Urban Grassland Management Implications for Soil C and N Dynamics: A Microbial Perspective
- School of Integrative Plant Science, Cornell University, Ithaca, NY, United States
Urban grasslands are turfgrass dominated landscapes of varying functions and uses that are ubiquitous in areas associated with human population growth and urbanization. While these landscapes are perceived to serve a primarily aesthetic function, they provide a multitude of beneficial ecosystem functions that impervious surfaces do not provide. Urban grassland soils have been shown to accumulate carbon (C) and nitrogen (N) for decades, matching native grasslands and eastern hardwood forest soils in terms of C and N densities. The establishment and maintenance of urban grasslands alters many microbially-mediated biogeochemical processes in soils, including soil organic matter (SOM) dynamics. Despite strong evidence of alterations to soil C and N cycling, the impacts of maintaining urban grasslands on soil microbiomes and their functions remain understudied compared to other ecosystems. Typical management practices can directly and indirectly affect edaphic factors in urban grasslands, which in turn, could impact soil processes mediated by microorganisms. We reviewed the existing literature on urban grassland management, focusing on how mowing, fertilization, irrigation, grass species composition, and soil cultivation could impact the composition and function of soil microorganisms. Although sparse, the literature indicates that the techniques used to maintain urban grassland habitats broadly select for copiotrophic microorganisms adapted to higher resource availability. Additionally, the studies indicate that greater soil fertility and plant productivity found in urban grasslands facilitate the accumulation of soil C and N, as well as SOM as compared to other land-use types. However, effects on soil biology and biogeochemistry depend on specific management practices, which are quite variable. Future research on soil C and N dynamics in urban grasslands should focus on the dominant component of this ecosystem—residential lawns—however, much of the existing scientific literature featuring turfgrass systems focus heavily on golf courses, athletic fields, and major tourist parks.
Introduction
A key feature of urbanization is the expansion of suburban and exurban developments consisting of larger parcel sizes where residential turfgrass lawns are a major land cover type (Robbins and Birkenholtz, 2003; Pouyat et al., 2009; Wang et al., 2017; Ignatieva and Hedblom, 2018). In the United States, where more than 80% of the population lives in urban areas (US Census Bureau, 2011), turfgrass landscapes, including residential, commercial, and institutional lawns, parks, athletic fields, and golf courses, make up roughly 2% of the total terrestrial land area—an area larger than the state of Georgia (Milesi et al., 2005). Moreover, a study on urbanization in Sweden found lawns to be 52% of all urban greenspaces (Hedblom et al., 2017). With more than half of the global population living in urban areas (WHO, 2010), and recent projections that 60% of all urban areas in the world that will exist by 2030 have yet to be built (Secretariat of the Convention on Biological Diversity, 2012), there is an expectation that turfgrass will expand in land area if current development patterns persist.
At a landscape scale, turfgrass forms a contiguous ecosystem that extends beyond individual property boundaries. While humans recognize property boundaries, wildlife, and soil biota are largely free to expand into adjoining parcels, which allows turfgrass to functionally serve as a distinct ecosystem type. Referred to as “urban grasslands,” these ecosystems are comprised of perennial grasses dominated by turf-forming species, grown and managed by humans for aesthetic, recreational, or functional purposes (Groffman et al., 2009; Thompson and Kao-Kniffin, 2017). In this review, the terms “lawns, turf, or turfgrass” are used throughout to refer to urban grasslands. The urban grassland concept includes a range of management intensities from high-end athletic fields and intensively managed golf courses to unirrigated, unfertilized, and infrequently mown roadsides and residential, commercial, institutional, and park lawns or playing fields (Thompson and Kao-Kniffin, 2017).
In recent years, there has been growing recognition that urban grasslands provide additional ecosystem benefits beyond commonly recognized cultural services (e.g., recreation and aesthetics); these include regulating services (e.g., green infrastructure support and soil erosion control), provisioning services (e.g., stormwater and pollution mitigation), and supporting services (e.g., soil formation) (Monteiro, 2017). Intriguingly, urban grasslands have been shown to alter key supporting ecosystem services, such as soil carbon (C) and nitrogen (N) sequestration and soil organic matter (SOM) accumulation. SOM is the largest terrestrial C pool and represents the long-term difference between autotrophic C fixation and heterotrophic respiration of CO2 (Shi et al., 2012; Cotrufo et al., 2015). The amount of C and proportions of C and N entering the stabilized SOM pool are key factors that determine the long-term potential for soil C sequestration and N cycling (Cotrufo et al., 2013). SOM dynamics have long been studied in agricultural settings and in various terrestrial ecosystems, but there has been an increasing interest on SOM and nutrient cycling dynamics in urban areas (Pouyat et al., 2002; Pataki et al., 2011; Smith et al., 2018).
Urban biogeochemistry is complex and differs from native ecosystems in regards to C and N cycling (Kaye et al., 2006; Pickett et al., 2008), and is particularly the case for urban grassland soils (Pouyat et al., 2009; Raciti et al., 2011; Smith et al., 2018). Though variable, turfgrass lawn soils have been found to contain soil organic carbon (SOC) stocks in excess of native shortgrass prairie landscapes (Kaye et al., 2005; Pouyat et al., 2009), agricultural systems (Kaye et al., 2005; Pouyat et al., 2006), and northeastern hardwood forest soils (Pouyat et al., 2002, 2006). Turfgrass was also shown to be highly retentive of N, when applied as a fertilizer (Petrovic, 1990) or received via atmospheric-N deposition (Groffman et al., 2004; Raciti et al., 2008). Empirical and modeling results suggest turfgrass ecosystems can accumulate SOM for 25–40 years or more (Qian and Follett, 2012; Shi et al., 2012). The potential for urban grasslands to store SOC, retain N, or accumulate SOM is dependent on many edaphic and management factors; additionally, the measurement and interpretation of such results depend on the sampling depth and method, therefore soil C and N dynamics of urban grassland soils compared to other landcover types may vary at local or regional scales. Given the wide-spread adoption of urban grasslands and the range of management intensities they receive, understanding the drivers of C and N cycling in these novel environments is of increasing importance.
Soil microorganisms mediate many fundamental belowground ecological processes, such as nutrient cycling and SOM accumulation or depletion, thus even typical urban grassland management practices such as mowing, the return or removal of mowed-off grass clippings, irrigation, fertilization, lawn species composition, or soil disturbance, have the potential to affect soil microbial structure and function. Changes in soil microbial processes may have tremendous influence on long-term C and N dynamics, including determining the fate of SOM in urban grasslands (Shi et al., 2012; Zhalnina et al., 2015; Crouch et al., 2017; Wang et al., 2017). Shifts in the composition of the soil microbiome could signify alterations to microbial functions that regulate biogeochemical transformations and other valuable ecosystem services (Shi et al., 2007, 2012). Despite the complexity and heterogeneous nature of soils, research has shown that soil microbiota respond to changes in environmental conditions and management practices that are at resolutions detectable with standard sequencing approaches (Janssen, 2006; Fierer et al., 2009a; Crouch et al., 2017; Wang et al., 2017). While relative abundances and diversity matrices of broad taxa can be tracked to show microbiome structural changes, soil functional attributes are also detectable (Schimel et al., 2005; Isobe and Ohte, 2014; Reisinger et al., 2016; Wang et al., 2017). Soil environmental controls on microbially-mediated processes, such as substrate availability, redox status, and pH, strongly influence soil microbial dynamics (Isobe and Ohte, 2014; Reisinger et al., 2016; Wang et al., 2017). In urban grasslands, much of these environmental variables are managed, including organic matter levels (mowing, topdressing with compost), soil moisture (irrigation), soil pH (liming), and nutrient additions (fertilizer applications), with potential impacts to soil microbiota and their functions.
In this review, we summarize the distinguishable aspects of urban grasslands, by specifically emphasizing how management practices used in these ecosystems affect soil microbial communities, and consequently, the potential for alterations to soil C and N cycling. Additionally, we address the concept of urban grasslands and management implications on soil SOM, C, and N dynamics in light of emerging models of SOM processing that explicitly emphasize the role of soil microbiota. Finally, we discuss how urban grassland management practices influence soil microbiome structure and function relevant to C and N cycling, and we conclude with a perspective on how advances in urban grassland design and management can optimize a multitude of microbially-mediated ecosystem services.
Characterizing urban grasslands and their management
As defined by Groffman et al. (2009), urban grasslands are “adapted to semi-regular mowing, may be fertilized, may be irrigated, are not grazed, and are kept for aesthetic or recreational value.” This definition constitutes a range of land uses, spatial scales, and interconnectivity, from sports fields or golf courses to residential lawns or roadsides (Figure 1). In all cases, urban grasslands are herbaceous, perennial landscapes of primarily turf-forming grass species, although dominant species composition is regionally adapted (Thompson and Kao-Kniffin, 2017; Trammell et al., 2019). Moreover, different types of urban grasslands experience a wide range of management intensities (Figure 2). A small fraction of turf includes competition-level athletic fields and golf courses that are highly managed with fertilizers, irrigation, pesticides, and intensive cultivation practices. However, the majority of turf landscapes comprise a broad and extensive network of patches encompassing residential and institutional lawns, school playing fields, parks, and right-of-way zones (e.g., turfgrass corridors along sidewalks, roads, electric utility paths, and railways) that are maintained to a lesser degree (Turgeon, 2005; Puhalla et al., 2010; Christians et al., 2016). There is variability in management intensity with lawns, ranging from no-inputs other than mowing, to infrequent or moderate fertilizer, irrigation, and chemical use, to the more intensive professionally-contracted landscape turf management services (Cheng et al., 2008). An in-depth discussion of turfgrass maintenance is provided in Turgeon (2005), Guertal (2012), and Christians et al. (2016), however, this review focuses on the most typical management practices that apply to the majority of urban grasslands—primarily lawns, playing fields, and parks.
