Molecular Characterization and Immuno-Reactivity Patterns of a Novel Plasmodium falciparum Armadillo-Type Repeat Protein, PfATRP
- 1West African Center for Cell Biology of Infectious Pathogens, University of Ghana, Accra, Ghana
- 2Department of Biochemistry, Kogi State University, Anyigba, Nigeria
- 3Department of Biomedical Sciences, SBBS, University of Health and Allied Sciences, Ho, Ghana
- 4Cambridge Institute for Medical Research, University of Cambridge, Cambridge, United Kingdom
- 5Department of Biochemistry, Cell and Molecular Biology, College of Basic and Applied Sciences, University of Ghana, Accra, Ghana
Nearly half of the genes in the Plasmodium falciparum genome have not yet been functionally investigated. We used homology-based structural modeling to identify multiple copies of Armadillo repeats within one uncharacterized gene expressed during the intraerythrocytic stages, PF3D7_0410600, subsequently referred to as P. falciparum Armadillo-Type Repeat Protein (PfATRP). Soluble recombinant PfATRP was expressed in a bacterial expression system, purified to apparent homogeneity and the identity of the recombinant PfATRP was confirmed by mass spectrometry. Affinity-purified α-PfATRP rabbit antibodies specifically recognized the recombinant protein. Immunofluorescence assays revealed that α-PfATRP rabbit antibodies reacted with P. falciparum schizonts. Anti-PfATRP antibody exhibited peripheral staining patterns around the merozoites. Given the localization of PfATRP in merozoites, we tested for an egress phenotype during schizont arrest assays and demonstrated that native PfATRP is inaccessible on the surface of merozoites in intact schizonts. Dual immunofluorescence assays with markers for the inner membrane complex (IMC) and microtubules suggest partial colocalization in both asexual and sexual stage parasites. Using the soluble recombinant PfATRP in a screen of plasma samples revealed that malaria-infected children have naturally acquired PfATRP-specific antibodies.
Introduction
Malaria is a major global health problem that poses a threat to half of the world's population. Globally, 272 000 malaria deaths were estimated to be in children aged under 5 years (WHO, 2019). The intra-erythrocytic stage of the parasite life cycle is a key target for vaccine and drug development, since all clinical symptoms of malaria occur during this stage. Erythrocyte invasion by the malaria parasite is a complicated and highly coordinated process that involves attachment and penetration mediated by a sophisticated network of parasite proteins discharged from two apical secretory organelles, the rhoptries and micronemes (Cowman et al., 2017). Another key invasion-associated organelle is the inner membrane complex (IMC), which contributes to the maintenance of cell morphology and rigidity (Aikawa et al., 1981; Meszoely et al., 1987; Kono et al., 2012), but also plays a role in motility and invasion, by acting as an anchor for the actin-myosin motor that provides the pre-requisite force necessary for invasion processes (Soldati et al., 2004; Baum et al., 2006, 2008; Jones et al., 2006; Yeoman et al., 2011).
The Armadillo Repeat Motif (ARM) is present in proteins across the eukaryotic lineage and has been associated with protein-protein interactions such as bridging the cytoplasmic domains of cadherins to α-catenin and the actin cytoskeleton (McCrea et al., 1991; Hülsken et al., 1994). In Plasmodium and other related parasites, ARM-containing proteins have been shown to have multiple functions including DNA-binding (Mitra et al., 2016), apical positioning of the rhoptry organelle, a pre-requisite for host cell invasion (Mueller et al., 2013), clustering of rhoptry organelles (Mueller et al., 2016), cell signaling, cytoskeletal organization, gene regulation (Coates, 2003; Tewari et al., 2010) and recently, IMC formation (Absalon et al., 2016). PF3D7_0410600-interacting partner proteins have been discovered using a proteome-wide yeast-2-hybrid screen approach (LaCount et al., 2005). Interestingly, one of the PF3D7_0410600 binding partners; 14-3-3 is a hub protein that plays important roles in many regulatory processes including mitogenic signal transduction, apoptotic cell death, cell cycle control, and protein localization (Fu et al., 2000; Schechtman et al., 2001; Assossou et al., 2003). In T. gondii, 14-3-3 protein has shown potential as a vaccine candidate against toxoplasmosis (Meng et al., 2012) and the protein has been implicated in the mechanism developed by parasites to stimulate host immune responses. Considering the future prospects in taking advantage of protein-protein interactions for the development of better diagnostic tools for malaria infection, we sought to functionally characterize PF3D7_0410600 protein especially that it may play an important role in parasite-specific processes or could be a potential biomarker.
