Reprogramming Microbial CO2-Metabolizing Chassis With CRISPR-Cas Systems
- 1School of Environmental Science and Engineering, Shandong University, Qingdao, China
- 2State Key Laboratory of Microbial Technology, Shandong University, Qingdao, China
- 3Sino-French Research Institute for Ecology and Environment, Shandong University, Qingdao, China
Global warming is approaching an alarming level due to the anthropogenic emission of carbon dioxide (CO2). To overcome the challenge, the reliance on fossil fuels needs to be alleviated, and a significant amount of CO2 needs to be sequestrated from the atmosphere. In this endeavor, carbon-neutral and carbon-negative biotechnologies are promising ways. Especially, carbon-negative bioprocesses, based on the microbial CO2-metabolizing chassis, possess unique advantages in fixing CO2 directly for the production of fuels and value-added chemicals. In order to fully uncover the potential of CO2-metabolizing chassis, synthetic biology tools, such as CRISPR-Cas systems, have been developed and applied to engineer these microorganisms, revolutionizing carbon-negative biotechnology. Herein, we review the recent advances in the adaption of CRISPR-Cas systems, including CRISPR-Cas based genome editing and CRISPR interference/activation, in cyanobacteria, acetogens, and methanogens. We also envision future innovations via the implementation of rising CRISPR-Cas systems, such as base editing, prime editing, and transposon-mediated genome editing.
Introduction
Anthropogenic emission of carbon dioxide (CO2) has driven an unprecedented high level of CO2 in the atmosphere, leading to an approximately 1.1°C increase in the average global temperature (Tollefson, 2021). This increase has reached an alarming level and has caused global and local climate issues. It also leaves a very small window to achieve the 1.5°C target settled in the Paris Agreement and reinforced in the UN Climate Conference in Glasgow (COP26). The atmospheric CO2 level must be lowered by a significant amount by controlling the emission of CO2 and sequestering CO2 from the atmosphere at the same time. In this endeavor, biotechnology provides promising routes. In one way, carbon-neutral biotechnology utilizes sustainable carbon sources (e.g., agriculture and forest wastes) to produce chemicals (e.g., ethanol, butanol, and 2,3-butanediol), alleviating the reliance on fossil fuels and reducing CO2 emissions (Liu et al., 2020). In another way, carbon-negative biotechnology directly consumes industrial or atmospheric CO2 for the bioproduction of fuels and value-added chemicals (Liu et al., 2020; Liew et al., 2022).
With metabolic engineering and synthetic biology, the inventory of products from biological routes has been greatly expanded, and the production and yield have been improved. For instance, the baker’s yeast Saccharomyces cerevisiae has been genetically engineered to convert lignocellulosic feedstock to bioethanol and chemicals, exhibiting the potential of carbon-neutral biotechnology (Wei et al., 2013; Sun et al., 2021). Recently, S cerevisiae has been engineered to utilize wasteful CO2 accumulated during lignocellulosic sugar fermentation by the installation of a CO2 fixation pathway, transforming the correlated biotechnology from a carbon-neutral process to a carbon-negative technology (Li et al., 2017; Xia et al., 2017). Inspiringly, Gassler et al. (2020) generated an engineered yeast Pichia pastoris capably of growing with CO2 and methanol, opening a new window for heterotrophic yeast to use one-carbon (C1) compounds as sole carbon sources. Similar enterprises have been made in Escherichia coli, and artificial autotrophic E. coli has been generated via the implementation of CO2 fixation pathways and adaptive laboratory evolution (Antonovsky et al., 2016; Gleizer et al., 2019; Flamholz et al., 2020).
Another biological path is to employ microorganisms that metabolize CO2 innately, such as photoautotrophic cyanobacteria and chemoautotrophs, including acetogens and methanogens. These organisms can use CO2 as a carbon source from either industrial waste gases or the atmosphere (Fackler et al., 2021). CO2-metabolizing microorganisms have shown great potential as microbial chassis, and industrial attempts have been made (Liu et al., 2020; Liew et al., 2022). Given the advances in synthetic biology, these microbes play more important roles on the path towards a sustainable future with enhanced CO2 utilization efficiency and an expanded spectrum of products. For instance, cyanobacterium Synechocystis sp. PCC 6803 has been modularly engineered to produce a high titer of 1-butanol, short/medium-chain carbohydrate, and lactate from CO2 (Liu X. et al., 2019; Shabestary et al., 2021; Yunus et al., 2022). Lately, a pioneer study conducted by LanzaTech, Inc. (Skokie, IL, United States) shows that Clostridium autoethanogenum can convert syngas (consisting of CO2, CO, and H2) to acetone and isopropanol, and a pilot-scale fermentation in a 125-L scalable reactor was demonstrated (Liew et al., 2022). These advances have validated the capability of CO2-metabolizing chassis in the fixation of CO2 and production of value-added chemicals, and these succuss illustrated the ever-increasing power of synthetic biology in biotechnology.
CRISPR-Cas systems, the bacterial and archaeal immune systems, have been repurposed as synthetic biology tools for gene editing and regulation (Knott and Doudna, 2018). They have been revolutionizing biotechnology in fundamental ways. Though still in its infant stage, multiple CRISPR-Cas-based synthetic biology tools have been developed for cyanobacteria, acetogens, and methanogens, driving the rising of novel biotechnologies based on CO2-metabolizing microbes. Herein, we summarize the current progress of CRISPR-Cas systems in genetically engineering microbial CO2-metabolizing chassis, especially cyanobacteria, acetogens, and methanogens, for the conversion of CO2 to biofuels and value-added products, and we discuss the challenges and future endeavors in developing more efficient synthetic biology tools.
Microbial CO2 Metabolizing Organisms
Microbial CO2-metabolizing chassis, mainly autotrophic microorganisms, can use CO2 as a sole carbon source for catabolic and anabolic activities. Until now, six CO2 fixation pathways have been identified, among which the Calvin cycle and Wood-Ljungdahl Pathway are the most understood and applicable routes (Fuchs, 2011; Muller, 2019; Gleizer et al., 2020). Compared to the complementary physiochemical strategies, biological ways have advantages in forming carbon-carbon bonds with one-carbon (C1) building blocks using either solar energy or redox power from inorganic compounds, i.e., iron, sulfide, and ammonia, offering better opportunities for bioproduction by the microbes themselves or in combined biotic-abiotic processes (Li et al., 2012; Sakimoto et al., 2016; Jin et al., 2021).
