2868. doi:10.2741/2277
Rameshbabu, A. P., Bankoti, K., Datta, S., Subramani, E., Apoorva, A., Ghosh, P., et al. (2018). Silk sponges ornamented with a placenta-derived extracellular matrix augment full-thickness cutaneous wound healing by stimulating neovascularization and cellular migration. ACS Appl. Mater Interfaces 10, 16977–16991. doi:10.1021/acsami.7b19007
Riddy, D. M., Goy, E., Delerive, P., Summers, R. J., Sexton, P. M., and Langmead, C. J. (2018). Comparative genotypic and phenotypic analysis of human peripheral blood monocytes and surrogate monocyte-like cell lines commonly used in metabolic disease research. PLoS One 13, e0197177. doi:10.1371/journal.pone.0197177
Rinastiti, M., Harijadisantoso, A. L., and Sosroseno, W. (2006). Histological evaluation of rabbit gingival wound healing transplanted with human amniotic membrane. Int. J. Oral Maxillofac. Surg. 35, 247–251. doi:10.1016/j.ijom.2005.09.012
Ringden, O., Baygan, A., Remberger, M., Gustafsson, B., Winiarski, J., Khoein, B., et al. (2018). Placenta-derived decidua stromal cells for treatment of severe acute graft-versus-host disease. Stem Cells Transl. Med. 7, 325–331. doi:10.1002/sctm.17-0167
Rossi, D., Pianta, S., Magatti, M., Sedlmayr, P., and Parolini, O. (2012). Characterization of the conditioned medium from amniotic membrane cells: Prostaglandins as key effectors of its immunomodulatory activity. PLoS One 7, e46956. doi:10.1371/journal.pone.0046956
Ruiz-Canada, C., Bernabe-Garcia, A., Liarte, S., Insausti, C. L., Angosto, D., Moraleda, J. M., et al. (2018). Amniotic membrane stimulates cell migration by modulating transforming growth factor-beta signalling. J. Tissue Eng. Regen. Med. 12, 808–820. doi:10.1002/term.2501
Ruiz-Canada, C., Bernabe-Garcia, A., Liarte, S., Rodriguez-Valiente, M., and Nicolas, F. J. (2021). Chronic wound healing by amniotic membrane: TGF-beta and EGF signaling modulation in Re-epithelialization. Front. Bioeng. Biotechnol. 9, 689328. doi:10.3389/fbioe.2021.689328
Sabapathy, V., Sundaram, B., Mankuzhy, P., and Kumar, S. (2014). Human Wharton's Jelly Mesenchymal Stem Cells plasticity augments scar-free skin wound healing with hair growth. PLoS One 9, e93726. doi:10.1371/journal.pone.0093726
Sabzevari, R., Roushandeh, A. M., Mehdipour, A., Alini, M., and Roudkenar, M. H. (2020). SA/G hydrogel containing hCAP-18/LL-37-engineered WJ-MSCs-derived conditioned medium promoted wound healing in rat model of excision injury. Life Sci. 261, 118381. doi:10.1016/j.lfs.2020.118381
Sadeghi, B., Roshandel, E., Pirsalehi, A., Kazemi, S., Sankanian, G., Majidi, M., et al. (2021). Conquering the cytokine storm in COVID-19-induced ARDS using placenta-derived decidua stromal cells. J. Cell Mol. Med. 25, 10554–10564. doi:10.1111/jcmm.16986
Samandari, M. H., Yaghmaei, M., Ejlali, M., Moshref, M., and Saffar, A. S. (2004). Use of amnion as a graft material in vestibuloplasty: A preliminary report. Oral Surg. Oral Med. Oral Pathol. Oral Radiol. Endod. 97, 574–578. doi:10.1016/j.tripleo.2003.10.031
Shu, J., He, X., Zhang, L., Li, H., Wang, P., and Huang, X. (2015). Human amnion mesenchymal cells inhibit lipopolysaccharide-induced TNF-alpha and IL-1beta production in THP-1 cells. Biol. Res. 48, 69. doi:10.1186/s40659-015-0062-3