In this report, we identified a novel P. falciparum, Armadillo-Type Repeat Protein (PfATRP: PF3D7_0410600/PFD0525w) based on its gene expression profile and potential function in protein-protein interactions. We hypothesized that PfATRP may be involved in merozoite egress or invasion and tested for an egress phenotype during schizont arrest assays and demonstrated that native PfATRP is inaccessible on the surface of merozoites in intact schizonts. We also determined the subcellular localization of the protein and measured its antibody levels in children exposed to varying intensities of P. falciparum infection in Ghana.
Results
PfATRP Structural Characteristics and Time-Resolved Expression Analysis
The PfATRP gene (PF3D7_0410600) is a 3-exon gene located on chromosome 4 and encodes a 326-amino acid protein with a predicted molecular weight of 32 kDa. Plasmodium database (PlasmoDB) shows that the protein lacks a recognizable signal peptide and transmembrane domain (Figure 1A). Also, expert protein analysis system (ExPASy), (Gasteiger et al., 2005) indicates that the protein does not possess any myristoylation or acetylation signal (Figure 1A). PfATRP is evolutionarily conserved across rodent and primate Plasmodium species (Figure 1B) and all orthologs have a positionally-conserved cysteine residue at the C-terminal end of the protein. PfATRP structure was predicted by homology modeling using both Phyre 2 and I-TASSER. Homology modeling by Phyre 2 predicts that PfATRP exhibits structural features of the β-catenin family that harbor armadillo repeats (Figure S1A). Similar modeling using I-TASSER, revealed that PfATRP shares structural similarities with importin-β (Figure S1B). These results from the two protein prediction servers were consistent, since armadillo repeats are known to adopt similar structural conformation in β-catenin and importins (Lee et al., 2000; Koike et al., 2004). Overall, PfATRP seems to be globular in nature with a very short disordered region at the C-terminus. Also, PfATRP possesses an Armadillo Repeat Motif (ARM), a characteristic feature of the β-Catenin family of proteins. These motifs are also potential docking sites for protein-protein interactions (Coates, 2003; Tewari et al., 2010), and ARM containing proteins have been shown to be associated with invasion-related organelles in P. falciparum merozoites.
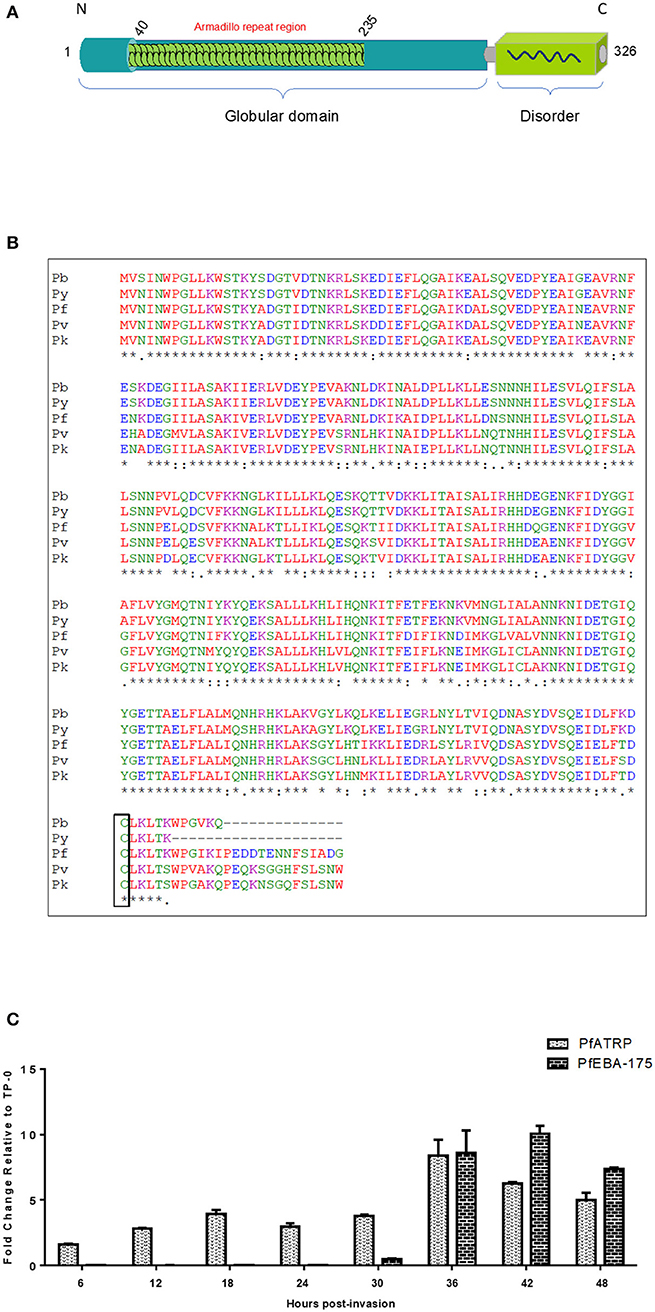