Photoautotrophic organisms, such as microalgae and cyanobacteria, can fix CO2 with solar energy through the Calvin cycle and produce a large variety of organic compounds. Notably, efforts have been made to design and build engineered cyanobacteria for the production of biofuels (i.e., biodiesel, bioethanol, and isobutanol), value-added chemicals (Santos-Merino et al., 2019; Xia et al., 2019), and food-related products, such as starch (Luan et al., 2019). Besides the photoautotrophs, Cupriavidus necator (formerly Ralstonia eutropha), a facultative chemolithotroph, can grow on CO2 through the Calvin cycle as well with H2 or formate as the electron donor without light. C. necator has also been engineered as a novel chassis for bioproduction (Li et al., 2012; Panich et al., 2021). Chemoautotrophic organisms harboring the Wood-Ljungdahl Pathway can utilize CO2 and H2 anaerobically. As the key representatives, acetogens, especially strains from the class of Clostridia (e.g., C. autoethanogenum, Clostridium ljungdahlii, Acetobacterium woodii, and Eubacterium limosum), have been interrogated and engineered to utilize CO2 (with H2) or CO2-containing mixed gases (Muller, 2019; Fackler et al., 2021). Due to the requirement and capability of co-utilization of H2, acetogens can also be the bridge connecting bioproduction and “Power-to-Gas” technology, generating a novel nexus “Power-to-X” (Molitor et al., 2019; Mishra et al., 2020). Similarly, methanogens play important roles in different bioprocesses in various niches, such as in the gut, in soil, and in engineered systems (i.e., wastewater treatment facilities). They produce CH4 from CO2 and H2 or other one-carbon compounds, like methanol (Thauer et al., 2008; Zabranska and Pokorna, 2018). As methanogens are archaea, they typically possess unique industrial merits, including high tolerance to temperature and osmatic stress, making them advantageous CO2-metabolizing chassis.
CRISPR-Cas Based Systems
CRISPR-Cas-based synthetic biology tools are repurposed from the bacterial and archaeal immune systems (Jiang and Doudna, 2017), and the innovations in CRISPR-Cas-based systems have been reshaping biotechnology in fundamental ways (Knott and Doudna, 2018; Pickar-Oliver and Gersbach, 2019). For instance, an artificial autotrophic P. pastoris was generated via the integration of six foreign genes and deletion of three innate genes with the CRISPR-Cas-based gene-editing tool (Gassler et al., 2020). Recently, CRISPR-Cas-based methods have also been deployed to upgrade carbon-negative bioprocess by manipulating CO2-metabolizing chassis. In this section, we focus on the adaption of different CRISPR-Cas systems for the perturbation of CO2-metabolizing microbes. We highlight achievements and challenges in cyanobacteria, acetogens, and methanogens.
CRISPR-Cas-Based Genome Editing
CRISPR-Cas-based genome editing in microbes typically has two steps: RNA-guided DNA cleavage and DNA repair of the double-strand break, the latter of which eventually resulted in the editing of a target gene (Selle and Barrangou, 2015; Knott and Doudna, 2018). Taking the Class II Type II CRISPR-Cas system from Streptococcus pyogenes as an example, the single CRISPR effector Cas9 is led by a guide RNA (gRNA) consisting of a targeting sequence (spacer), which is complementary to the target sequence (protospacer), a CRISPR RNA (crRNA) and trans-activating CRISPR RNA (tracer RNA) (Jiang and Doudna, 2017). When the complex of Cas9 and gRNA reached the target sequence, it recognizes the protospacer when a protospacer adjacent motif (PAM) presents. Then, the Cas9 nuclease cleaves the DNA and leaves a double-strand break, generating a “dead or alive” scenario for the microbe (Vento et al., 2019). With DNA repair mechanisms, the target gene will be edited to survive the deadly cleavage of Cas9 (Figure 1A). CRISPR-Cas-based genome editing has been prosperous due to the ease of use and the clean editing products without leaving a marker or a scar.
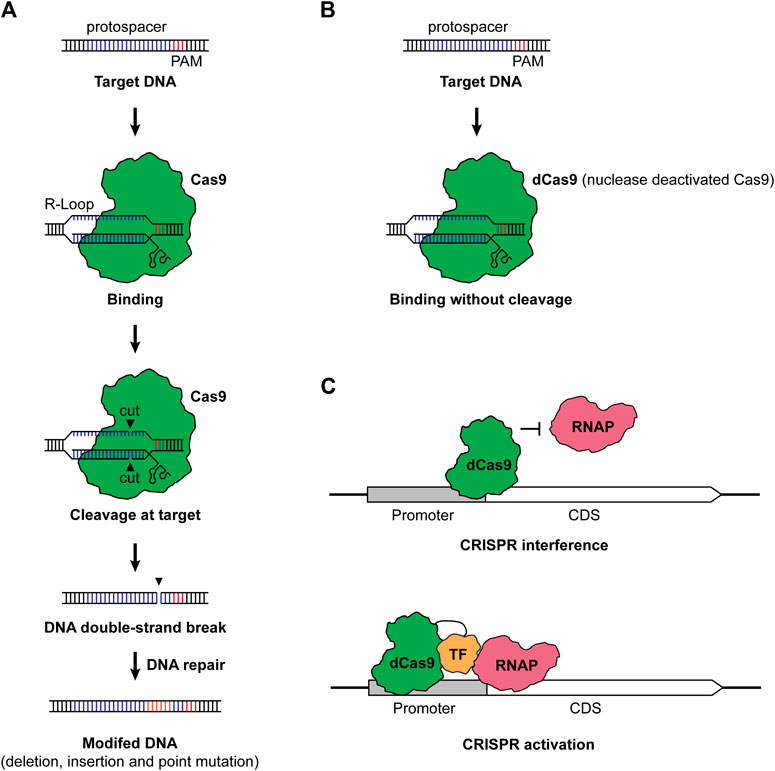
FIGURE 1. (A) CRISPR-Cas-based genome editing. Under the guidance of a gRNA containing a spacer complementary to the protospacer and a scaffold consisting of crRNA and tracer RNA, the Cas9 and gRNA complex finds and binds to the protospacer with a PAM, forming an R-loop. Then, Cas9 cuts the DNA in each strand, leaving a double-strand break (DSB) in the target. Finally, the target DNA will be modified with the repair of DSB. (B) Nuclease deactivated Cas9 protein (dCas9). When the nuclease activity of Cas protein was deactivated, the dCas9 still binds to the target sequence but it will not cleave the DNA anymore. (C) CRISPRi and CRISPRa. For CRISPRi, dCas9 binds to the promoter or coding region of a gene of interest and prevents the binding of RNAP, resulting in the repression of transcription. For CRISPRa, the dCas9-transcription factor (TF) fusion binds to the up region of the promoter. The TF helps recruit RNAP and allows the activation of transcription. We employed Cas9 from S. pyogenes as a representative in the figure, while a great variety of CRISPR systems can be used for genome editing, CRISPRi, and CRISPRa. TF stands for transcription factor, RNAP stands for RNA polymerase, CDS stands for coding sequence, and PAM stands for protospacer adjacent motif.