Silini, A., Parolini, O., Huppertz, B., and Lang, I. (2013). Soluble factors of amnion-derived cells in treatment of inflammatory and fibrotic pathologies. Curr. Stem Cell Res. Ther. 8, 6–14. doi:10.2174/1574888x11308010003
Silini, A. R., Cargnoni, A., Magatti, M., Pianta, S., and Parolini, O. (2015). The long path of human placenta, and its derivatives, in regenerative medicine. Front. Bioeng. Biotechnol. 3, 162. doi:10.3389/fbioe.2015.00162
Silini, A. R., DI Pietro, R., Lang-Olip, I., Alviano, F., Banerjee, A., Basile, M., et al. (2020). Perinatal derivatives: Where do we stand? A roadmap of the human placenta and consensus for tissue and cell nomenclature. Front. Bioeng. Biotechnol. 8, 610544. doi:10.3389/fbioe.2020.610544
Singh, R., Chouhan, U. S., Purohit, S., Gupta, P., Kumar, P., Kumar, A., et al. (2004). Radiation processed amniotic membranes in the treatment of non-healing ulcers of different etiologies. Cell Tissue Bank. 5, 129–134. doi:10.1023/b:catb.0000034077.05000.29
Solomon, A., Wajngarten, M., Alviano, F., Anteby, I., Elchalal, U., Pe'Er, J., et al. (2005). Suppression of inflammatory and fibrotic responses in allergic inflammation by the amniotic membrane stromal matrix. Clin. Exp. Allergy 35, 941–948. doi:10.1111/j.1365-2222.2005.02285.x
Stojadinovic, A., Carlson, J. W., Schultz, G. S., Davis, T. A., and Elster, E. A. (2008). Topical advances in wound care. Gynecol. Oncol. 111, S70–S80. doi:10.1016/j.ygyno.2008.07.042
Strech, D., and Dirnagl, U. (2019). 3Rs missing: Animal research without scientific value is unethical. BMJ Open Sci. 3. doi:10.1136/bmjos-2018-000048
Sun, J., Zhang, Y., Song, X., Zhu, J., and Zhu, Q. (2019). The healing effects of conditioned medium derived from mesenchymal stem cells on radiation-induced skin wounds in rats. Cell Transpl. 28, 105–115. doi:10.1177/0963689718807410
Sun, Q., Li, F., Li, H., Chen, R. H., Gu, Y. Z., Chen, Y., et al. (2015). Amniotic fluid stem cells provide considerable advantages in epidermal regeneration: B7H4 creates a moderate inflammation microenvironment to promote wound repair. Sci. Rep. 5, 11560. doi:10.1038/srep11560
Talwadekar, M. D., Kale, V. P., and Limaye, L. S. (2015). Placenta-derived mesenchymal stem cells possess better immunoregulatory properties compared to their cord-derived counterparts-a paired sample study. Sci Rep. 5, 15784. doi:10.1038/srep15784
Thery, C., Witwer, K. W., Aikawa, E., Alcaraz, M. J., Anderson, J. D., Andriantsitohaina, R., et al. (2018). Minimal information for studies of extracellular vesicles 2018 (MISEV2018): A position statement of the international society for extracellular vesicles and update of the MISEV2014 guidelines. J. Extracell. Vesicles 7, 1535750. doi:10.1080/20013078.2018.1535750
Torre, P., and Flores, A. I. (2020). Current status and future prospects of perinatal stem cells. Genes (Basel) 12, 6. doi:10.3390/genes12010006
Turabelidze, A., Guo, S., Chung, A. Y., Chen, L., Dai, Y., Marucha, P. T., et al. (2014). Intrinsic differences between oral and skin keratinocytes. PLoS One 9, e101480. doi:10.1371/journal.pone.0101480
Ucci, M., DI Tomo, P., Tritschler, F., Cordone, V. G. P., Lanuti, P., Bologna, G., et al. (2019). Anti-inflammatory role of carotenoids in endothelial cells derived from umbilical cord of women affected by gestational diabetes mellitus. Oxid. Med. Cell Longev. 2019, 8184656. doi:10.1155/2019/8184656