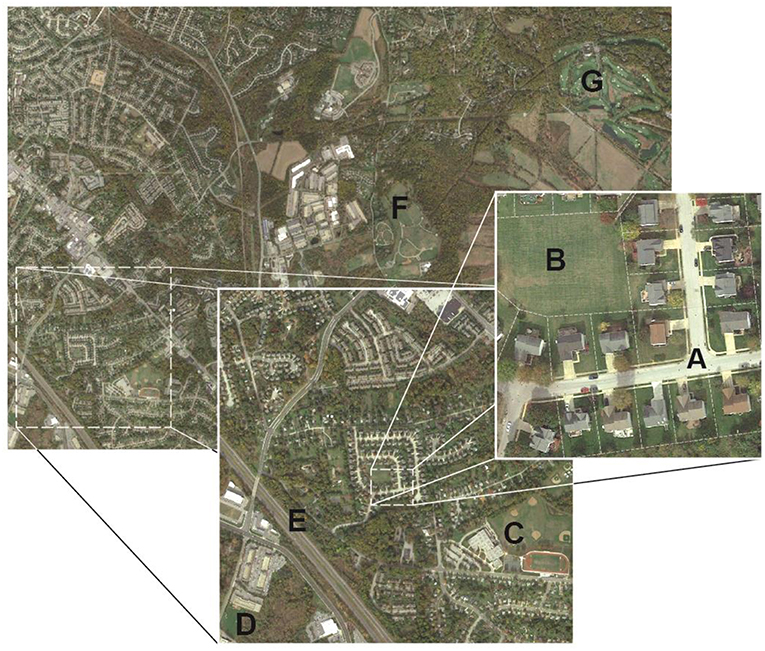
Figure 1. Urban grasslands encompass a range of landscape types, management intensities, and landscape scales including those associated with: (A) residential development, (B) parks and recreational spaces, (C) school athletic fields, (D) institutional campuses, (E) roadsides and medians, (F) civic grounds, and (G) golf courses. While the land-uses and management goals and social drivers of urban grasslands differ, common maintenance practices can have discernable effects on local and regional carbon (C) and nitrogen (N) dynamics and associated soil microbiomes.
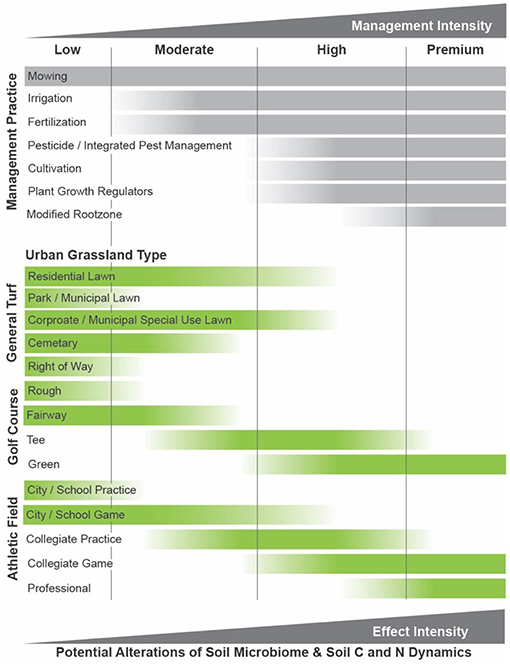
Figure 2. The wide-range of maintenance practices that are used to manage urban grasslands can be grouped into increasing levels of management intensity. They range from low-to-high for most urban grassland types, except for some golf course and athletic fields that require intensive management and inputs. This framework can be used to gauge the potential for alterations to the soil microbiome or soil biogeochemical cycling of different urban grassland types on the basis of management intensity, though more research is needed.
Other characteristics that distinguish urban grasslands from other ecosystem types include ecophysiological traits. For example, urban grasslands photosynthesize, transpire, and assimilate nutrients for a longer portion of the growing season compared to deciduous forests or conventional agricultural systems (Pickett et al., 2008). The long growing season is suggested to be one factor contributing to the high net aboveground productivity observed in urban grasslands (Pouyat et al., 2002; Qian and Follett, 2012). Estimates of turfgrass primary productivity are 1,000 to 1,600 g m−2 yr−1, which is near that of corn or tallgrass prairie systems (Falk, 1980; Shi et al., 2007). Typically, high turfgrass plant densities, combined with dense, fibrous root system architecture, results in relatively large portions of the soil volume to rooting depth being influenced by grass roots (Beard and Green, 1994). The relatively extensive rhizospheres of urban grasslands is also highly dynamic as turfgrass species allocate significant amounts of annual productivity below ground, 2.5–5 times that of cultivated corn or wheat or native grasslands (Kaye et al., 2005). Regular defoliation of turfgrass through mowing increases belowground C allocation, where turf root exudates stimulate rhizosphere microorganisms to mineralize SOM, thereby making nutrients available for turf regrowth after mowing (Hamilton and Frank, 2001). The turfgrass leaf blades removed by mowing, known as clippings, are high in N and rapidly decompose if returned to the soil surface. As much as 20–30% of leaf clippings C and N was mineralized in a 28-day incubation study (Shi et al., 2006). Compared to the return of senesced plant tissue, such as deciduous leaf litter fall, returning fresh grass clippings increases labile C and N availability at the soil surface that could prime the mineralization of SOM near the surface, while frequent mowing additionally stimulates root exudation priming of SOM mineralization. Thus, high productivity, a relatively large and active rhizosphere, and the potential for more frequent return of labile litter suggest that urban grasslands can be highly dynamic systems with rapid components of nutrient cycling (Yao and Shi, 2010; Guertal, 2012).
Soil C and N in turfgrass ecosystems
There is increasing interest in understanding the dynamics of biogeochemical cycles in urban environments, how they differ from other ecosystems, and how human management affects these cycles (Groffman et al., 2004; Kaye et al., 2006; Pickett et al., 2008; Pataki et al., 2011). There is also particular interest in C and N parameters of urban grasslands, including the context of their expansion in regions exhibiting increases in population densities (Milesi et al., 2005; Wang et al., 2014, 2017; Monteiro, 2017; Smith et al., 2018). The scale and rapid rate of land-use change from forests and agriculture to residential landscapes could alter regional C and N dynamics.
There is growing evidence that urban soils can accumulate C at densities equal to or exceeding native and cultivated ecosystems. Pouyat et al. (2002) found that urban areas in the continental United States, on the whole, had higher soil carbon densities than the average for all land-uses in the country (continental 48 states) with 8.2 and 6.8 kg C m−2 at 1 m depth, respectively. Residential areas with substantial lawns had among the greatest pool of soil C (15.5 kg C m−2 at 1 m depth) by land-use, rivaling or exceeding eastern deciduous hardwood forest soils (11.2–16.2 kg C m−2 at 1 m depth; Pouyat et al., 2002). Urban soil C represents about 4.2% of the continental USA soil C pool (2.6 Pg of 61.9 Pg), with residential soils (primarily lawns) holding slightly more than one-third of the urban soil C pool total (0.98 Pg; Pouyat et al., 2002).
Regional variation in climate, native vegetation, and management practices influence if urban grasslands represent an increase or decrease in soil C storage potential compared to regional native ecosystems (Pouyat et al., 2009). For example, turfgrass SOC densities in Baltimore, MD were similar to suburban remnant forest soils (11.0 vs. 11.0 kg C m−2 to 1 m depth) and in Denver, CO, residential turfgrass had greater SOC than native shortgrass prairie soils (12.7 vs. 7.3 kg C m−2 to 1 m depth; Pouyat et al., 2009). The researchers suggested that supplemental irrigation in turfgrass landscapes alleviated water limitations and increased plant production in the Colorado sites. These results and interpretation are consistent with earlier work (Golubiewski, 2006), that compared urban landscapes to native grasslands and agriculture in Colorado. Golubiewski (2006) found SOC increased as urban green spaces aged, such that by 50 years after development, urban sites had significantly more (~2–3 times) SOC to 30 cm depth than either native grasslands or cultivated systems. Despite the difference in sampling depths, these studies present similar conclusions: that turfgrass SOC can rival or exceed native ecosystems, especially when supplemental irrigation and fertilization alleviate constraints on productivity.
Land-use history, in addition to regional climate, has been shown to be a factor in SOC and N accumulation in urban grassland soils. In a study of residential lawn soils with contrasting land-use histories (previously cultivated or hardwood forest), Raciti et al. (2011) found that on average, residential properties contained greater soil C and N densities than nearby reference forests (6.95 vs. 5.44 kg C m−2 and 552 vs. 403 g N m−2, all to 1 m depth). Housing age was a significant predictor of C and N for homes constructed on formerly cultivated lands. They found initially lower C and N densities immediately after residential development on properties that were previously held agricultural lands, but were followed by significant accumulation rates of 0.082 kg C m−2 y−1 (Raciti et al., 2011).
While there is great potential for soil C accumulation in urban grasslands, the activities associated with this ecosystem are dependent on fossil fuels (e.g., mowing, irrigation, and fertilization) that create C-emissions, which offset any gains in soil C storage (Golubiewski, 2006; Guertal, 2012). More fundamentally, a focus on soil C does not account for changes in aboveground C, which can be profound, especially in areas where the native vegetation is hardwood forest. Estimating greenhouse gas emissions for urban grassland maintenance is difficult, but typical lawns can be C-sinks if fertilization is kept to a minimum (−108 g CO2 m−2 y−1 for 10 g N m−2 y−1; Townsend-Small and Czimczik, 2010). Urban grasslands become a C source if they are fertilized at higher rates (+285 g CO2 for 75 g N m−2 y−1), when accounting for emissions from mowing and fertilization against soil C storage (Townsend-Small and Czimczik, 2010). Additionally, the authors found that lawns managed in a way that minimizes SOM accumulation, or those that are frequently disturbed, such as athletic fields, are consistently C-sources (+405 to +798 g CO2 m−2 y−1).
Microbial processing of plant litter into SOM
To better understand how urban grasslands and their management practices affect soil C and N dynamics, it is necessary to consider how plant litter is processed by soil microorganisms into more stable pools of soil C. Here, we attempt to lay a common groundwork for understanding plant-microbe interactions with regards to soil C and N, and how emerging understandings of organic matter decomposition are shifting the emphasis away from a plant-centric view to a more microbially-focused perspective. While much of the research and models focus on natural ecosystems and agriculture, we summarize the extent of plant-microbial interactions and microbial decomposition research most relevant to urban grasslands and the maintenance of this vegetation type.
A major shift is underway in understanding, predicting, and characterizing vegetation litter decomposition dynamics (Grandy and Neff, 2008; Cotrufo et al., 2013; Wieder et al., 2014; Buchkowski et al., 2017; Morrison et al., 2019; Robertson et al., 2019). Emerging concepts are highlighting the role of microbial processing as a controller of the movement of C from plant litter into stabilized SOM pools, in contrast to previously held ideas about litter chemistry being of primary importance. Advances in analytical methods to characterize SOM structure, as well as conceptual advances in linking plant-soil-microbial interactions, are leading the paradigm shift (Grandy and Neff, 2008; Wieder et al., 2014; Cotrufo et al., 2015; Buchkowski et al., 2017; Liang et al., 2017). The improved understanding of microbe-mediated SOM formation could provide important insights for modifying the management of urban grasslands toward greater C sequestration.