In cyanobacteria, CRISPR-Cas-based genome editing has been realized (Xia et al., 2019). Li et al. (2016) adapted a CRISPR-Cas genome editing tool for cyanobacterium Synechococcus elongatus PCC 7942 based on S. pyogenes Cas9 with a transient expression system. Later, a plasmid-based CRISPR-Cas system was developed for S. elongatus UTEX 2973, a fast-growing cyanobacterium showing great potential in sustainable bioproduction (Wendt et al., 2016). However, these countable successes implied the severe toxicity of Cas9 on cyanobacteria. Due to the toxicity of Cas9, conventional CRISPR-Cas-based genome editing tools with a “dead or alive” selection have not been thriving in cyanobacteria. To surmount this bottleneck, two strategies have been engaged. One is to use alternative Cas proteins. For instance, the Class II Type V CRISPR system with Cas12a as the effector showed lower toxicity than Cas9 to cyanobacteria. By using Cas12a, Ungerer and Pakrasi (2016) achieved CRISPR-Cas-based gene editing in S. elongatus UTEX 2973, Synechocystis sp. PCC 6803 and Anabaena sp. PCC 7102. Another way is to control the expression of the CRISPR-Cas system tightly. Hudson and colleagues hired a tightly regulated RNA device, the theophylline-responsive riboswitch, to maintain a low enough OFF-state expression of Cas9 to prevent its toxicity, and induce the genome-editing when required (Cengic et al., 2022). By applying this system, the reliable transformation of a replicable plasmid harboring CRISPR-Cas9 was obtained, leading to successful deletions and insertions of DNA fragments in the genome of Synechocystis. To our best knowledge, this study also reported multiplex genome editing in cyanobacteria for the first time regardless of methods. As multiple genes are typically involved in engineering a microbe for desired functionalities, multiplexing is of great importance in synthetic biology by saving considerable time and labor.
CRISPR-Cas-based genome editing tools have also been established in acetogens and methanogens. For acetogens, Cas9 and Cas12a based methods have been devised in C. autoethanogenum (Nagaraju et al., 2016), C. ljungdahlii (Huang et al., 2016; Zhao et al., 2019), and Eubacterium limosum (Shin et al., 2019). To be noted, enhanced genome editing was achieved via a combination of CRISPR-Cas and serine recombinase (Huang et al., 2019). As reported, a phage serine recombinase was used for the integration of large DNA fragments while CRISPR-Cas inserts a small recognition motif of the recombinase. With this method, a butyric acid production pathway was successfully introduced to C. ljungdahlii for the production of butyric acid from syngas (Huang et al., 2019). Similar to the abovementioned method for cyanobacteria, a tightly regulated system controlled by a riboswitch, namely RiboCas, was designed to enable CRISPR-Cas-based genome editing in Clostridium strains, including Clostridium pasteurianum, Clostridium difficile, and Clostridium sporogenes (Canadas et al., 2019). Moreover, CRISPR-Cas-based deletion and integration were accomplished in methanogen Methanosarcina acetivorans via applying an inducible CRISPR-Cas9 system from S. pyogenes (Nayak and Metcalf, 2017). In the same study, the authors reported CRISPR-based deletion via the implementation of a foreign non-homologous end-joining (NHEJ) machinery from Methanocella paludicola, enabling the deletion of gene fragments (75–2.7 kb) without repairing DNAs. Recently, CRISPR-Cas-based genome editing was reported in methanogenic archaea Methanococcus maripaludis with the Cas12a system from Lachnospiraceae bacterium (Bao et al., 2022), further expanding the genome editing tools for methanogens.
CRISPR Interference (CRISPRi) and CRISPR Activation (CRISPRA)
Actually, the toxicity of Cas proteins is not an exclusive issue in cyanobacteria, while most bacteria suffer from the toxic effects of CRISPR-Cas systems (Banno et al., 2018; Canadas et al., 2019; Vento et al., 2019). Despite screening alternative CRISPR-Cas systems and implementation of fine control modules, one alternative way is to employ the nuclease deactivated Cas protein (dCas) (Figure 1B). When the nuclease activity of Cas proteins is dead (dCas) or partially dead (nCas), dCas or nCas proteins are less toxic to bacteria compared to fully functional Cas effectors. By using dCas9, the resulting CRISPR-Cas system will no longer cleave the target DNA sequence but bind to the target. When dCas9 binds to the promoter or the coding sequence of a gene of interest, it will prevent the binding of RNA polymerase (RNAP), thus silencing the target gene at the transcription level and generating the method CRISPRi (Figure 1C) (Larson et al., 2013; Qi et al., 2013).
Due to the alleviated toxicity, CRISPRi obtained more popularity and has been developed for cyanobacteria. For instance, Yao et al. (2016) employed dCas9 to enable multiplex CRISPRi in Synechocystis sp. PCC 6803, Choi and Woo (2020) demonstrated CRISPRi with dCas12a in S. elongatus PCC 7942, and a dCas12a-based CRISPRi system was also established for S. elongatus UTEX 2973 (Knoot et al., 2020). In the former two reports, multiplex CRISPRi was achieved, and up to four genes were repressed at a single time, showing the huge potential of CRISPRi in engineering cyanobacteria for sustainable production (Yao et al., 2016; Choi and Woo, 2020). Notably, a CRISPRi system was devised for generating a gene repression library in Synechocystis sp. PCC 6803 in order to interrogate the genotype-phenotype interactions. By doing so, an industrially relevant strain with higher production of lactate, as a proof of principle, was engineered via CRISPRi-based repression of correlated essential genes (i.e., gltA and pcnB) related to lactate synthesis (Yao et al., 2020). More recently, CRISPRi was programmed as a genetic switch between cell growth and product synthesis. Shabestary et al. (2021) employed CRISPRi-based gltA regulation in a lactate-producing Synechocystis and achieve a high yield of lactate by decoupling cell growth and lactate production.
CRISPRi displays potential for acetogens and methanogens as well (Dhamad and Lessner, 2020; Fackler et al., 2021). Woolston et al. (2018) developed an inducible dCas9-based CRISPRi system for the repression of essential genes related to carbon metabolism in Clostridium ljungdahlii. Specifically, the pta gene encoding the phosphotransacetylase and the aor2 gene encoding the aldehyde:ferredoxin oxidoreductase were repressed with CRISPRi individually and in a multiplex mode, redirecting carbon from acetate to the desired product 3-hydroxybutyrate with significantly increased titer and yield (Woolston et al., 2018). In a pioneer study, a CRISPRi system was developed for archaeal methanogen M. acetivorans by applying S. pyogenes dCas9, and the system was evaluated by interrogating the gene cluster related to nitrogen fixation (nif operon) and its regulator (nrpR1) (Dhamad and Lessner, 2020).
Besides gene repression, transcriptional activation can also be possible with CRISPR activation (CRISPRa) (Bikard et al., 2013; Liu Y. et al., 2019). CRISPRa deploys a combination of dCas protein and transcription factor, such as the ω subunit of the RNAP. When CRISPRa targets the upstream of a promoter, it will help bring RNAP and activate the transcription of the corresponding gene (Figure 1C). Bikard et al. (2013) first developed a CRISPRa system for bacteria and employed the dCas9-ω fusion to allow upregulation of gfp and lacZ expressions in E. coli. Recently, more advances in CRISPRa have been reported in bacteria (Liu Y. et al., 2019; Schilling et al., 2020; Kiattisewee et al., 2021; Villegas Kcam et al., 2021; Tickman et al., 2022). Given these advances, new transcriptional factors have been systematically screened, and the application has been expanded from E. coli to other bacteria, including Paenibacillus polymyxa (Schilling et al., 2020) and Pseudomonas putida (Kiattisewee et al., 2021). Moreover, a full range of gene regulation from repression to upregulation has been achieved by programmable CRISPRi/a circuits (Tickman et al., 2022) and by designing the targeting loci (Liu Y. et al., 2019). Though not been adapted yet, the application of CRISPRa and CRISPRi/a circuits will be a powerful tool for gene regulations in CO2-metabolizing microbes.