Figure 1. PfATRP structural characteristics and time-resolved expression analysis. (A) Domain architecture of PfATRP showing the features of the full-length protein. (B) Clustal 0 (1.2.4) multiple sequence alignment (72% similarity) shows that PfATRP is conserved across different Plasmodium species orthologs. (C) Time-resolved qRT-PCR expression profile of PfATRP asexual parasite stages. The gene transcript levels for PfATRP was analyzed with PfEBA-175 as control at eight (8) different time-points (6 h interval).
To confirm when PfATRP was expressed, we extracted RNA from multiple time points across the P. falciparum spaced six (6) h apart. Time-resolved qRT-PCR revealed that PfATRP is expressed through all the time-points during the asexual stage. However, PfATRP and PfEBA-175 (Bozdech et al., 2003) peaks maximally at between 36–42 h post-invasion, respectively (Figure 1C), suggesting that PfATRP may also play a role in egress or invasion. These data are in keeping with previously published RNAseq data (Otto et al., 2010), which also confirm a late-stage expression profile for PfATRP, and suggests a role in late-stage development, such as merozoite generation, egress, or erythrocyte invasion.
Production of Recombinant PfATRP and Antibody Generation
Recombinant PfATRP expressed in bacterial system produced a dominant 35 kDa protein species consistent with its theoretical molecular weight determined based on its codon-optimized sequence (Figures S2, S3A) Recombinant PfATRP was purified under non-denaturing conditions using immobilized metal affinity column that resulted in the enrichment of both 35 kDa and a second, much fainter, ~80 kDa recombinant PfATRP species (Figure S3B). Anti-6xHistidine tag mouse monoclonal antibody detected the 35 kDa and ~80 kDa species of PfATRP during immunoblotting of the nickel-nitrilotriacetic acid (Ni-NTA) purified recombinant protein (Figures 2i,ii). The Ni-NTA eluates were pooled, concentrated using 10 kDa cutoff centricons and further purified on size exclusion chromatography (SEC) column (Figure S3B). Using the codon optimized sequences (Figures S2i-iv), the control antigens, PF3D7_1404900 (30 kDa), PfMSP7 (50 kDa), and PF3D7_0308300 (40 kDa) were expressed and purified to apparent homogeneity (Figure S4). The identities of the control recombinant proteins were confirmed by immunoblotting using α-6xHistidine mouse monoclonal antibody (Figure S4).