Plant litter mass loss studies, in various forms, dominated the decomposition research, with scientists concluding that “recalcitrance” is a function of initial litter chemistry. Specifically, high lignin, phenolic, waxy compound content or high C:N ratios determine the proportion of litter entering soils as particulate inputs are stabilized through microbial processing (Cotrufo et al., 2015). Until recently, it was assumed that soluble, labile, N-rich, and non-structural compounds lost early in decomposition contributed little to SOM (Cotrufo et al., 2013; Liang et al., 2017). However, results from more recent studies challenge these original assumptions. Research involving isotopic tracers of plant litter decomposition (Bird et al., 2008; Rubino et al., 2010), accumulation of microbial decomposition byproducts and microbial necromass in SOM (Mambelli et al., 2011; Liang et al., 2017), and studies showing a lack of preferential accumulation of recalcitrant plant litter components in SOM (Marschner et al., 2008) support the concept of microbially-driven SOM dynamics (Cotrufo et al., 2013; Liang et al., 2017; Robertson et al., 2019). Under the traditional litter decomposition models, turfgrass clippings that contain few structural compounds and higher N content would be expected to contribute little to SOM. However, under a microbially-centered paradigm, the frequent litter return rates of turfgrass leaf blades could contribute more to soil C and N storage than previously thought.
It is now understood that SOM formation is the joint product of litter decomposition and the microbially-mediated enzymatic depolymerization of litter-derived organic polymers and resynthesis into recalcitrant polymers (Grandy and Neff, 2008; Cotrufo et al., 2013; Wieder et al., 2014; Liang et al., 2017). At different levels, SOM is resistant to microbial decomposition by physical protection in soil microaggregates (occlusion), physio-chemical associations with mineral soil fractions (silt and clay, <53 μm), or biochemically stabilized by microbial processing and resynthesis (Six et al., 2002; Grandy and Neff, 2008). Furthermore, microbial residues and exudates, in the form of extracellular enzymes and decaying microbial biomass (necromass), preferentially form mineral-associated SOM (MAOM) pools distinct from plant-derived pools in the particulate soil fraction (>53 μm) (Grandy and Neff, 2008; Wieder et al., 2014). Labile plant residues that are efficiently processed through microbial decomposition can become protected from further decomposition by associations with the mineral soil fraction, which is now considered a primary means by which SOM accumulates. Soil C accumulation in macroaggregates (>250 μm) is another major pathway, although more prone to decomposition if aggregate structure is disrupted by soil disturbance, such as soil grading during construction, tillage, or practices that breakdown soil aggregates (Grandy and Robertson, 2007).
Urban grassland management effects on soil microbiome composition and function
By understanding the contributions of soil microbiota to C and N dynamics, we can assess how different ecosystem properties can be modified to influence SOM formation processes. Alterations to the structural habitat of soil microbiota can impact their composition and function. Similarly, changes to the resource inputs could affect soil microbiota and plant physiological variables—both of which influence SOM processes. In the next section, we tease apart the environmental variables (e.g., mowing and clipping management, fertilization and irrigation, plant species composition, and soil disturbance) that are modified in urban grasslands and discuss their potential impacts on soil microbiota, as summarized in Table 1.
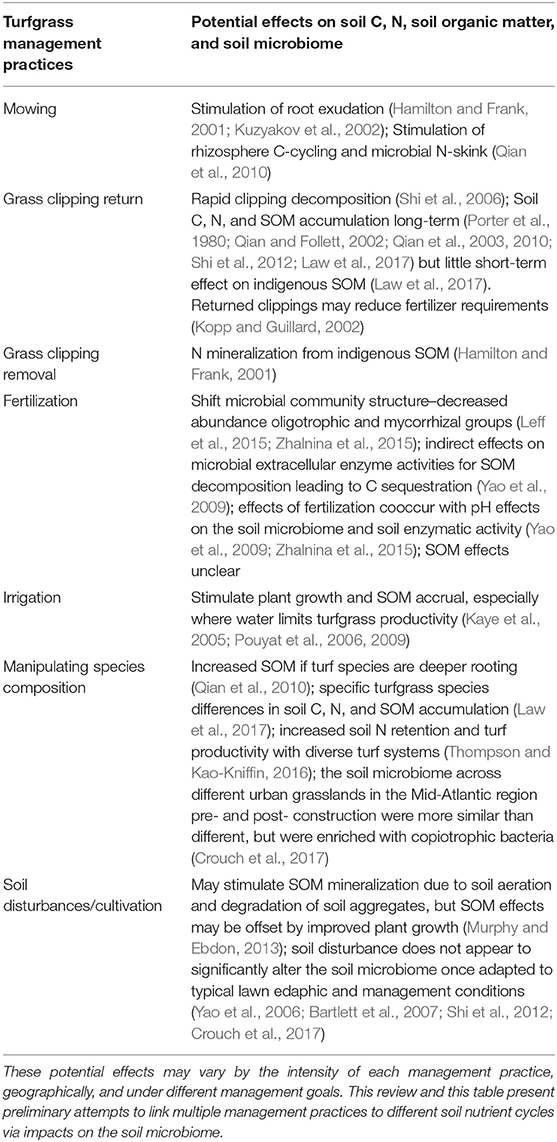
Table 1. Common turfgrass management practices their effects on soil C, N, soil organic matter (SOM), and the soil microbiome.
Mowing and Clipping Management
Regular defoliation by mowing is among the most fundamental turfgrass maintenance practices, akin to ungulate grazing in natural grasslands. Mowing serves to maintain a usable groundcover surface, reduce weedy encroachment of species intolerant of mowing, and sustaining the turf (Turgeon, 2005). Clipping management (i.e., return or removal of mown off plant material) is a major factor controlling microbial growth and activity and nutrient cycling in urban grasslands (Figure 3).
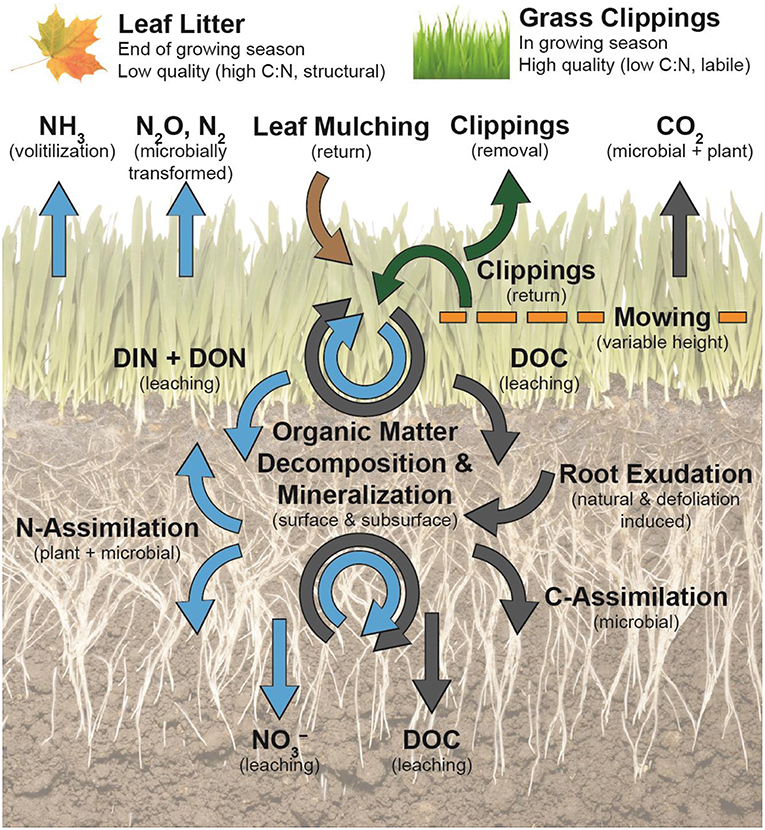
Figure 3. Turfgrass litter management practices affect dominant C (gray arrows) and N (blue arrows) flows and soil organic matter dynamics at the soil surface and subsurface. Defoliation by mowing stimulates turfgrass root exudation, which supplies the soil microbiome with labile C and creates a microbial N-sink. Microbially mineralized N can be assimilated by microorganisms or turfgrass roots, leached, or lost via gaseous pathways. Grass clippings (green arrows) are more labile forms of litter in comparison to senesced urban tree leaves and will accelerate decomposition and mineralization at the soil surface and in the thatch layer, if returned. Leached labile C and N compounds from decomposing clippings at the soil surface can contribute to rhizosphere N demands. Removal of grass clippings take away C and N. Leaf litter mulching (brown arrow) is more recalcitrant, contains less N, and is more resistant to microbial degradation. The effect of leaf litter residue on soil C and N in lawns is less understood.
Hamilton and Frank (2001) studied the effect of clipping removal on rhizosphere plant-microbial feedbacks in unmanaged grasslands that was dominated by Kentucky bluegrass (Poa pratensis L.), a common cool-season turfgrass species. While the authors intended to simulate ungulate grazing in Yellowstone National Park, this study is informative of processes that occur in low maintenance lawns (unfertilized and receiving no supplemental irrigation) where clippings are not returned after mowing. By clipping P. pratensis and removing the clippings, Hamilton and Frank (2001) stimulated root C exudation that was assimilated by rhizosphere microorganisms, increasing microbial biomass C. In clipped plots with more microbial biomass, the authors observed an increase in potential N mineralization that made inorganic soil N (-N and -N) more available in the soil, which corresponded to greater net photosynthesis and shoot N content of P. pratensis a week after clipping. Defoliated grasses simulated soil microbes through root exudates to mineralize indigenous SOM to supply N for turf regrowth (Hamilton and Frank, 2001).
Law et al. (2017) compared the effects of clipping return or removal on labile soil C, total soil C, SOM, and total N accumulation in field plots of Kentucky bluegrass (P. pratensis L.) and tall fescue [Schedonorus arundinaceus (Schreb.) Dumort. syn. Festuca arundinacea Schreb. syn. Lolium arundinaceum (Schreb.) Darbysh]. The authors found that after 2 years of clipping treatments, returned clippings contained 3.3% (826 vs. 800 mg C kg−1 soil) more labile C, 3.3% more total soil C (24.7 vs. 23.9 g C kg-−1 soil), and 4.6% total soil N (2.28 vs. 2.18 g N kg−1 soil), but SOM was unaffected. Law et al. (2017) reported that their experimental results of total soil C and N accumulation closely followed results predicted by the CENTURY model, which simulates C and N accumulation from clipping return (Qian et al., 2003). They suggested that with more time, changes to the SOM pool from clipping management practices may be observed, but that clipping return resulted in significant soil C and N accumulation within just 2 years (Law et al., 2017).
The interactions of clipping management and fertilizer application rates was studied in mixed Kentucky bluegrass (P. pratensis L.), creeping red fescue (Festuca rubra L.), and perennial ryegrass (Lolium perenne L.) turfgrass lawn plots by Kopp and Guillard (2002). Clipping return or removal was compared across four fertilizer application levels (0, 98, 196, or 392 kg N ha−1 yr−1). Returning clippings increased turfgrass dry matter yields by 30–72%, total N uptake (NUP) by 48–60%, and N use efficiency (NUE) by 52–71%. The authors concluded that returning turfgrass clippings had no negative effects on turf quality and could reduce N fertilization rates by 50% or more (Kopp and Guillard, 2002). While this study did not explicitly study soil microorganisms, it can be inferred that microbial N mineralization of returned turfgrass clippings supplied additional N that resulted in greater turf growth. The benefits of clipping return were seen across the fertility treatments, which suggests that regardless of the level of management an urban grassland receives in terms of supplemental fertilization, clipping return would be beneficial.