Rising CRISPR-Cas Systems
Base Editing
A novel CRISPR-Cas-based genome editing was invented via combining nucleotide deamination, namely base editing (Figure 2A). For base editing, a dCas9 or nCas9 was fused with cytosine deaminase or adenine deaminase, and when binding to a target sequence, the deamination generates a mismatched base pair which will be repaired, resulting in C-to-T or A-to-G substitution (Komor et al., 2016; Nishida et al., 2016; Gaudelli et al., 2017). Along with emerging base editing methods, the single nucleotide substitution has been expanded to more combinations including C-to-G and C-to-A (Kurt et al., 2021; Zhao et al., 2021). Base editing was demonstrated in bacteria and exhibited prodigious capacities in engineering microbes for designed functionalities (Tong et al., 2019; Cheng et al., 2020; Rodrigues et al., 2021; Wu et al., 2021). Until now, one attempt reported the development and application of base editing in the chemoautotrophic acetogen. Xia et al. (2020) designed a C-to-T base-editing method for C. ljungdahlii using dCas9 and the activation-induced cytidine deaminase from the sea lamprey Petromyzon marinus, enabling precision genome editing at a one-nucleotide resolution. By applying this method, the carbon flux in C. ljungdahlii was redirected from ethanol to acetate, leading to increased production of acetate from CO2 and H2. Besides the common merits of CRISPR-Cas systems, base editing exhibits unique advantages in engineering CO2 metabolizing microbes in that: 1) the core module of base editing can be dCas or nCas which are less toxic to the host, 2) the system does not need repairing DNAs (donor DNA), and 3) it does not require high transformation efficiency to screen a survival cell from the direct “dead or alive” selection (Molla and Yang, 2019; Gu et al., 2021). These advantages make base editing a promising candidate for genome editing in CO2-metabolizing chassis.
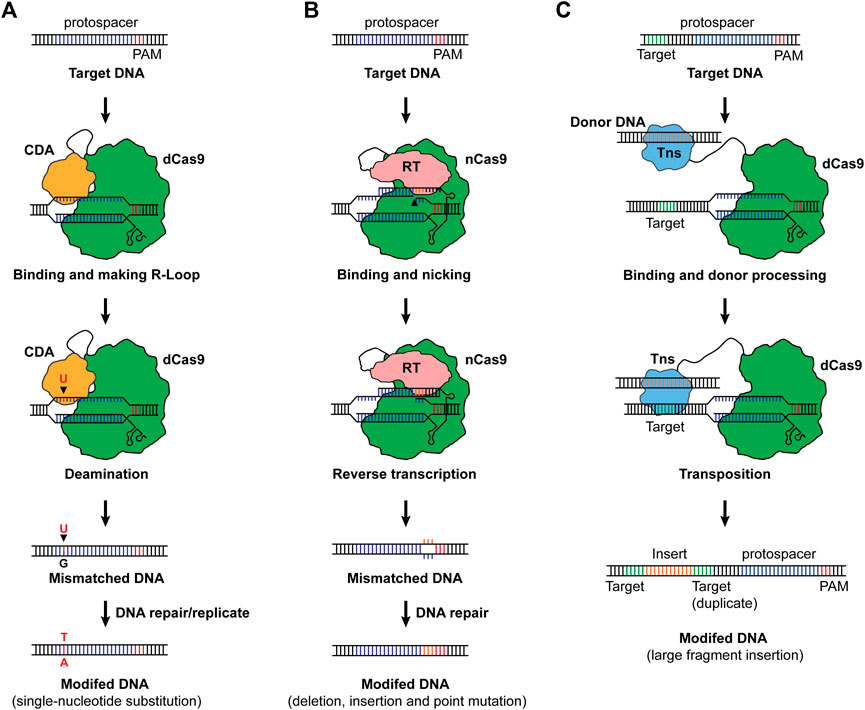
FIGURE 2. (A) Base editing. The cytosine base editor with a combination of dCas9 cytosine deaminase is shown as an example to illustrate the working mechanism. When dCas9 binds to its target sequence and an R-loop is formed, the cytosine deaminase (CDA) mutates a cytosine (C) to uracil (U), generating a uracil-guanine (U–G) mismatch. The C will then be replaced by thymine (T) along with the reparation of the mismatch upon DNA repair or replication, resulting in a C-to-T substitution at a one-nucleotide resolution. For adenine base editors, an A-to-G substitution will occur as a result of base editing. Notably, nCas9 can also be used as the effector for base editors. (B) Prime editing. The prime editing takes advantage of nCas9 and a fused reverse-transcription (RT). By designing a pegRNA consisting of the spacer for targeting, RT template with designed edits, and primer binding site (PBS), deletions, insertions and point mutations can be achieved. (C) Transposon-mediated CRISPR-Cas system for DNA integration. As a paradigm, dCas9 was fused to a transposase (Tns), and when the transposase was led to the target sequence, it recognizes the motif for transposition and integrates the donor DNA into the upstream of the protospacer, enabling large fragment insertions. Cas9 was used as the Cas effector for illustration of the basic working principles of base editing, prime editing and transposon-mediated editing.
Prime Editing and Transposon-Mediated Integration
Despite the merits of base editing, the precision feature of base editing limits its capability in the deletion and insertion of DNA fragments. As a one-step forward, prime-editing was invented via a combination of nCas9 and a reverse-transcriptase, allowing the insertion of small DNA fragments without generating DNA double-strand breaks or requiring a donor DNA (Anzalone et al., 2019) (Figure 2B). Tong et al. (2021) further adapted this method for bacteria, making possible the introduction of deletions, insertions, and nucleotide substitutions with prime editing in E. coli. More specifically, an up to 97 bp of DNA fragment was deleted, and an up to 33 bp of DNA fragment was inserted into the genome of E. coli with high fidelity and efficiency (Tong et al., 2021). To enable large DNA insertion, a more recent study invented a twin-prime system with a prime editing system and serine recombinase. This system first inserts two motifs using the twin prime editing systems, and then the motifs will be recognized by the serine recombinase. Upon activation of the serine recombinase and the presence of a donor DNA, the DNA fragment can be integrated into the genome (over 5,000 bp of DNA fragment) or be inverted (up to 40 kb of DNA fragment) (Anzalone et al., 2021).