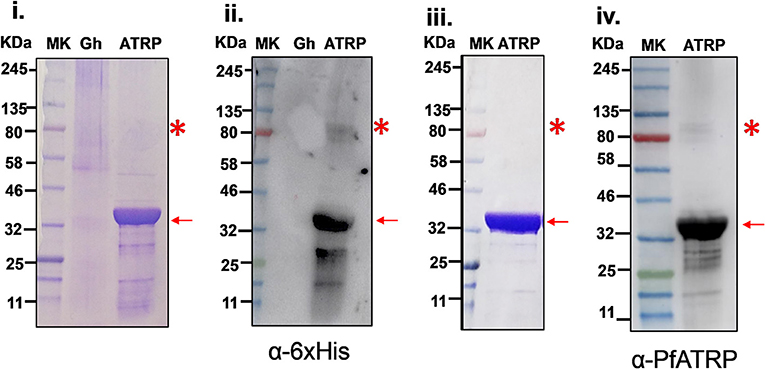
Figure 2. Production of recombinant PfATRP and immunoblotting. (i) Coomassie-stained gel shows the recombinant PfATRP (35kDa) that was purified on Ni-NTA column. (ii) The recombinant protein was detected by immunoblotting using α-Histidine mouse monoclonal antibody. (iii-iv) Coomassie-stained gel shows the recombinant PfATRP (35 kDa) which was also detected by immunoblotting using α-PfATRP rabbit antibody. Gh: solubilized erythrocyte ghost membrane proteins. Protein samples were ran along side with color, pre-stained protein standard (P7712S) broad range (11–245 kDa).
The identity of the recombinant PfATRP was further confirmed by Liquid chromatography-Mass Spectrometry (LC-MS), (Table S1) and recombinant PfATRP was immunized in rabbits following a prime-double booster immunization regimen and antibodies were generated by Biobasic, Canada. To explore the identity of the two bands detected by α-6xHistidine mouse monoclonal antibody (Figures 2i,ii), immunoblotting was performed for the SEC-purified PfATRP. PfATRP was specifically detected during immunoblotting using α-PfATRP rabbit antibody (Figures 2iii,iv) which indicated that SEC removed most of the ~80 kDa recombinant PfATRP species.
Anti-PfATRP Rabbit Antibodies Reacts With Plasmodium falciparum Mature Schizonts
To determine if α-PfATRP rabbit antibody specifically reacts with native PfATRP in schizont lysates, we performed immunoblotting using 3D7 detergent-treated schizont lysates. Coomassie stained gel shows the profile of proteins in the parasite lysates which was probed with α-PfATRP rabbit antibody that specifically recognized the 35 kDa protein band corresponding to the expected molecular weight for native PfATRP (Figures 3Ai,ii). The detection of a prominent signal during immunoblotting for native PfATRP excludes the possibility of any off-target reactivity of α-PfATRP rabbit antibody. Next, we performed permeabilized dual immunofluorescence assays (IFAs) during which we tested whether α-PfAMA1 (micronemal marker) or α-PfMSP 1 mouse and α-PfATRP rabbit antibodies reacted with segmenting schizonts. These showed that α-PfAMA1 mouse and α-PfATRP rabbit antibodies labeled the periphery of schizonts with dotty staining patterns suggesting an overlap of PfATRP with PfAMA1 (Figure 3B). We performed co-staining of α-PfATRP rabbit and α-PfMSP1 mouse antibodies in asexual stage parasites and this indicated that PfATRP is not likely localized on the merozoite surface (Figure 3C and Figure S4). Similarly, dual IFAs were performed for gametocytes using α-PfATRP rabbit antibody and α-Pfs48/45 mouse antibody) which showed that PfATRP is not localized on the surface of gametocytes (Figure S5).