In a study of a chronosequence of golf course fairway soils, Shi et al. (2006) found that turfgrass clippings decomposed rapidly, with 20–30% of C and N from clippings mineralized over a 28-day incubation. The fairways received N applications of approximately 150 kg N ha−1 yr−1 (Shi et al., 2006). While golf course age was not correlated with decomposition or net N mineralization, the potential nitrification rate was significantly different in younger vs. older fairway soils. Young soils exhibited an initially low potential nitrification rate that increased after clipping addition, whereas old turf soils had an initially high potential nitrification rate that was unaffected by the clippings (Shi et al., 2006). Following clipping addition, potential nitrification rates were similar despite soil age. The authors concluded that returning grass clippings stimulated rapid decomposition of the clippings (~30% within 7 days), but not mineralization of indigenous SOM. Additionally, they found that turfgrass age was not a factor, thus mowing practices with clipping return would have similar effects despite turf age.
Together, these studies indicate the importance of turfgrass defoliation (mowing) and clipping management to SOM dynamics, particularly N cycling (Hamilton and Frank, 2001; Kopp and Guillard, 2002; Kuzyakov et al., 2002; Shi et al., 2006). Defoliation by mowing stimulates rhizosphere C-cycling, creating a microbial N-sink (Qian et al., 2010) and stimulating microbial mineralization to meet the N demand. Turfgrass roots can intercept some of the mineralized N. If clippings are returned to the soil surface, they are rapidly decomposed and dissolved labile C and N from the clippings can leach into the soil to meet the microbial and root N demand. Alternatively, clipping removal can stimulate the mineralization of indigenous SOM (Hamilton and Frank, 2001) to meet microbial and plant N needs. Returning grass clippings reduces the need for N fertilization by up to 50% without compromising turfgrass quality (Kopp and Guillard, 2002) and protects SOM from microbial mining, allowing SOM and soil C to accumulate for a 20–40 year period following turfgrass establishment (Qian and Follett, 2002; Qian et al., 2010; Shi et al., 2012). Concurrently with SOM accumulation, N has been found to accumulate in turfgrass soils with clipping and fertilizer additions for more than a decade after establishment (Porter et al., 1980). Furthermore, simulation modeling studies suggest that properly managed turfgrass can be an N-sink for at least 20–30 years (Qian et al., 2003).
Fertilization and Irrigation
Supplemental fertilization and irrigation are common maintenance practices for many urban grassland types (Figure 2), especially those maintained to moderate and higher degrees, and can affect soil microbiome composition and nutrient cycling. Multiple studies have shown that urban grassland irrigation in arid or semiarid regions leads to enhanced C cycling and altered regional soil C dynamics since irrigation alleviates water limitations (Kaye et al., 2005; Pouyat et al., 2006, 2009). Fertilization has been shown to indirectly affect microbiome structure in native grasslands through plant-community mediated responses (Leff et al., 2015) and in lawns through increased fertility. The indirect effects of fertilization also impact soil microbiota, as fertilizer sources can lower soil pH through soil acidification (Zhalnina et al., 2015). Additionally, indirect effects were reported for microbial extracellular enzyme activities in response to fertilization (Yao et al., 2009).
Kaye et al. (2005) found that fertilized and irrigated lawns in Colorado, USA had 4–5 times greater aboveground net primary productivity (ANPP) and 2.5–5 times greater total belowground C allocation than unmanaged shortgrass steppe. Phospholipid fatty acid (PLFA) analysis of microbiome structure showed that irrigated and fertilized urban lawn microbial biomass was twice that of fallow-wheat, irrigated corn, or unmanaged shortgrass steppe, but the relative abundance of broad soil microbial taxonomic groups was not consistently different across land-use types (Kaye et al., 2005).
Since there are still relatively few studies that explicitly focus on urban grassland soil microbiomes, it is important to understand microbial dynamics in natural grasslands. In a study of 25 globally-distributed native grasslands, Leff et al. (2015) found consistent shifts in microbiome composition with N and P additions, and that the magnitude of the compositional shift was proportional to the plant response to the nutrient input. Nutrient additions suppressed mycorrhizal fungi (Glomeromycota), oligotrophic bacteria adapted to low-fertility conditions (Acidobacteria), and methanogenic archaea (Euryarchaeota and candidate division [Parvarchaeota]). Leff et al. (2015) concluded that nutrient additions put the suppressed microbial groups at competitive disadvantages where their ecological adaptations for resource acquisition (mycorrhizal fungi) or N2 fixation (Euryarcheota) were not as valuable under non-resource limiting conditions. They noted that copiotrophic organisms (fungi: Ascomycotabacteria; archaea: Crenarchaeota; bacteria: Actinobacteria and Alphaproteobacteria) with fast growth rates were more competitive and thus more abundant in the sampled grassland soils. The authors pointed out that the reduced average bacterial genome size, gathered from shotgun metagenomic analysis, was suggestive of chronic fertilization enriching for fast-growing, copiotrophic microbial communities over time. Larger microbial genomes have been associated with more success in resource poor environments (Konstantinidis and Tiedje, 2004; Leff et al., 2015) and smaller average genomes reduce functional gene abundance and may have implications for nutrient cycling (Wieder et al., 2013; Leff et al., 2015) under fertilization.
Direct and indirect fertilization effects on turfgrass soil microbiome was explored by Zhalnina et al. (2015) with the Park Grass experiment (PGE) at Rothhamsted Research in the UK. The PGE has been ongoing since 1856 and is to study the long-term effects of how fertilizers affect plant productivity. It is the longest-running plant ecology experiment in the world (Crawley et al., 2005). Over the more than 150 years since the study was initiated, fertilization has resulted in soil acidification from ammonium sulfate [(NH4)2SO4] or sodium nitrate (NH4NO3) (Zhalnina et al., 2015). Turfgrass species richness in the plots declined with soil pH and was further reduced when N and P fertilizers were used in combination (Crawley et al., 2005). Zhalnina et al. (2015) found using 16S rRNA sequencing techniques that soil pH was the primary driver of soil microbiome composition, diversity, and biomass within the PGE. The lowest microbial diversity was found in plots with low pH, receiving (NH4)2SO4. Overall, following the oligotrophic-copiotrophic continuum of organisms, respectively, adapted to low and high resource environments (Fierer et al., 2007; Leff et al., 2015), oligotrophic bacteria (e.g., Verrucomicrobia and Chloroflexi) were negatively correlated with N fertilization treatments. The abundance of nitrifying Thaumarchaeota and Nitrospirae were positively correlated with -N and -N. Phylum- and genus-level microbial community composition was also altered by soil pH (Zhalnina et al., 2015). The Ca. Solidobacter (Acidobacteria) and Clostridium (Firmicutes) are bacteria with preferences for acidic conditions, and they were found in low pH plots. The Rothamsted Park Green experiment study demonstrates that long-term urban grassland management with different formulations of N fertilizers, in conjunction with P fertilizers, and the indirect effects of soil acidification can affect the composition of the soil microbiome, including the abundance of organisms with functional roles in N cycling.
Kuzyakov et al. (2002) found that cut perennial ryegrass (Lolium perenne L.) decreased belowground C losses (root respiration and exudation) during regrowth when fertilizer was added, further suggesting that when an alternative N source is available (e.g., fertilization or clipping return), that cut turfgrasses may rely less on simulating microbial mineralization of indigenous SOM to supply N for regrowth. Microbially-produced soil extracellular enzymes catalyze rate-limiting steps in SOM decomposition (Sinsabaugh et al., 2008; Fanin et al., 2016), therefore nutrient additions that affect microbiome structure or enzymatic activity can affect soil C dynamics in human managed ecosystems. It is generally expected that increased N availability will enhance decomposition of litter-derived cellulose by hydrolytic enzyme activity, but diminish oxidative degradation of lignified or complex compounds (Sinsabaugh, 2010; Grandy et al., 2013). Although uncertainties remain regarding net soil C stocks in response to N fertilization as plant-residues are increased concurrently with enhanced decomposition (Grandy et al., 2013). When comparing two turfgrass chronosequences, fertilization (60 mg N kg−1 soil) induced minor negative effects on soil oxidase and hydrolytic enzymatic activity (Yao et al., 2009), leading to C sequestration. Consistent with previous reports (Sinsabaugh et al., 2008), Yao et al. (2009) found soil pH in turfgrass ecosystems to be another important control on soil enzymatic activity. Under alkaline conditions, Yao et al. (2009) found a two-fold increase in phenol oxidase activity correlated with a two-fold increase in soluble phenolic compounds and a decrease in hydrolytic enzyme activities, resulting in C accumulation in alkaline soils. The authors concluded that soil pH had a strong influence on enzymatic activity but that, consistent with agroecosystems, N fertilization and litter return enhances soil C inputs (Yao et al., 2009). High quality turfgrass litter [e.g., C:N ratio of ~10.8 (Yao et al., 2009)] are below reported thresholds [e.g., <15 C:N (Craine et al., 2007)] where N fertilization has little impact on recalcitrant plant residues (Shi et al., 2012).
Since fertilizer sources and rates can be modified easily in urban grasslands, it is important to understand their effects on soil C and N. The relative strength of N or pH controls on enzymatic activity, and thus SOM degradation within turfgrass ecosystems is complex. However, multiple studies indicate that fertilization directly and indirectly (through acidification) affects plant productivity, soil microbiota, and the activities of microbially-produced extracellular enzymes that facilitate soil biogeochemical nutrient cycling.
Plant Species Composition
Soil C accumulation in urban grasslands occurs primarily within the upper 10–20 cm of soil, where root density is the greatest (Qian et al., 2010), though significant soil C has been observed to over 70 cm (Raciti et al., 2011). Turfgrasses allocate substantial amounts of C (~2.5–5 times more than agroecosystems or native shortgrass prairies) to belowground tissues (Kaye et al., 2005). As microbial biomass and activity increases with SOM content (Shi et al., 2007), increasing the depth of root growth and rhizosphere activity may be one means to increasing SOC accumulation in urban grasslands. Limited research on turfgrass species rooting depth supports this, indicating increasing C accumulation with depth associated with deeper rooting fine-fescue species (Qian et al., 2010) and tall fescues (Law et al., 2017). The study by Law et al. (2017), mentioned previously as a clipping return study, also contrasted turfgrass species effects (Kentucky bluegrass vs. tall fescue) on soil C and N accumulation. They found that tall fescue, after <3 years of growth, increased labile soil C by 9.9% (851 vs. 774 mg C kg−1 soil), elevated total soil C by 4.2% (24.8 vs. 23.8 g C kg−1 soil) and raised SOM by 8% (41.7 vs. 38.6 SOM kg−1 soil) compared to Kentucky bluegrass. While Law et al. (2017) did not consider the soil microbiome, clear turfgrass plant species differences in soil C and N cycling suggest that there were effects on microbial variables.