Another CRISPR-Cas-based tool for insertion of large-DNA-fragment is the transposon-mediated integration (Klompe et al., 2019; Strecker et al., 2019) (Figure 2C). Strecker et al. (2019) discovered a CRISPR-associated transposase from Scytonema hofmanni containing a Tn7-like transposase and a Type V-K Cas protein (Cas12k) and achieved insertion of 60–66 bp DNA fragment. Another work designed a system with dCas9 and Tn7-like transposon, enabling the integration of DNA fragments. The results show that the system is able to insert 1,000 bp of fragments with maximum efficiency, and efficient integration could also be achieved with a larger fragment (Klompe et al., 2019). Given these advances, prime editing and transposon-mediated integration, though not been realized yet, may offer powerful synthetic biology tools for genome editing in CO2-metabolizing microorganisms.
Concluding Remarks
In this review, we summarized recent advances in developing and applying CRISPR-Cas systems for CO2-metabolizing chassis. CRISPR-Cas-based genome editing and CRISPRi, have been reported in these microbes, and the methods have been advancing biorefinery and bioproduction with CO2 as the carbon source, exhibiting great potential in alleviating CO2 emissions and in reducing atmospheric CO2 levels. However, more efforts are imperative to awake the full power of CRISPR-Cas systems in these CO2-metabolizing chassis. CRISPRa, base editing, prime editing, and transposon-mediated integration may offer encouraging future directions in developing novel CRISPR-Cas systems for CO2-metabolizing microorganisms. Moreover, discoveries of new CRISPR-Cas systems with special properties (e.g., a thermostable Cas9) are needed to engineer CO2-metabolizing microorganisms, such as thermophilic strains Thermoanaerobacter kivui and Methanothermobacter thermautotrophicus (Moon et al., 2019; Fink et al., 2021).
Besides CO2, CO, methane, methanol and formate are also important greenhouse gases and C1 compounds that can be obtained from waste gases and products or byproducts of clean energy industries. As such, natural and engineered C1-metabolizing microbes, including but not constrained in the autotrophs discussed here, will also be favorable microbial chassis for sustainable bioproduction. The development of novel synthetic biological tools, such as CRISPR-Cas systems, for C1 metabolizing organisms, will significantly foster innovations in carbon-negative biotechnologies.
Author Contributions
H-YY, S-GW, and P-FX wrote the manuscript.
Funding
This work was supported by the National Natural Science Foundation of China (U20A20146 and 42076091), the Distinguished Young Scholar Program of Shandong Province (Overseas) (2022HWYQ-017) and the Qilu Young Scholar Program of Shandong University (to P-FX).
Conflict of Interest
The authors declare that the research was conducted in the absence of any commercial or financial relationships that could be construed as a potential conflict of interest.
Publisher’s Note
All claims expressed in this article are solely those of the authors and do not necessarily represent those of their affiliated organizations, or those of the publisher, the editors and the reviewers. Any product that may be evaluated in this article, or claim that may be made by its manufacturer, is not guaranteed or endorsed by the publisher.
References
Antonovsky, N., Gleizer, S., Noor, E., Zohar, Y., Herz, E., Barenholz, U., et al. (2016). Sugar Synthesis from CO2 in Escherichia coli. Cell 166 (1), 115–125. doi:10.1016/j.cell.2016.05.064
Anzalone, A. V., Gao, X. D., Podracky, C. J., Nelson, A. T., Koblan, L. W., Raguram, A., et al. (2021). Programmable Deletion, Replacement, Integration and Inversion of Large DNA Sequences with Twin Prime Editing. Nat. Biotechnol. 40, 731–740. doi:10.1038/s41587-021-01133-w
Anzalone, A. V., Randolph, P. B., Davis, J. R., Sousa, A. A., Koblan, L. W., Levy, J. M., et al. (2019). Search-and-replace Genome Editing without Double-Strand Breaks or Donor DNA. Nature 576 (7785), 149–157. doi:10.1038/s41586-019-1711-4
Banno, S., Nishida, K., Arazoe, T., Mitsunobu, H., and Kondo, A. (2018). Deaminase-mediated Multiplex Genome Editing in Escherichia coli. Nat. Microbiol. 3 (4), 423–429. doi:10.1038/s41564-017-0102-6
Bao, J., de Dios Mateos, E., and Scheller, S. (2022). Efficient CRISPR/Cas12a-based Genome Editing Toolbox for Metabolic Engineering in Methanococcus Maripaludis. bioRxiv. 2021.2012.2029.474413. doi:10.1101/2021.12.29.474413
Bikard, D., Jiang, W., Samai, P., Hochschild, A., Zhang, F., and Marraffini, L. A. (2013). Programmable Repression and Activation of Bacterial Gene Expression Using an Engineered CRISPR-Cas System. Nucleic Acids Res. 41 (15), 7429–7437. doi:10.1093/nar/gkt520
Cañadas, I. C., Groothuis, D., Zygouropoulou, M., Rodrigues, R., and Minton, N. P. (2019). RiboCas: a Universal CRISPR-Based Editing Tool for Clostridium. ACS Synth. Biol. 8 (6), 1379–1390. doi:10.1021/acssynbio.9b00075
Cengic, I., Cañadas, I. C., Minton, N. P., and Hudson, E. P. (2022). Inducible CRISPR/Cas9 Allows for Multiplexed and Rapidly Segregated Single Target Genome Editing in Synechocystis Sp. PCC 6803. bioRxiv, 482598. 2022.2003.2002. doi:10.1101/2022.03.02.482598
Cheng, L., Min, D., He, R. L., Cheng, Z. H., Liu, D. F., and Yu, H. Q. (2020). Developing a Base-Editing System to Expand the Carbon Source Utilization Spectra of Shewanella Oneidensis MR-1 for Enhanced Pollutant Degradation. Biotechnol. Bioeng. 117 (8), 2389–2400. doi:10.1002/bit.27368