Van Deun, J., Mestdagh, P., Agostinis, P., Akay, O., Anand, S., Anckaert, J., et al. EV-TRACK Consortium. (2017). EV-TRACK: Transparent reporting and centralizing knowledge in extracellular vesicle research. Nat. Methods 14, 228–232. doi:10.1038/nmeth.4185
Vellasamy, S., Sandrasaigaran, P., Vidyadaran, S., Abdullah, M., George, E., and Ramasamy, R. (2013). Mesenchymal stem cells of human placenta and umbilical cord suppress T-cell proliferation at G0 phase of cell cycle. Cell Biol. Int. 37, 250–256. doi:10.1002/cbin.10033
Vonbrunn, E., Mueller, M., Pichlsberger, M., Sundl, M., Helmer, A., Wallner, S. A., et al. (2020). Electrospun PCL/PLA scaffolds are more suitable carriers of placental mesenchymal stromal cells than collagen/elastin scaffolds and prevent wound contraction in a mouse model of wound healing. Front. Bioeng. Biotechnol. 8, 604123. doi:10.3389/fbioe.2020.604123
Wang, G., Joel, M. D. M., Yuan, J., Wang, J., Cai, X., Ocansey, D. K. W., et al. (2020). Human umbilical cord mesenchymal stem cells alleviate inflammatory bowel disease by inhibiting ERK phosphorylation in neutrophils. Inflammopharmacology 28, 603–616. doi:10.1007/s10787-019-00683-5
Wang, H., Chen, L., Liu, Y., Luo, B., Xie, N., Tan, T., et al. (2016a). Implantation of placenta-derived mesenchymal stem cells accelerates murine dermal wound closure through immunomodulation. Am. J. Transl. Res. 8, 4912–4921.
Wang, S., Mo, M., Wang, J., Sadia, S., Shi, B., Fu, X., et al. (2018). Platelet-derived growth factor receptor beta identifies mesenchymal stem cells with enhanced engraftment to tissue injury and pro-angiogenic property. Cell Mol. Life Sci. 75, 547–561. doi:10.1007/s00018-017-2641-7
Wang, S., Yang, H., Tang, Z., Long, G., and Huang, W. (2016b). Wound dressing model of human umbilical cord mesenchymal stem cells-alginates complex promotes skin wound healing by paracrine signaling. Stem Cells Int. 2016, 3269267. doi:10.1155/2016/3269267
Wertz, P. W. (2021). Synopsis of barrier function of skin and oral mucosa-volume 1. Int. J. Mol. Sci. 22. doi:10.3390/ijms22179383
Winkler, T., Costa, M. L., Ofir, R., Parolini, O., Geissler, S., Volk, H. D., et al. (2022). Hipgen: A randomized, multicentre phase III study using intramuscular PLacenta-eXpanded stromal cells therapy for recovery following hip fracture arthroplasty : A study design. Bone Jt. Open 3, 340–347. doi:10.1302/2633-1462.34.bjo-2021-0156.r1
Yang, C., Wu, M., You, M., Chen, Y., Luo, M., and Chen, Q. (2021). The therapeutic applications of mesenchymal stromal cells from human perinatal tissues in autoimmune diseases. Stem Cell Res. Ther. 12, 103. doi:10.1186/s13287-021-02158-3
Yang, J., Chen, Z., Pan, D., Li, H., and Shen, J. (2020). Umbilical cord-derived mesenchymal stem cell-derived exosomes combined pluronic F127 hydrogel promote chronic diabetic wound healing and complete skin regeneration. Int. J. Nanomedicine 15, 5911–5926. doi:10.2147/ijn.s249129
Yang, K., Li, D., Wang, M., Xu, Z., Chen, X., Liu, Q., et al. (2019). Exposure to blue light stimulates the proangiogenic capability of exosomes derived from human umbilical cord mesenchymal stem cells. Stem Cell Res. Ther. 10, 358. doi:10.1186/s13287-019-1472-x