Increasing turfgrass diversity from monocultures to 3-, 6-, and 12-part polycultures of commonly used turfgrass species and cultivars has been shown to increase turfgrass productivity (verdure and clippings), aid in N retention, and increase soil bacterial and fungal diversity (Thompson and Kao-Kniffin, 2016). The study was not designed to test long-term soil C or N accumulation, but the experimental was designed to explicitly manipulate turfgrass species composition diversity in relation to soil microbiome outcomes. While it is only one study, the results do parallel findings of a decade-long study in perennial grassland biodiversity-ecosystem function (BEF) research that suggests increasing plant species diversity will enhance root biomass, soil carbon, and N retention (Pasari et al., 2013). Linkages between above and belowground diversity were determined by Thompson and Kao-Kniffin (2016) using terminal restriction fragment length polymorphism (T-RFLP) community fingerprinting techniques. When Zhalnina et al. (2015) used next-generation sequencing techniques, which generates more detailed taxonomic data than community fingerprinting, they found that plant species richness and plant productivity were not significantly related to microbial diversity.
When the lawn of the US National Mall in Washington, DC was renovated with new sod (a 50/50 mixed-blend of Festuca arundinaceae Schreb. [syn. Schedonorus phoenix (Scop.)] and P. pratensis L., the soil microbiome was compared pre- and post-renovation with the originating sod farm (Crouch et al., 2017). Bacterial 16S rRNA sequencing revealed that across all four lawns in the study, the Gram-negative, Proteobacteria phylum was 52.2–58.6% of the observed sequences, of which the genus Rhodoplanes was widely abundant (26.4–37.3%). Rhodoplanes has been shown to be abundant in other turfgrass lawns and golf course greens (Zhalnina et al., 2015; Beirn et al., 2017; Crouch et al., 2017). While Rhodoplanes are known denitrifiers, the importance of these organisms in natural systems and their ecological role is not well-understood (Crouch et al., 2017). The authors concluded that despite pre- and post- lawn reconstruction differences and geographic differences of lawns within the Mid Atlantic coast, the lawn microbiomes were more similar than different (Crouch et al., 2017). With reference to the soil microbiome copiotrophic-oligotrophic continuum (Leff et al., 2015), the lawns sampled by Crouch et al. (2017) were a majority Proteobacteia and Actinobacteria (collectively 84.2–88.3% of all operational taxonomic units: OTUs), indicating the effects of higher nutrient conditions in shaping the lawn soil microbiome.
The results suggest that turfgrass composition replacement did not impose significant perturbations to alter the soil microbiome compared with the more significant effects observed by Zhalnina et al. (2015), that resulted from long-term fertility and acidification effects in the Park Grass experiment. However, as noted by Yao et al. (2006), Bartlett et al. (2007), and Shi et al. (2012), microbiome shifts may be more significant during land-use change than over subsequent management of a landscape. The Crouch et al. (2017) study did not include soil physiochemical parameters that could be correlated with community composition data, nor did it measure mineralization, plant growth, or other ecological functions that are important considerations of soil microbiome research to link composition to function. Groffman et al. (1996) suggested that the potential for grass species compositional changes alone to affect microbial biomass will be secondary to soil factors (e.g., pH and texture) and dependent on differences in plant-derived labile C inputs. Although Groffman et al. (1996) was not considering microbiome compositional effects of grass species diversity, the magnitude of soil physiochemistry in shaping the soil microbiome is becoming increasingly understood as a primary controller of microbial species presence and abundance (Fierer et al., 2009b).
To explore N budgets of southern California lawns, Wang et al. (2014) studied regionally adapted lawn species under different management intensities: a “typical” cool-season (C3 photosynthesis) tall fescue [Schedonorus phoenix (Scop.), syn. Festuca arundinacea Schreb.] irrigated and fertilized at 192 kg−1 ha−1 yr−1; a “low input” warm-season (C4 photosynthesis) seashore paspalum (Paspalum vaginatum Sw.) irrigated and fertilized at 123 kg−1 ha−1 yr−1; and a local best-practice “low impact” lawn of native sedge (Carex spp.) species irrigated and fertilized at 48 kg−1 ha−1 yr−1. Wang et al. (2014) concluded that reducing fertilizer applications could reduce denitrification in the low input lawns and that lawns composed of warm-season C4 grasses could reduce irrigation and fertilization needs while still maintaining high productivity and N retention. The proportion of C3/C4 grasses present in lawns across the United States is a function of the mean annual temperature of the region, elevated urban temperatures due to heat island effects, elevated CO2 and lawn management dynamics (Bijoor et al., 2008; Trammell et al., 2019). More research is needed to determine how the C3/C4 composition of urban grasslands affects soil microbiome composition and function as it relates to urban biogeochemical processes.
Explicitly manipulating plant species composition in urban grasslands should be studied as potential ways to increase SOC and N storage potential in urban grasslands. Intentionally selecting individual turfgrass species can increase soil C and N accumulation (Law et al., 2017); increasing urban grassland plant species diversity simultaneously alters N dynamics and belowground microbial diversity (Thompson and Kao-Kniffin, 2016); and taking advantage of alternate turfgrass photosynthetic pathways affects productivity and N retention under certain environmental conditions (Wang et al., 2014). Plant species effects are likely to be small relative to differences induced by management practices that directly or indirectly edaphic factors affecting water, nutrient, and oxygen availability for soil microorganisms (Groffman et al., 1996), but further research is needed to explore how such plant species compositional changes directly or indirectly affect the soil microbiome.
Soil Disturbance
A majority of urban grasslands are long-term perennial ecosystems that experience relatively little soil disturbance (Guertal, 2012) compared to other human-managed ecosystems (e.g., conventional agricultural tillage). For turfgrass management, major soil disturbance involving tillage methods may occur infrequently through periodic renovations of damaged or compacted turf. More routine soil disturbance that may occur every 1–3 years in moderately managed systems is compaction alleviation involving equipment that pierces through the soil (e.g., tines or core aerifiers). Altering soil structure could impact soil microbiota in many ways, including changes to pore spaces, gas exchange, water infiltration, root proliferation, and organic matter decomposition.
Urban development frequently results in significant soil disturbance that includes the degradation of soil structure, mixing of soil layers, and removal of entire horizons using large mechanical equipment, especially for newly-constructed sites or those experiencing redevelopment (Turgeon, 2005). These practices result in varying degrees of disruption to soil structure depending on the intensity of grading practices. Rough and fine grading practices can disrupt soil peds, macro-, and microaggregates, which can alter soil pore spaces and expose occluded SOM to heterotopic decomposition (Baker et al., 2007). Turfgrass soil microbiota have been shown to change primarily at the time of turf establishment (i.e., land-use change) and remain stable over the course of many decades, despite typical turfgrass management techniques (Yao et al., 2006; Bartlett et al., 2007; Shi et al., 2012) or during lawn renovation via sod replacement (Crouch et al., 2017).
With few exceptions, nearly all turfgrass ecosystems experience some form of compaction resulting from foot or light vehicular traffic. Depending on the intended use, turfgrass may experience even more frequent compaction and wear from dedicated sports field activities, to recreational activities in small residential lawns, and for overflow event parking (Murphy and Ebdon, 2013). Soil compaction in urban grasslands is particularly a concern where loads are applied to fine-textured soils or repeated traffic is applied to the same areas (Murphy and Ebdon, 2013). In all cases, compaction itself is a soil disturbance of varying intensity and can indirectly affect soil microbial communities by decreasing water infiltration, percolation, restricting soil gas exchange, and if soil density is great enough, even inhibiting root growth and soil fauna movement (Zabinski and Gannon, 1997). Soil compaction can be measured as surface hardness, the resistance a soil penetromer experiences while being pushed into the soil, or as overall bulk density. Even surface hardening within the upper few cm of a soil can affect water and gas dynamics deeper in the soil (Murphy and Ebdon, 2013). Common turfgrass cultivation techniques involving the use of tines, core aerification, or vertical mowing to alleviate soil compaction, disturb relatively little of the soil profile compared to conventional agricultural tillage techniques (Turgeon, 2005; Guertal, 2012). Specialized techniques used to maintain sports and putting green turf such as sand topdressing, rolling, other sports turf-specific cultivation techniques, and entirely sand-based soil profiles for golf greens are beyond the scope of this review, but can be found in Stier et al. (2013). While the majority of turfgrass literature covering cultivation techniques focuses on athletic fields and golf course settings, they do not focus on soil biota or biogeochemical processes (Murphy and Ebdon, 2013). Soil compaction may not be a primary concern for most turf systems beyond sports fields and golf courses, but long-term compaction, even on residential sites, has the potential to alter edaphic conditions that affect C and N transformations within the soil.
Future Directions in Urban Grassland Management and Implications on C and N Dynamics
We summarized in this review that urban grasslands are a dominant component of human settlements across the United States and in many parts of the world. Adoption and maintenance of this land-use has implications for regional C and N budgets through impacts on microbially-mediated C and N transformations. Urban grasslands have been shown to accumulate soil C, and retain N for decades after establishment. There is great variability in regional climates that affect urban grassland plant species composition (Trammell et al., 2019), productivity and nutrient retention and accumulation (Qian and Follett, 2012; Smith et al., 2018), and the need for supplemental inputs to maintain urban grassland quality. The management intensity of urban grasslands also varies as a function of intended use, of numerous social-ecological factors (Cook et al., 2012), and of personal preference by property owners and managers. Microbiome research specific to urban grasslands is increasing, but the few published studies indicate a general shift toward copiotrophic taxa consistent with increasing nutrient availability in urban grasslands (Zhalnina et al., 2015; Crouch et al., 2017; Wang et al., 2017). The change in microbial composition may be a result of both turf fertilization and the observed increase in C and N cycling, even in low-input turfgrass plots.
Research linking turf management practices to microbial mechanisms of litter decomposition and SOM accumulation or depletion is still limited. To date, empirical data for turfgrass ecosystems is primarily drawn from golf courses, with relatively less research on turfgrass soil ecology in residential lawns and other non-sports dedicated turf, which cover a greater area and thus have the potential for greater regional impacts than golf courses. Much research has focused either on soil C or plant-centric measures of turf growth and quality, with much less attention paid to microbially-mediated ecosystem services or plant-microbial interactions within urban grasslands. Coupled biogeochemical and human behavioral studies in residential landscapes are emerging, but urban grasslands are still underrepresented in terrestrial ecosystem research as a whole. Advanced high-throughput sequencing techniques, along with metagenomic or other “-omic” techniques, in conjunction with microbial biomarker techniques (e.g., PLFA or FAME) for classifying soil microorganisms are needed to more directly link microbiome composition to functional capacities that affect ecosystem services in urban ecosystems.