Choi, S. Y., and Woo, H. M. (2020). CRISPRi-dCas12a: a dCas12a-Mediated CRISPR Interference for Repression of Multiple Genes and Metabolic Engineering in Cyanobacteria. ACS Synth. Biol. 9 (9), 2351–2361. doi:10.1021/acssynbio.0c00091
Dhamad, A. E., and Lessner, D. J. (2020). A CRISPRi-dCas9 System for Archaea and its Use to Examine Gene Function during Nitrogen Fixation by Methanosarcina Acetivorans. Appl. Environ. Microbiol. 86 (21). doi:10.1128/AEM.01402-20
Fackler, N., Heijstra, B. D., Rasor, B. J., Brown, H., Martin, J., Ni, Z., et al. (2021). Stepping on the Gas to a Circular Economy: Accelerating Development of Carbon-Negative Chemical Production from Gas Fermentation. Annu. Rev. Chem. Biomol. Eng. 12, 439–470. doi:10.1146/annurev-chembioeng-120120-021122
Fink, C., Beblawy, S., Enkerlin, A. M., Mühling, L., Angenent, L. T., and Molitor, B. (2021). A Shuttle-Vector System Allows Heterologous Gene Expression in the Thermophilic Methanogen Methanothermobacter Thermautotrophicus ΔH. mBio 12 (6), e0276621. doi:10.1128/mBio.02766-21
Flamholz, A. I., Dugan, E., Blikstad, C., Gleizer, S., Ben-Nissan, R., Amram, S., et al. (2020). Functional Reconstitution of a Bacterial CO2 Concentrating Mechanism in Escherichia coli. elife 9. doi:10.7554/eLife.59882
Fuchs, G. (2011). Alternative Pathways of Carbon Dioxide Fixation: Insights into the Early Evolution of Life? Annu. Rev. Microbiol. 65 (1), 631–658. doi:10.1146/annurev-micro-090110-102801
Gassler, T., Sauer, M., Gasser, B., Egermeier, M., Troyer, C., Causon, T., et al. (2020). The Industrial Yeast Pichia pastoris Is Converted from a Heterotroph into an Autotroph Capable of Growth on CO2. Nat. Biotechnol. 38 (2), 210–216. doi:10.1038/s41587-019-0363-0
Gaudelli, N. M., Komor, A. C., Rees, H. A., Packer, M. S., Badran, A. H., Bryson, D. I., et al. (2017). Programmable Base Editing of at to GC in Genomic DNA without DNA Cleavage. Nature 551 (7681), 464–471. doi:10.1038/nature24644
Gleizer, S., Bar-On, Y. M., Ben-Nissan, R., and Milo, R. (2020). Engineering Microbes to Produce Fuel, Commodities, and Food from CO2. Cell Rep. Phys. Sci. 1 (10), 100223. doi:10.1016/j.xcrp.2020.100223
Gleizer, S., Ben-Nissan, R., Bar-On, Y. M., Antonovsky, N., Noor, E., Zohar, Y., et al. (2019). Conversion of Escherichia coli to Generate All Biomass Carbon from CO2. Cell 179 (6), 1255–1263. e1212. doi:10.1016/j.cell.2019.11.009
Gu, S., Bodai, Z., Cowan, Q. T., and Komor, A. C. (2021). Base Editors: Expanding the Types of DNA Damage Products Harnessed for Genome Editing. Gene Genome Ed. 1, 100005. doi:10.1016/j.ggedit.2021.100005
Huang, H., Chai, C., Li, N., Rowe, P., Minton, N. P., Yang, S., et al. (2016). CRISPR/Cas9-based Efficient Genome Editing in Clostridium Ljungdahlii, an Autotrophic Gas-Fermenting Bacterium. ACS Synth. Biol. 5 (12), 1355–1361. doi:10.1021/acssynbio.6b00044
Huang, H., Chai, C., Yang, S., Jiang, W., and Gu, Y. (2019). Phage Serine Integrase-Mediated Genome Engineering for Efficient Expression of Chemical Biosynthetic Pathway in Gas-Fermenting Clostridium Ljungdahlii. Metab. Eng. 52, 293–302. doi:10.1016/j.ymben.2019.01.005
Jiang, F., and Doudna, J. A. (2017). CRISPR-Cas9 Structures and Mechanisms. Annu. Rev. Biophys. 46 (1), 505–529. doi:10.1146/annurev-biophys-062215-010822
Jin, S., Jeon, Y., Jeon, M. S., Shin, J., Song, Y., Kang, S., et al. (2021). Acetogenic Bacteria Utilize Light-Driven Electrons as an Energy Source for Autotrophic Growth. Proc. Natl. Acad. Sci. U.S.A. 118 (9), e2020552118. doi:10.1073/pnas.2020552118
Kiattisewee, C., Dong, C., Fontana, J., Sugianto, W., Peralta-Yahya, P., Carothers, J. M., et al. (2021). Portable Bacterial CRISPR Transcriptional Activation Enables Metabolic Engineering in Pseudomonas Putida. Metab. Eng. 66, 283–295. doi:10.1016/j.ymben.2021.04.002
Klompe, S. E., Vo, P. L. H., Halpin-Healy, T. S., and Sternberg, S. H. (2019). Transposon-encoded CRISPR-Cas Systems Direct RNA-Guided DNA Integration. Nature 571 (7764), 219–225. doi:10.1038/s41586-019-1323-z
Knoot, C. J., Biswas, S., and Pakrasi, H. B. (2020). Tunable Repression of Key Photosynthetic Processes Using Cas12a CRISPR Interference in the Fast-Growing Cyanobacterium Synechococcus Sp. UTEX 2973. ACS Synth. Biol. 9 (1), 132–143. doi:10.1021/acssynbio.9b00417
Knott, G. J., and Doudna, J. A. (2018). CRISPR-cas Guides the Future of Genetic Engineering. Science 361 (6405), 866–869. doi:10.1126/science.aat5011
Komor, A. C., Kim, Y. B., Packer, M. S., Zuris, J. A., and Liu, D. R. (2016). Programmable Editing of a Target Base in Genomic DNA without Double-Stranded DNA Cleavage. Nature 533 (7603), 420–424. doi:10.1038/nature17946
Kurt, I. C., Zhou, R., Iyer, S., Garcia, S. P., Miller, B. R., Langner, L. M., et al. (2021). CRISPR C-To-G Base Editors for Inducing Targeted DNA Transversions in Human Cells. Nat. Biotechnol. 39 (1), 41–46. doi:10.1038/s41587-020-0609-x
Larson, M. H., Gilbert, L. A., Wang, X., Lim, W. A., Weissman, J. S., and Qi, L. S. (2013). CRISPR Interference (CRISPRi) for Sequence-specific Control of Gene Expression. Nat. Protoc. 8 (11), 2180–2196. doi:10.1038/nprot.2013.132
Li, H., Opgenorth, P. H., Wernick, D. G., Rogers, S., Wu, T.-Y., Higashide, W., et al. (2012). Integrated Electromicrobial Conversion of CO2 to Higher Alcohols. Science 335 (6076), 1596. doi:10.1126/science.1217643
Li, H., Shen, C. R., Huang, C.-H., Sung, L.-Y., Wu, M.-Y., and Hu, Y.-C. (2016). CRISPR-Cas9 for the Genome Engineering of Cyanobacteria and Succinate Production. Metab. Eng. 38, 293–302. doi:10.1016/j.ymben.2016.09.006