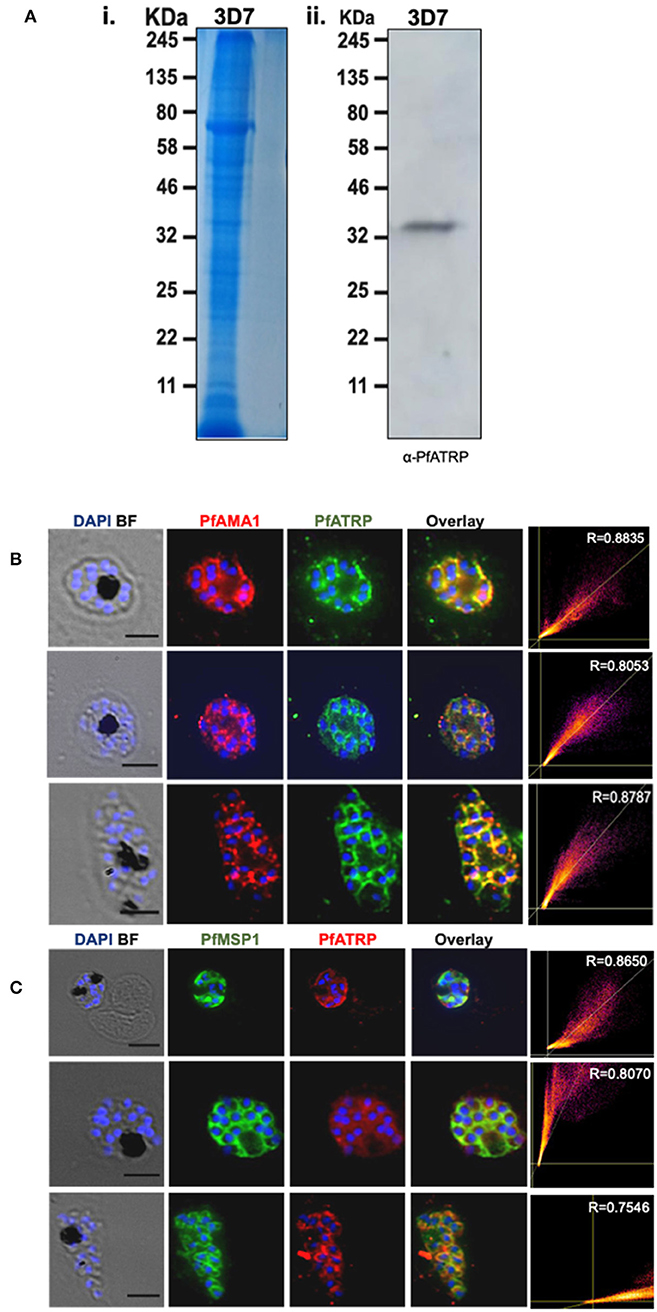
Figure 3. Anti-PfATRP rabbit antibody reacts with P. falciparum matured schizonts. (A) Commassie stained gel showing the profile of proteins in 3D7 detergent-treated schizont lysates (i). Anti-PfATRP rabbit antibody detects native PfATRP in 3D7 detergent-treated schizonts lysates by immunoblotting (ii). (B) Dual IFA staining performed under permeabilized conditions using segmenting schizonts showed that α-PfAMA1 mouse antibody (red); (1:100) and α-PfATRP rabbit antibody (green); 1:100 labeled schizonts. (C) α-PfATRP rabbit antibody (red); (1:100) and α-PfMSP1 mouse antibody (green); (1:100) labeled schizonts. The nuclei were stained with DAPI. Black lines on IFA images represent scale bar. R signifies the colocalization coefficient.
PfATRP Is Not Localized on the Parasite Surface
Given ATRP location in developing merozoites, one possibility is that it may be involved in merozoite egress from infected erythrocytes. We tested for an egress phenotype using the schizont arrest assay that is based on the permeability of parasitized erythrocytes to macromolecules, including antibodies, at the later stages of schizogony (Ahlborg et al., 1996; Goodyer et al., 1997; Bergmann-Leitner et al., 2009; Raj et al., 2014). We showed only ~15% egress inhibition by 10 μM E64, a cysteine protease that is known to arrest the egress of merozoites and subsequently resulted in low parasitemia upon reinvasion of erythrocytes. This was because we used segmented schizonts and this was to ensure antibodies got access to the parasites within the erythrocytes. Besides, it was reported previously (Hill et al., 2012) that segmented schizonts are less affected by E64 treatment. Anti-PfATRP rabbit antibody at 100 μg/mL did not block merozoite egress or subsequent reinvasion of new erythrocytes (Figure 4A). This suggests that PfATRP may not be accessible on the parasite surface.