In developing this review, the authors have identified the following three areas for additional research to link urban grassland management explicitly with the composition and function of the soil microbiome: (1) more studies are needed in residential and non-sports-related urban grasslands to elucidate management effects, since non-sports dedicated turf comprises the majority of this ecosystem type; (2) a greater regional focus on urban grasslands is needed to address the appropriateness of turfgrass as a groundcover, particularly as more arid regions are adopting xeric landscaping or synthetic turf; and (3) improved integration of biogeochemical and plant-soil-microbial measurements to link with turfgrass management for improved ecosystem services that go beyond aesthetics and recreation. Likewise, it cannot be ignored that there are critical social factors at play in the management decisions for urban grasslands; therefore, future research should continue to attempt to integrate and synthesize the socioeconomic and biophysical outcomes for urban landscapes (Groffman et al., 2004).
Looking forward, urban grassland management is also expected to change in the coming years as environmental regulations for fertilizers, pesticides, and stormwater mitigation are passed, as irrigation water is restricted, as environmental sensors become more widely used, and as resource conserving technologies gain mainstream adoption (Figure 4). Emerging environmental regulations are restricting when fertilizers may be applied and limiting phosphorous applications (Maryland, 2018). Moreover, some states are moving toward limitations on pesticides in turfgrass systems, particularly for areas where children are in contact with turf (Department of Environmental Conservation, 2010; Department of Energy Environmental Protection, 2012). Commercial irrigation systems have relied on weather stations and turfgrass evapotranspiration models to supply water based on actual conditions instead of regularly scheduled applications, particularly on larger scale systems. Model water-efficient landscape ordinances are stipulating stricter controls on irrigation systems, including determinations on which areas should be irrigated and making moves to limit high water demand plantings—all of which will alter how urban grasslands are implemented and managed in the future (California Department of Water Resources, 2018). The evaluation of in-ground wireless temperature and moisture sensors at landscape level networks is proving useful for ecosystem research and has potential in industry to further water use efficiency (Szlavecz et al., 2007; Terzis et al., 2010). Lastly, battery-powered autonomous mowing robots have been found to conserve energy and improve turf quality compared to traditional gasoline-powered rotary mowers (Grossi et al., 2016). Human-guided or autonomous electric mowers, including solar-powered models, have the potential to minimize carbon emissions from urban grasslands, if adopted at wide-scale. As this review illustrates, current and potential changes for urban grassland management that are on the horizon may all have direct and indirect implications for the soil microbiome and consequently belowground biogeochemistry in urban grasslands. Many of these policy and technological changes to turfgrass landscapes could alter resource inputs to soil microbiota. For example, significant changes to water and fertilizer use in these ecosystems can impact ecophysiological components of the vegetation alter impact litter chemistry and microbial decomposition of litter.
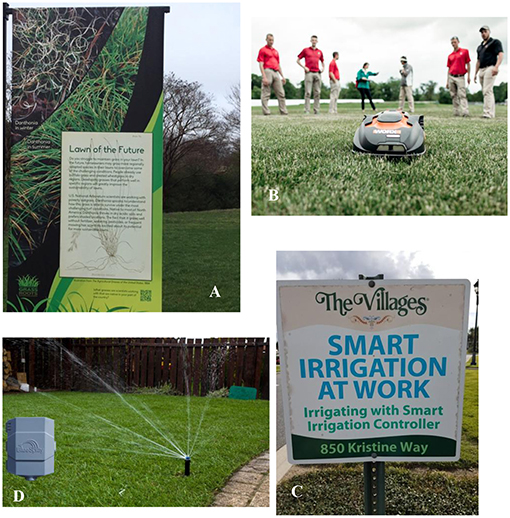
Figure 4. The future of urban grasslands in the U.S. is rapidly changing with adoption of resource conserving technologies and environmental regulatory practices. Examples of changing practices aimed to promote more sustainable management of urban grasslands: (A) development of new lawn cultivars that are drought tolerant and require less maintenance, sign displayed at the Grass Roots Initiative exhibit at the National Arboretum in Washington DC; (B) autonomous, battery-powered lawn mower evaluations at the Cornell University Turf and Landscape Research and Extension Center in Ithaca, NY; (C) signage explaining the Smart Irrigation System installed in an expanding residential community in Florida; and (D) wireless, sensor-based irrigation network. Photos by: (A) Jenny Kao-Kniffin, (B) Justin James Muir, courtesy of Cornell CALS, (C) Shirley Kniffin, and (D) BlueSpray, Avidz LLC. Written consent was obtained for all photo images.
Collectively, rapid changes in turfgrass management that are driven by regulatory action and market forces will alter the scale and composition of urban grasslands and the soil microorganisms that mediate C and N cycling processes in these systems. These changes to the management practices of urban grasslands could enhance the sequestration potential of C and N in soils, in addition to decreasing the emissions of C. However, there is a need for more research on soil microorganisms in urban grasslands, especially given the potential to modify environmental factors that influence long-term sequestration of soil C and N. As this review seeks to illustrate, common urban grassland management strategies like mowing, clipping return, aerification, fertilization, and vegetation replacement can have direct and indirect effects on the structure of soil microbiota and their functions, with the ultimate effect of altering soil biogeochemical cycles at localized and regional scales. As management practices advance or become more regulated, there is a continued need to measure the impact of land management practices on soil microbial communities and ecosystem function.
Author Contributions
This article was written by GT and JK-K, with revisions by both authors.
Funding
This article was supported financially by the National Science Foundation (NSF) IGERT—From Microbe to Global Climate: Research and Training in Cross-scale Biogeochemistry and Climate (#1069193) and the NSF Coupled Natural and Human Dynamics Program (#1615560).
Conflict of Interest Statement
The authors declare that the research was conducted in the absence of any commercial or financial relationships that could be construed as a potential conflict of interest.
Acknowledgments
The authors thank Dr. Peter M. Groffman and Dr. Kyle G. Wickings for editorial comments on the draft manuscript, and for photos by Justin James Muir (courtesy of Cornell College of Agriculture and Life Sciences), Shirley Kniffin, and Dr. Frank Rossi's turfgrass program. The authors thank the National Science Foundation for supporting the research.
References
Baker, J. M., Ochsner, T. E., Venterea, R. T., and Griffis, T. J. (2007). Tillage and soil carbon sequestration—what do we really know? Agr. Ecosyst. Environ. 118, 1–5. doi: 10.1016/j.agee.2006.05.014
Bartlett, M. D., James, I. T., Harris, J. A., and Ritz, K. (2007). Interactions between microbial community structure and the soil environment found on golf courses. Soil Biol. Biochem. 39, 1533–1541. doi: 10.1016/j.soilbio.2007.01.003
Beard, J. B., and Green, R. L. (1994). The role of turfgrasses in environmental protection and their benefits to humans. J. Environ. Qual. 23, 452–460. doi: 10.2134/jeq1994.00472425002300030007x
Beirn, L. A., Hempfling, J. W., Schmid, C. J., Murphy, J. A., Clarke, B. B., and Crouch, J. A. (2017). Differences among soil-inhabiting microbial communities in Poa annua turf throughout the growing season. Crop Sci. 57(suppl. 1), S-262–S-273. doi: 10.2135/cropsci2016.06.0463
Bijoor, N. S., Czimczik, C. I., Pataki, D. E., and Billings, S. A. (2008). Effects of temperature and fertilization on nitrogen cycling and community composition of an urban lawn. Glob. Change Biol. 14, 2119–2131. doi: 10.1111/j.1365-2486.2008.01617.x
Bird, J. A., Kleber, M., and Torn, M. S. (2008). 13C and 15N stabilization dynamics in soil organic matter fractions during needle and fine root decomposition. Organ. Geochem. 39, 465–477. doi: 10.1016/j.orggeochem.2007.12.003
Buchkowski, R. W., Bradford, M. A., Grandy, A. S., Schmitz, O. J., and Wieder, W. R. (2017). Applying population and community ecology theory to advance understanding of belowground biogeochemistry. Ecol. Lett. 20, 231–245. doi: 10.1111/ele.12712
California Department of Water Resources (2018). Model Water Efficient Landscape Ordinance. Available online at: https://water.ca.gov/Programs/Water-Use-And-Efficiency/Urban-Water-Use-Efficiency/Model-Water-Efficient-Landscape-Ordinance (accessed August 16, 2018).
Cheng, Z., Richmond, D. S., Salminen, S. O., and Grewal, P. S. (2008). Ecology of urban lawns under three common management programs. Urban Ecosyst. 11, 177–195. doi: 10.1007/s11252-008-0048-9
Christians, N. E., Patton, A. J., and Law, Q. D. (2016). Fundamentals of Turfgrass Management. Hoboken, NJ: John Wiley & Sons, Inc. doi: 10.1002/9781119308867
Cook, E. M., Hall, S. J., and Larson, K. L. (2012). Residential landscapes as social-ecological systems: a synthesis of multi-scalar interactions between people and their home environment. Urban Ecosyst. 15, 19–52. doi: 10.1007/s11252-011-0197-0
Cotrufo, M. F., Soong, J. L., Horton, A. J., Campbell, E. E., Haddix, M. L., Wall, D. H., et al. (2015). Formation of soil organic matter via biochemical and physical pathways of litter mass loss. Nat. Geosci. 8, 776–779. doi: 10.1038/ngeo2520
Cotrufo, M. F., Wallenstein, M. D., Boot, C. M., Denef, K., and Paul, E. (2013). The Microbial Efficiency-Matrix Stabilization (MEMS) framework integrates plant litter decomposition with soil organic matter stabilization: do labile plant inputs form stable soil organic matter? Glob. Change Biol. 19, 988–995. doi: 10.1111/gcb.12113
Craine, J. M., Morrow, C., and Fierer, N. (2007). Microbial nitrogen limitation increases decomposition. Ecology 88, 2105–2113. doi: 10.1890/06-1847.1
Crawley, M. J., Johnston, A. E., Silvertown, J., Dodd, M., Mazancourt, C. d., et al. (2005). Determinants of species richness in the Park Grass Experiment. Am. Nat. 165, 179–192. doi: 10.1086/427270
Crouch, J. A., Carter, Z., Ismaiel, A., and Roberts, J. A. (2017). The US National Mall microbiome: a census of rhizosphere bacteria inhabiting landscape turf. Crop Sci. 57(suppl. 1), S-341–S-348. doi: 10.2135/cropsci2016.10.0849
Department of Energy Environmental Protection, S.o.C. (2012). Lawn Care Pesticide Ban on Grounds of Schools and Day Care Centers. Available online at: http://www.ct.gov/deep/cwp/view.asp?A=2708&Q=468812 (accessed August 14, 2018).