Li, Y.-J., Wang, M.-M., Chen, Y.-W., Wang, M., Fan, L.-H., and Tan, T.-W. (2017). Engineered Yeast with a CO2-fixation Pathway to Improve the Bio-Ethanol Production from Xylose-Mixed Sugars. Sci. Rep. 7 (1), 43875. doi:10.1038/srep43875
Liew, F. E., Nogle, R., Abdalla, T., Rasor, B. J., Canter, C., Jensen, R. O., et al. (2022). Carbon-negative Production of Acetone and Isopropanol by Gas Fermentation at Industrial Pilot Scale. Nat. Biotechnol. 40, 335–344. doi:10.1038/s41587-021-01195-w
Liu, X., Miao, R., Lindberg, P., and Lindblad, P. (2019a). Modular Engineering for Efficient Photosynthetic Biosynthesis of 1-butanol from CO2 in Cyanobacteria. Energy Environ. Sci. 12 (9), 2765–2777. doi:10.1039/c9ee01214a
Liu, Y., Wan, X., and Wang, B. (2019b). Engineered CRISPRa Enables Programmable Eukaryote-like Gene Activation in Bacteria. Nat. Commun. 10 (1), 3693. doi:10.1038/s41467-019-11479-0
Liu, Z., Wang, K., Chen, Y., Tan, T., and Nielsen, J. (2020). Third-generation Biorefineries as the Means to Produce Fuels and Chemicals from CO2. Nat. Catal. 3 (3), 274–288. doi:10.1038/s41929-019-0421-5
Luan, G., Zhang, S., Wang, M., and Lu, X. (2019). Progress and Perspective on Cyanobacterial Glycogen Metabolism Engineering. Biotechnol. Adv. 37 (5), 771–786. doi:10.1016/j.biotechadv.2019.04.005
Mishra, A., Ntihuga, J. N., Molitor, B., and Angenent, L. T. (2020). Power-to-Protein: Carbon Fixation with Renewable Electric Power to Feed the World. Joule 4 (6), 1142–1147. doi:10.1016/j.joule.2020.04.008
Molitor, B., Mishra, A., and Angenent, L. T. (2019). Power-to-protein: Converting Renewable Electric Power and Carbon Dioxide into Single Cell Protein with a Two-Stage Bioprocess. Energy Environ. Sci. 12 (12), 3515–3521. doi:10.1039/c9ee02381j
Molla, K. A., and Yang, Y. (2019). CRISPR/Cas-mediated Base Editing: Technical Considerations and Practical Applications. Trends Biotechnol. 37 (10), 1121–1142. doi:10.1016/j.tibtech.2019.03.008
Moon, J., Henke, L., Merz, N., and Basen, M. (2019). A Thermostable Mannitol-1-Phosphate Dehydrogenase Is Required in Mannitol Metabolism of the Thermophilic Acetogenic bacterium Thermoanaerobacter Kivui. Environ. Microbiol. 21 (10), 3728–3736. doi:10.1111/1462-2920.14720
Müller, V. (2019). New Horizons in Acetogenic Conversion of One-Carbon Substrates and Biological Hydrogen Storage. Trends Biotechnol. 37 (12), 1344–1354. doi:10.1016/j.tibtech.2019.05.008
Nagaraju, S., Davies, N. K., Walker, D. J. F., Köpke, M., and Simpson, S. D. (2016). Genome Editing of Clostridium Autoethanogenum Using CRISPR/Cas9. Biotechnol. Biofuels 9 (1), 219. doi:10.1186/s13068-016-0638-3
Nayak, D. D., and Metcalf, W. W. (2017). Cas9-mediated Genome Editing in the Methanogenic Archaeon Methanosarcina Acetivorans. Proc. Natl. Acad. Sci. U.S.A. 114 (11), 2976–2981. doi:10.1073/pnas.1618596114
Nishida, K., Arazoe, T., Yachie, N., Banno, S., Kakimoto, M., Tabata, M., et al. (2016). Targeted Nucleotide Editing Using Hybrid Prokaryotic and Vertebrate Adaptive Immune Systems. Science 353 (6305), aaf8729. PMID - 27492474. doi:10.1126/science.aaf8729
Panich, J., Fong, B., and Singer, S. W. (2021). Metabolic Engineering of Cupriavidus Necator H16 for Sustainable Biofuels from CO2. Trends Biotechnol. 39 (4), 412–424. doi:10.1016/j.tibtech.2021.01.001
Pickar-Oliver, A., and Gersbach, C. A. (2019). The Next Generation of CRISPR-Cas Technologies and Applications. Nat. Rev. Mol. Cell Biol. 20 (8), 490–507. doi:10.1038/s41580-019-0131-5
Qi, L. S., Larson, M. H., Gilbert, L. A., Doudna, J. A., Weissman, J. S., Arkin, A. P., et al. (2013). Repurposing CRISPR as an RNA-Guided Platform for Sequence-specific Control of Gene Expression. Cell 152 (5), 1173–1183. doi:10.1016/j.cell.2013.02.022
Rodrigues, S. D., Karimi, M., Impens, L., Van Lerberge, E., Coussens, G., Aesaert, S., et al. (2021). Efficient CRISPR-Mediated Base Editing in Agrobacterium Spp. Proc. Natl. Acad. Sci. U.S.A. 118 (2). doi:10.1073/pnas.2013338118
Sakimoto, K. K., Wong, A. B., and Yang, P. (2016). Self-photosensitization of Nonphotosynthetic Bacteria for Solar-To-Chemical Production. Science 351 (6268), 74–77. doi:10.1126/science.aad3317
Santos-Merino, M., Singh, A. K., and Ducat, D. C. (2019). New Applications of Synthetic Biology Tools for Cyanobacterial Metabolic Engineering. Front. Bioeng. Biotechnol. 7, 33. doi:10.3389/fbioe.2019.00033
Schilling, C., Koffas, M. A. G., Sieber, V., and Schmid, J. (2020). Novel Prokaryotic CRISPR-Cas12a-Based Tool for Programmable Transcriptional Activation and Repression. ACS Synth. Biol. 9 (12), 3353–3363. doi:10.1021/acssynbio.0c00424
Selle, K., and Barrangou, R. (2015). Harnessing CRISPR-Cas Systems for Bacterial Genome Editing. Trends Microbiol. 23 (4), 225–232. doi:10.1016/j.tim.2015.01.008
Shabestary, K., Hernández, H. P., Miao, R., Ljungqvist, E., Hallman, O., Sporre, E., et al. (2021). Cycling between Growth and Production Phases Increases Cyanobacteria Bioproduction of Lactate. Metab. Eng. 68, 131–141. doi:10.1016/j.ymben.2021.09.010
Shin, J., Kang, S., Song, Y., Jin, S., Lee, J. S., Lee, J.-K., et al. (2019). Genome Engineering of Eubacterium Limosum Using Expanded Genetic Tools and the CRISPR-Cas9 System. ACS Synth. Biol. 8 (9), 2059–2068. doi:10.1021/acssynbio.9b00150
Strecker, J., Ladha, A., Gardner, Z., Schmid-Burgk, J. L., Makarova, K. S., Koonin, E. V., et al. (2019). RNA-guided DNA Insertion with CRISPR-Associated Transposases. Science 365 (6448), 48–53. doi:10.1126/science.aax9181
Sun, L., Lee, J. W., Yook, S., Lane, S., Sun, Z., Kim, S. R., et al. (2021). Complete and Efficient Conversion of Plant Cell Wall Hemicellulose into High-Value Bioproducts by Engineered Yeast. Nat. Commun. 12 (1), 4975. doi:10.1038/s41467-021-25241-y