Yue, C., Guo, Z., Luo, Y., Yuan, J., Wan, X., and Mo, Z. (2020). c-Jun overexpression accelerates wound healing in diabetic rats by human umbilical cord-derived mesenchymal stem cells. Stem Cells Int. 2020, 7430968. doi:10.1155/2020/7430968
Zantl, R., and Horn, E. (2011). Chemotaxis of slow migrating mammalian cells analysed by video microscopy. Methods Mol. Biol. 769, 191–203. doi:10.1007/978-1-61779-207-6_13
Zhang, B., Shi, Y., Gong, A., Pan, Z., Shi, H., Yang, H., et al. (2016). HucMSC exosome-delivered 14-3-3zeta orchestrates self-control of the wnt response via modulation of YAP during cutaneous regeneration. Stem Cells 34, 2485–2500. doi:10.1002/stem.2432
Zhang, B., Wu, X., Zhang, X., Sun, Y., Yan, Y., Shi, H., et al. (2015). Human umbilical cord mesenchymal stem cell exosomes enhance angiogenesis through the Wnt4/beta-catenin pathway. Stem Cells Transl. Med. 4, 513–522. doi:10.5966/sctm.2014-0267
Zhang, S., Chen, L., Zhang, G., and Zhang, B. (2020). Umbilical cord-matrix stem cells induce the functional restoration of vascular endothelial cells and enhance skin wound healing in diabetic mice via the polarized macrophages. Stem Cell Res. Ther. 11, 39. doi:10.1186/s13287-020-1561-x
Zhao, B., Liu, J. Q., Zheng, Z., Zhang, J., Wang, S. Y., Han, S. C., et al. (2016). Human amniotic epithelial stem cells promote wound healing by facilitating migration and proliferation of keratinocytes via ERK, JNK and AKT signaling pathways. Cell Tissue Res. 365, 85–99. doi:10.1007/s00441-016-2366-1
Zhao, G., Liu, F., Liu, Z., Zuo, K., Wang, B., Zhang, Y., et al. (2020). MSC-derived exosomes attenuate cell death through suppressing AIF nucleus translocation and enhance cutaneous wound healing. Stem Cell Res. Ther. 11, 174. doi:10.1186/s13287-020-01616-8
Keywords: perinatal derivatives, mesenchymal stromal cells, amniotic epithelial cells, amniotic membrane, functional assays, inflammation, angiogenesis, wound healing
Citation: Flores AI, Pipino C, Jerman UD, Liarte S, Gindraux F, Kreft ME, Nicolas FJ, Pandolfi A, Tratnjek L, Giebel B, Pozzobon M, Silini AR, Parolini O, Eissner G and Lang-Olip I (2022) Perinatal derivatives: How to best characterize their multimodal functions in vitro. Part C: Inflammation, angiogenesis, and wound healing. Front. Bioeng. Biotechnol. 10:965006. doi: 10.3389/fbioe.2022.965006
Received: 09 June 2022; Accepted: 07 July 2022;
Published: 04 August 2022.
Edited by:
Wolfgang Holnthoner, Ludwig Boltzmann Institute for Experimental and Clinical Traumatology, AustriaReviewed by:
Alfred Gugerell, Medical University of Vienna, AustriaBetül Çelebi Saltik, Hacettepe University, Turkey
Copyright © 2022 Flores, Pipino, Jerman, Liarte, Gindraux, Kreft, Nicolas, Pandolfi, Tratnjek, Giebel, Pozzobon, Silini, Parolini, Eissner and Lang-Olip. This is an open-access article distributed under the terms of the Creative Commons Attribution License (CC BY). The use, distribution or reproduction in other forums is permitted, provided the original author(s) and the copyright owner(s) are credited and that the original publication in this journal is cited, in accordance with accepted academic practice. No use, distribution or reproduction is permitted which does not comply with these terms.
*Correspondence: Günther Eissner, guenther.eissner@ucd.ie; Sergio Liarte, sdll1@um.es
†These authors have contributed equally to this work