Department of Environmental Conservation, N.Y.S. (2010). Pesticide Management for Schools, Day Care Centers, and Parents. Available online at: https://dec.ny.gov/chemical/41822.html (accessed August 14, 2018).
Falk, J. H. (1980). The primary productivity of lawns in a temperate environment. J. Appl. Ecol. 17, 689–695. doi: 10.2307/2402647
Fanin, N., Moorhead, D., and Bertrand, I. (2016). Eco-enzymatic stoichiometry and enzymatic vectors reveal differential C, N, P dynamics in decaying litter along a land-use gradient. Biogeochemistry 129, 1–16. doi: 10.1007/s10533-016-0217-5
Fierer, N., Bradford, M. A., and Jackson, R. B. (2007). Toward an ecological classification of soil bacteria. Ecology 88, 1354–1364. doi: 10.1890/05-1839
Fierer, N., Grandy, A. S., Six, J., and Paul, E. A. (2009a). Searching for unifying principles in soil ecology. Soil Biol. Biochem. 41, 2249–2256. doi: 10.1016/j.soilbio.2009.06.009
Fierer, N., Strickland, M. S., Liptzin, D., Bradford, M. A., and Cleveland, C. C. (2009b). Global patterns in belowground communities. Ecol. Lett. 12, 1238–1249. doi: 10.1111/j.1461-0248.2009.01360.x
Golubiewski, N. E. (2006). Urbanization increases grassland carbon pools: effects of landscaping in Colorado's Front Range. Ecol. Appl. 16, 555–571. doi: 10.1890/1051-0761(2006)016[0555:UIGCPE]2.0.CO;2
Grandy, A. S., and Neff, J. C. (2008). Molecular C dynamics downstream: the biochemical decomposition sequence and its impact on soil organic matter structure and function. Sci. Total Environ. 404, 297–307. doi: 10.1016/j.scitotenv.2007.11.013
Grandy, A. S., and Robertson, G. P. (2007). Land-use intensity effects on soil organic carbon accumulation rates and mechanisms. Ecosystems 10, 59–74. doi: 10.1007/s10021-006-9010-y
Grandy, A. S., Salam, D. S., Wickings, K., McDaniel, M. D., Culman, S. W., and Snapp, S. S. (2013). Soil respiration and litter decomposition responses to nitrogen fertilization rate in no-till corn systems. Agr. Ecosyst. Environ. 179, 35–40. doi: 10.1016/j.agee.2013.04.020
Groffman, P. M., Eagan, P., Sullivan, W., and Lemunyon, J. L. (1996). Grass species and soil type effects on microbial biomass and activity. Plant Soil 183, 61–67. doi: 10.1007/BF02185565
Groffman, P. M., Law, N. L., Belt, K. T., Band, L. E., and Fisher, G. T. (2004). Nitrogen fluxes and retention in urban watershed ecosystems. Ecosystems 7, 393–403. doi: 10.1007/s10021-003-0039-x
Groffman, P. M., Williams, C. O., Pouyat, R. V., Band, L. E., and Yesilonis, I. D. (2009). Nitrate leaching and nitrous oxide flux in urban forests and grasslands. J. Environ. Qual. 38, 1848–1860. doi: 10.2134/jeq2008.0521
Grossi, N., Fontanelli, M., Garramone, E., Peruzzi, A., Raffaelli, M., Pirchio, M., et al. (2016). Autonomous mower saves energy and improves quality of tall fescue lawn. HortTechnology 26, 825–830. doi: 10.21273/HORTTECH03483-16
Guertal, E. (2012). “Carbon sequestration in turfed landscapes: a review,” in Carbon Sequestration in Urban Ecosystems, eds Rattan, L. (Dordrecht: Springer), 197–213. doi: 10.1007/978-94-007-2366-5_10
Hamilton, E. W., and Frank, D. A. (2001). Can plants stimulate soil microbes and their own nutrient supply? Evidence from a grazing tolerant grass. Ecology 82, 2397–2402. doi: 10.1890/0012-9658(2001)082[2397:CPSSMA]2.0.CO;2
Hedblom, M., Lindberg, F., Vogel, E., Wissman, J., and Ahrné, K. (2017). Estimating urban lawn cover in space and time: case studies in three Swedish cities. Urban Ecosyst. 20, 1109–1119. doi: 10.1007/s11252-017-0658-1
Ignatieva, M., and Hedblom, M. (2018). An alternative urban green carpet. Science 362, 148–149. doi: 10.1126/science.aau6974
Isobe, K., and Ohte, N. (2014). Ecological perspectives on microbes involved in N-cycling. Microb. Environ. 29, 4–16. doi: 10.1264/jsme2.ME13159
Janssen, P. H. (2006). Identifying the dominant soil bacterial taxa in libraries of 16S rRNA and 16S rRNA genes. Appl. Environ. Microbiol. 72, 1719–1728. doi: 10.1128/AEM.72.3.1719-1728.2006
Kaye, J. P., Groffman, P. M., Grimm, N. B., Baker, L. A., and Pouyat, R. V. (2006). A distinct urban biogeochemistry? Trends Ecol. Evol. 21, 192–199. doi: 10.1016/j.tree.2005.12.006
Kaye, J. P., McCulley, R. L., and Burke, I. C. (2005). Carbon fluxes, nitrogen cycling, and soil microbial communities in adjacent urban, native and agricultural ecosystems. Glob. Change Biol. 11, 575–587. doi: 10.1111/j.1365-2486.2005.00921.x
Konstantinidis, K. T., and Tiedje, J. M. (2004). Trends between gene content and genome size in prokaryotic species with larger genomes. Proc. Natl. Acad. Sci. U.S.A. 101, 3160–3165. doi: 10.1073/pnas.0308653100
Kopp, K. L., and Guillard, K. (2002). Clipping management and nitrogen fertilization of turfgrass. Crop Sci. 42, 1225–1231. doi: 10.2135/cropsci2002.1225
Kuzyakov, Y., Biryukova, O., Kuznetzova, T., Mölter, K., Kandeler, E., and Stahr, K. (2002). Carbon partitioning in plant and soil, carbon dioxide fluxes and enzyme activities as affected by cutting ryegrass. Biol. Fert. Soils 35, 348–358. doi: 10.1007/s00374-002-0480-6
Law, Q. D., Trappe, J. M., Jiang, Y., Turco, R. F., and Patton, A. J. (2017). Turfgrass selection and grass clippings management influence soil carbon and nitrogen dynamics. Agron. J. 109, 1719–1725. doi: 10.2134/agronj2016.05.0307
Leff, J. W., Jones, S. E., Prober, S. M., Barberán, A., Borer, E. T., Firn, J. L., et al. (2015). Consistent responses of soil microbial communities to elevated nutrient inputs in grasslands across the globe. Proc. Natl. Acad. Sci. U.S.A. 112, 10967–10972. doi: 10.1073/pnas.1508382112
Liang, C., Schimel, J. P., and Jastrow, J. D. (2017). The importance of anabolism in microbial control over soil carbon storage. Nat. Microbiol. 2:17105. doi: 10.1038/nmicrobiol.2017.105
Mambelli, S., Bird, J. A., Gleixner, G., Dawson, T. E., and Torn, M. S. (2011). Relative contribution of foliar and fine root pine litter to the molecular composition of soil organic matter after in situ degradation. Organ. Geochem. 42, 1099–1108. doi: 10.1016/j.orggeochem.2011.06.008
Marschner, B., Brodowski, S., Dreves, A., Gleixner, G., Gude, A., Grootes, P. M., et al. (2008). How relevant is recalcitrance for the stabilization of organic matter in soils? J. Plant Nutr. Soil Sci. 171, 91–110. doi: 10.1002/jpln.200700049
Maryland, S. O. (2018). MD's Lawn Fertilizer Law. Available online at: http://mda.maryland.gov/Pages/fertilizer.aspx (accessed April 29, 2018).
Milesi, C., Running, S. W., Elvidge, C. D., Dietz, J. B., Tuttle, B. T., and Nemani, R. R. (2005). Mapping and modeling the biogeochemical cycling of turf grasses in the United States. Environ. Manag. 36, 426–438. doi: 10.1007/s00267-004-0316-2
Monteiro, J. A. (2017). Ecosystem services from turfgrass landscapes. Urban For. Urban Gree. 26, 151–157. doi: 10.1016/j.ufug.2017.04.001
Morrison, E. W., Pringle, A., van Diepen, L. T. A., Grandy, A. S., Melillo, J. M., and Frey, S. D. (2019). Warming alters fungal communities and litter chemistry with implications for soil carbon stocks. Soil Biol. Biochem. 132, 120–130. doi: 10.1016/j.soilbio.2019.02.005
Murphy, J. A., and Ebdon, J. S. (2013). “Study and management of turfgrass traffic stress,” in Turfgrass: Biology, Use, and Management, eds. Stier, J. C., Horgan, B. P., and Bonos, S. A. (Madison, WI: American Society of Agronomy; Crop Science Society of America; Soil Science Society of America), 1029–1074. doi: 10.2134/agronmonogr56.c27
Pasari, J. R., Levi, T., Zavaleta, E. S., and Tilman, D. (2013). Several scales of biodiversity affect ecosystem multifunctionality. Proc. Natl. Acad. Sci. U.S.A. 110, 10219–10222. doi: 10.1073/pnas.1220333110
Pataki, D. E., Carreiro, M. M., Cherrier, J., Grulke, N. E., Jennings, V., Pincetl, S., et al. (2011). Coupling biogeochemical cycles in urban environments: ecosystem services, green solutions, and misconceptions. Front. Ecol. Environ. 9:90220. doi: 10.1890/090220
Petrovic, A. M. (1990). The fate of nitrogenous fertilizers applied to turfgrass. J. Environ. Qual. 19, 1–14. doi: 10.2134/jeq1990.00472425001900010001x
Pickett, S. T. A., Cadenasso, M. L., Grove, J. M., Groffman, P. M., Band, L. E., Boone, C. G., et al. (2008). Beyond urban legends: an emerging framework of urban ecology, as illustrated by the Baltimore Ecosystem Study. BioScience 58, 139–150. doi: 10.1641/B580208
Porter, K., Bouldin, D., Pacenka, S., Kossack, R., and Shoemaker, C. (1980). Studies to Assess the Fate of Nitrogen Applied to Turf: Part I. Ithaca, NY: Cornell University.