Thauer, R. K., Kaster, A.-K., Seedorf, H., Buckel, W., and Hedderich, R. (2008). Methanogenic Archaea: Ecologically Relevant Differences in Energy Conservation. Nat. Rev. Microbiol. 6 (8), 579–591. doi:10.1038/nrmicro1931
Tickman, B. I., Burbano, D. A., Chavali, V. P., Kiattisewee, C., Fontana, J., Khakimzhan, A., et al. (2022). Multi-layer CRISPRa/i Circuits for Dynamic Genetic Programs in Cell-free and Bacterial Systems. Cell Syst. 13 (3), 215–229. e218. doi:10.1016/j.cels.2021.10.008
Tollefson, J. (2021). IPCC Climate Report: Earth Is Warmer Than It's Been in 125,000 Years. Nature 596 (7871), 171–172. doi:10.1038/d41586-021-02179-1
Tong, Y., Jørgensen, T. S., Whitford, C. M., Weber, T., and Lee, S. Y. (2021). A Versatile Genetic Engineering Toolkit for E. coli Based on CRISPR-Prime Editing. Nat. Commun. 12 (1), 5206. doi:10.1038/s41467-021-25541-3
Tong, Y., Whitford, C. M., Robertsen, H. L., Blin, K., Jørgensen, T. S., Klitgaard, A. K., et al. (2019). Highly Efficient DSB-free Base Editing for Streptomycetes with CRISPR-BEST. Proc. Natl. Acad. Sci. U.S.A. 116 (41), 20366–20375. doi:10.1073/pnas.1913493116
Ungerer, J., and Pakrasi, H. B. (2016). Cpf1 Is a Versatile Tool for CRISPR Genome Editing across Diverse Species of Cyanobacteria. Sci. Rep. 6, 39681. doi:10.1038/srep39681
Vento, J. M., Crook, N., and Beisel, C. L. (2019). Barriers to Genome Editing with CRISPR in Bacteria. J. Ind. Microbiol. Biotechnol. 46 (9-10), 1327–1341. doi:10.1007/s10295-019-02195-1
Villegas Kcam, M. C., Tsong, A. J., and Chappell, J. (2021). Rational Engineering of a Modular Bacterial CRISPR-Cas Activation Platform with Expanded Target Range. Nucleic Acids Res. 49 (8), 4793–4802. doi:10.1093/nar/gkab211
Wei, N., Quarterman, J., Kim, S. R., Cate, J. H. D., and Jin, Y.-S. (2013). Enhanced Biofuel Production through Coupled Acetic Acid and Xylose Consumption by Engineered Yeast. Nat. Commun. 4 (1), 2580. doi:10.1038/ncomms3580
Wendt, K. E., Ungerer, J., Cobb, R. E., Zhao, H., and Pakrasi, H. B. (2016). CRISPR/Cas9 Mediated Targeted Mutagenesis of the Fast Growing Cyanobacterium Synechococcus Elongatus UTEX 2973. Microb. Cell Fact. 15 (1), 115. doi:10.1186/s12934-016-0514-7
Woolston, B. M., Emerson, D. F., Currie, D. H., and Stephanopoulos, G. (2018). Rediverting Carbon Flux in Clostridium Ljungdahlii Using CRISPR Interference (CRISPRi). Metab. Eng. 48, 243–253. doi:10.1016/j.ymben.2018.06.006
Wu, J., Liu, D. F., Li, H. H., Min, D., Liu, J. Q., Xu, P., et al. (2021). Controlling Pathogenic Risks of Water Treatment Biotechnologies at the Source by Genetic Editing Means. Environ. Microbiol. 23 (12), 7578–7590. doi:10.1111/1462-2920.15851
Xia, P.-F., Casini, I., Schulz, S., Klask, C.-M., Angenent, L. T., and Molitor, B. (2020). Reprogramming Acetogenic Bacteria with CRISPR-Targeted Base Editing via Deamination. ACS Synth. Biol. 9 (8), 2162–2171. doi:10.1021/acssynbio.0c00226
Xia, P.-F., Zhang, G.-C., Walker, B., Seo, S.-O., Kwak, S., Liu, J.-J., et al. (2017). Recycling Carbon Dioxide during Xylose Fermentation by Engineered Saccharomyces cerevisiae. ACS Synth. Biol. 6 (2), 276–283. doi:10.1021/acssynbio.6b00167
Xia, P. F., Ling, H., Foo, J. L., and Chang, M. W. (2019). Synthetic Biology Toolkits for Metabolic Engineering of Cyanobacteria. Biotechnol. J. 14 (6), 1800496. doi:10.1002/biot.201800496
Yao, L., Cengic, I., Anfelt, J., and Hudson, E. P. (2016). Multiple Gene Repression in Cyanobacteria Using CRISPRi. ACS Synth. Biol. 5 (3), 207–212. doi:10.1021/acssynbio.5b00264
Yao, L., Shabestary, K., Björk, S. M., Asplund-Samuelsson, J., Joensson, H. N., Jahn, M., et al. (2020). Pooled CRISPRi Screening of the Cyanobacterium Synechocystis Sp PCC 6803 for Enhanced Industrial Phenotypes. Nat. Commun. 11 (1), 1666. doi:10.1038/s41467-020-15491-7
Yunus, I. S., Anfelt, J., Sporre, E., Miao, R., Hudson, E. P., and Jones, P. R. (2022). Synthetic Metabolic Pathways for Conversion of CO2 into Secreted Short-To Medium-Chain Hydrocarbons Using Cyanobacteria. Metab. Eng. 72, 14–23. doi:10.1016/j.ymben.2022.01.017
Zabranska, J., and Pokorna, D. (2018). Bioconversion of Carbon Dioxide to Methane Using Hydrogen and Hydrogenotrophic Methanogens. Biotechnol. Adv. 36 (3), 707–720. doi:10.1016/j.biotechadv.2017.12.003
Zhao, D., Li, J., Li, S., Xin, X., Hu, M., Price, M. A., et al. (2021). Glycosylase Base Editors Enable C-To-A and C-To-G Base Changes. Nat. Biotechnol. 39 (1), 35–40. doi:10.1038/s41587-020-0592-2
Keywords: carbon dioxide, CRISPR, genome editing, cyanobacteria, acetogen, methanogen
Citation: Yu H-Y, Wang S-G and Xia P-F (2022) Reprogramming Microbial CO2-Metabolizing Chassis With CRISPR-Cas Systems. Front. Bioeng. Biotechnol. 10:897204. doi: 10.3389/fbioe.2022.897204
Received: 15 March 2022; Accepted: 07 June 2022;
Published: 23 June 2022.
Edited by:
Shuobo Shi, Beijing University of Chemical Technology, ChinaReviewed by:
Guoliang Yuan, Oak Ridge National Laboratory (DOE), United StatesXinyu Song, Tianjin University, China
Shuaiyin Chen, Zhengzhou University, China
Copyright © 2022 Yu, Wang and Xia. This is an open-access article distributed under the terms of the Creative Commons Attribution License (CC BY). The use, distribution or reproduction in other forums is permitted, provided the original author(s) and the copyright owner(s) are credited and that the original publication in this journal is cited, in accordance with accepted academic practice. No use, distribution or reproduction is permitted which does not comply with these terms.
*Correspondence: Peng-Fei Xia, pfxia@sdu.edu.cn