Pouyat, R., Groffman, P., Yesilonis, I., and Hernandez, L. (2002). Soil carbon pools and fluxes in urban ecosystems. Environ. Pollut. 116, S107–S118. doi: 10.1016/S0269-7491(01)00263-9
Pouyat, R. V., Yesilonis, I. D., and Golubiewski, N. E. (2009). A comparison of soil organic carbon stocks between residential turf grass and native soil. Urban Ecosyst. 12, 45–62. doi: 10.1007/s11252-008-0059-6
Pouyat, R. V., Yesilonis, I. D., and Nowak, D. J. (2006). Carbon storage by urban soils in the United States. J. Environ. Qual. 35, 1566–1575. doi: 10.2134/jeq2005.0215
Puhalla, J., Krans, J. V., and Michael Goatley, J. (2010). Sports Fields: Design, Construction, and Maintenance. Hoboken, NJ: Wiley.
Qian, Y., and Follett, R. (2012). “Carbon dynamics and sequestration in urban turfgrass ecosystems,” in Carbon Sequestration in Urban Ecosystems, eds Rattan, L., and Bruce, A. (Dordrecht: Springer), 161–172. doi: 10.1007/978-94-007-2366-5_8
Qian, Y., and Follett, R. F. (2002). Assessing soil carbon sequestration in turfgrass systems using long-term soil testing data. Agron. J. 94, 930–935. doi: 10.2134/agronj2002.9300
Qian, Y., Follett, R. F., and Kimble, J. M. (2010). Soil organic carbon input from urban turfgrasses. Soil Sci. Soc. Am. J. 74, 366–371. doi: 10.2136/sssaj2009.0075
Qian, Y. L., Bandaranayake, W., Parton, W. J., Mecham, B., Harivandi, M. A., and Mosier, A. R. (2003). Long-term effects of clipping and nitrogen management in turfgrass on soil organic carbon and nitrogen dynamics. J. Environ. Qual. 32, 1694–1700. doi: 10.2134/jeq2003.1694
Raciti, S. M., Groffman, P., and Fahey, T. (2008). Nitrogen retention in urban lawns and forests. Ecol. Appl. 18, 1615–1626. doi: 10.1890/07-1062.1
Raciti, S. M., Groffman, P. M., Jenkins, J. C., Pouyat, R. V., Fahey, T. J., Pickett, S. T., et al. (2011). Accumulation of carbon and nitrogen in residential soils with different land-use histories. Ecosystems 14, 287–297. doi: 10.1007/s10021-010-9409-3
Reisinger, A. J., Groffman, P. M., and Rosi-Marshall, E. J. (2016). Nitrogen-cycling process rates across urban ecosystems. FEMS Microbiol. Ecol. 92, fiw198. doi: 10.1093/femsec/fiw198
Robbins, P., and Birkenholtz, T. (2003). Turfgrass revolution: measuring the expansion of the American lawn. Land Use Policy 20, 181–194. doi: 10.1016/S0264-8377(03)00006-1
Robertson, A. D., Paustian, K., Ogle, S., Wallenstein, M. D., Lugato, E., and Cotrufo, M. F. (2019). Unifying soil organic matter formation and persistence frameworks: the MEMS model. Biogeosciences 16, 1225–1248. doi: 10.5194/bg-16-1225-2019
Rubino, M., Dungait, J. A. J., Evershed, R. P., Bertolini, T., De Angelis, P., D'Onofrio, A., et al. (2010). Carbon input belowground is the major C flux contributing to leaf litter mass loss: Evidences from a 13C labelled-leaf litter experiment. Soil Biol. Biochem. 42, 1009–1016. doi: 10.1016/j.soilbio.2010.02.018
Schimel, J., Bennett, J., Fierer, N., Bardgett, R., Usher, M., and Hopkins, D. (2005). Microbial Community Composition and Soil Nitrogen Cycling: Is There Really a Connection? New York, NY: Cambridge University Press.
Secretariat of the Convention on Biological Diversity (2012). Cities and Biodiversity Outlook. Montreal, QC: Secretariat of the Convention on Biological Diversity.
Shi, W., Bowman, D., and Rufty, T. (2007). Soil microbial community composition and function in turfgrass ecosystems. Bioremed. Biodivers. Bioavailab. 1, 72–77.
Shi, W., Bowman, D., and Rufty, T. (2012). “Microbial control of soil carbon accumulation in turfgrass systems,” in Carbon Sequestration in Urban Ecosystems. (Dordrecht: Springer), 215–231. doi: 10.1007/978-94-007-2366-5_11
Shi, W., Muruganandam, S., and Bowman, D. (2006). Soil microbial biomass and nitrogen dynamics in a turfgrass chronosequence: a short-term response to turfgrass clipping addition. Soil Biol. Biochem. 38, 2032–2042. doi: 10.1016/j.soilbio.2006.01.005
Sinsabaugh, R. L. (2010). Phenol oxidase, peroxidase and organic matter dynamics of soil. Soil Biol. Biochem. 42, 391–404. doi: 10.1016/j.soilbio.2009.10.014
Sinsabaugh, R. L., Lauber, C. L., Weintraub, M. N., Ahmed, B., Allison, S. D., Crenshaw, C., et al. (2008). Stoichiometry of soil enzyme activity at global scale. Ecol. Lett. 11, 1252–1264. doi: 10.1111/j.1461-0248.2008.01245.x
Six, J., Conant, R., Paul, E., and Paustian, K. (2002). Stabilization mechanisms of soil organic matter: implications for C-saturation of soils. Plant Soil 241, 155–176. doi: 10.1023/A:1016125726789
Smith, R. M., Williamson, J. C., Pataki, D. E., Ehleringer, J., and Dennison, P. (2018). Soil carbon and nitrogen accumulation in residential lawns of the Salt Lake Valley, Utah. Oecologia 187, 1107–1118. doi: 10.1007/s00442-018-4194-3
Stier, J. C., Horgan, B. P., and Bonos, S. A. (eds.). (2013). Turfgrass: Biology, Use, and Management. Madison, WI: American Society of Agronomy; Crop Science Society of America; Soil Science Society of America.
Szlavecz, K., Terzis, A., Ozer, S., Musaloiu-E, R., Cogan, J., et al. (2007). Life under your feet: an end-to-end soil ecology sensor network, database, web server, and analysis service. arXiv preprint cs/0701170.
Terzis, A., Musaloiu, -E R, Cogan, J., Szlavecz, K., Szalay, A., et al. (2010). Wireless sensor networks for soil science. Int. J. Sens. Netw. 7, 53–70. doi: 10.1504/IJSNET.2010.031850
Thompson, G. L., and Kao-Kniffin, J. (2016). Diversity enhances NPP, N retention, and soil microbial diversity in experimental urban grassland assemblages. PLoS ONE 11:e0155986. doi: 10.1371/journal.pone.0155986
Thompson, G. L., and Kao-Kniffin, J. (2017). Applying biodiversity and ecosystem function theory to turfgrass management. Crop Sci. 57(suppl. 1), S-238–S-248. doi: 10.2135/cropsci2016.05.0433
Townsend-Small, A., and Czimczik, C. I. (2010). “Correction to Carbon sequestration and greenhouse gas emissions in urban turf,” Geophys. Res. Lett. 37, L06707. doi: 10.1029/2010GL042735
Trammell, T. L. E., Pataki, D. E., Still, C. J., Ehleringer, J. R., Avolio, M. L., Bettez, N., et al. (2019). Climate and lawn management interact to control C4 plant distribution in residential lawns across seven U.S. cities. Ecol. Appl. 29:e01884. doi: 10.1002/eap.1884
US Census Bureau (2011). “Population Distribution and Change: 2000 to 2010,” in 2010 Census Briefs, U.S. Department of Commerce (Washington, DC: US Census Bureau), 1–12.
Wang, H., Marshall, C. W., Cheng, M., Xu, H., Li, H., Yang, X., et al. (2017). Changes in land use driven by urbanization impact nitrogen cycling and the microbial community composition in soils. Sci. Rep. 7:44049. doi: 10.1038/srep44049
Wang, W. W., Haver, D., and Pataki, D. E. (2014). Nitrogen budgets of urban lawns under three different management regimes in southern California. Biogeochemistry 121, 127–148. doi: 10.1007/s10533-013-9942-1
WHO (2010). Hidden Cities: Unmasking and Overcoming Health Inequities in Urban Settings. Kobe: World Health Organization.
Wieder, W., Grandy, A., Kallenbach, C., and Bonan, G. (2014). Integrating microbial physiology and physio-chemical principles in soils with the MIcrobial-MIneral Carbon Stabilization (MIMICS) model. Biogeosciences 11, 3899–3917. doi: 10.5194/bg-11-3899-2014
Wieder, W. R., Bonan, G. B., and Allison, S. D. (2013). Global soil carbon projections are improved by modelling microbial processes. Nat. Clim. Change 3, 909–912. doi: 10.1038/nclimate1951
Yao, H., Bowman, D., Rufty, T., and Shi, W. (2009). Interactions between N fertilization, grass clipping addition and pH in turf ecosystems: implications for soil enzyme activities and organic matter decomposition. Soil Biol. Biochem. 41, 1425–1432. doi: 10.1016/j.soilbio.2009.03.020
Yao, H., Bowman, D., and Shi, W. (2006). Soil microbial community structure and diversity in a turfgrass chronosequence: land-use change versus turfgrass management. Appl. Soil Ecol. 34, 209–218. doi: 10.1016/j.apsoil.2006.01.009
Yao, H., and Shi, W. (2010). Soil organic matter stabilization in turfgrass ecosystems: importance of microbial processing. Soil Biol. Biochem. 42, 642–648. doi: 10.1016/j.soilbio.2010.01.003
Zabinski, C. A., and Gannon, J. E. (1997). Effects of recreational impacts on soil microbial communities. Environ. Manag. 21, 233–238. doi: 10.1007/s002679900022
Keywords: urban grasslands, soil microbiome, soil C and N, biogeochemistry, ecosystem services, turfgrass
Citation: Thompson GL and Kao-Kniffin J (2019) Urban Grassland Management Implications for Soil C and N Dynamics: A Microbial Perspective. Front. Ecol. Evol. 7:315. doi: 10.3389/fevo.2019.00315
Received: 31 January 2019; Accepted: 06 August 2019;
Published: 27 August 2019.
Edited by:
Galina Churkina, Yale University, United StatesReviewed by:
Shan Yin, Shanghai Jiao Tong University, ChinaIngo Schöning, Max-Planck-Institut für Biogeochemie, Germany
Copyright © 2019 Thompson and Kao-Kniffin. This is an open-access article distributed under the terms of the Creative Commons Attribution License (CC BY). The use, distribution or reproduction in other forums is permitted, provided the original author(s) and the copyright owner(s) are credited and that the original publication in this journal is cited, in accordance with accepted academic practice. No use, distribution or reproduction is permitted which does not comply with these terms.
*Correspondence: Jenny Kao-Kniffin, jtk57@cornell.edu
†Present Address: Grant L. Thompson, Department of Horticulture, Iowa State University, Ames, IA, United States