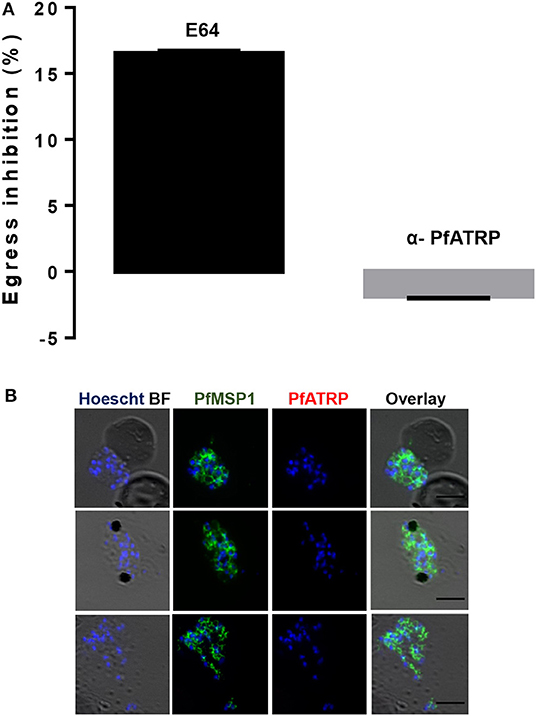
Figure 4. PfATRP is not localized on the parasite surface. (A) Egress assays were performed with 3D7 parasites using α-PfATRP rabbit antibody and E64 was used as control, showing that α-PfATRP rabbit antibody does not inhibit schizont egress or reinvasion of erythrocytes by merozoites. (B) α-PfMSP1 mouse antibody stained the surface of merozoites in non-permeabilized schizonts during in-solution IFA, but no staining was observed for α-PfATRP rabbit antibody. The nuclei were stained with Hoescht. Black lines on IFA images represent scale bar.
The IMC is the only known organelle consistent with a peripheral staining pattern around the merozoite that is not surface accessible. Proteins localizing to the IMC, a cytoskeleton compartment that sits immediately under the merozoite surface, can appear largely peripheral in IFAs. To distinguish between surface and IMC staining, we performed non-permeabilized in-solution IFA's during which we tested whether both α-PfATRP rabbit and α-PfMSP1 mouse antibodies reacted with the merozoite surface. Non-permeablized in-solution IFA's showed that α-PfMSP1 mouse antibody labeled the surfaces of merozoites in intact segmented schizonts (Figure 4B), but α-PfATRP rabbit antibodies did not label the merozoite surfaces (Figure 4B). Altogether, this provides two lines of evidence that PfATRP is not accessible on merozoite surface unlike PfMSP1 (Das et al., 2015) and PfAMA1 (Douglas et al., 2013) that are surface accessible as reported previously. PfATRP is suggested by the PlasmoGEM and piggyBac studies to be essential for blood stage growth and it is most highly expressed in schizonts. Therefore, it is likely that PfATRP has an essential function in schizonts that could be through a non-surface exposed mechanism required for merozoite development, egress or invasion.
PfATRP Probably Exhibits IMC and Microtubular Localization Patterns
To determine whether PfATRP is localized to the IMC in asexual stage parasites, we performed dual immunofluorescence staining of early-, late-, rupturing schizonts and free merozoites using α-PfATRP rabbit antibody with the IMC marker, α-Myosin A-tail Interacting Protein (PfMTIP) rat antibody (Dearnley et al., 2012). We observed that α-PfATRP rabbit antibody partly colocalizes with α-PfMTIP rat antibody (Figure 5A) indicating that a sub-population of PfATRP is localized to the IMC. In early-, late-, rupturing-schizonts and free merozoites, there was no overlap of α-PfATRP rabbit and α-Tubulin Acetyltransferase 1 (TAT1) mouse antibody. This suggests that PfATRP might not be associated with microtubules during asexual stages (Figure 5B).
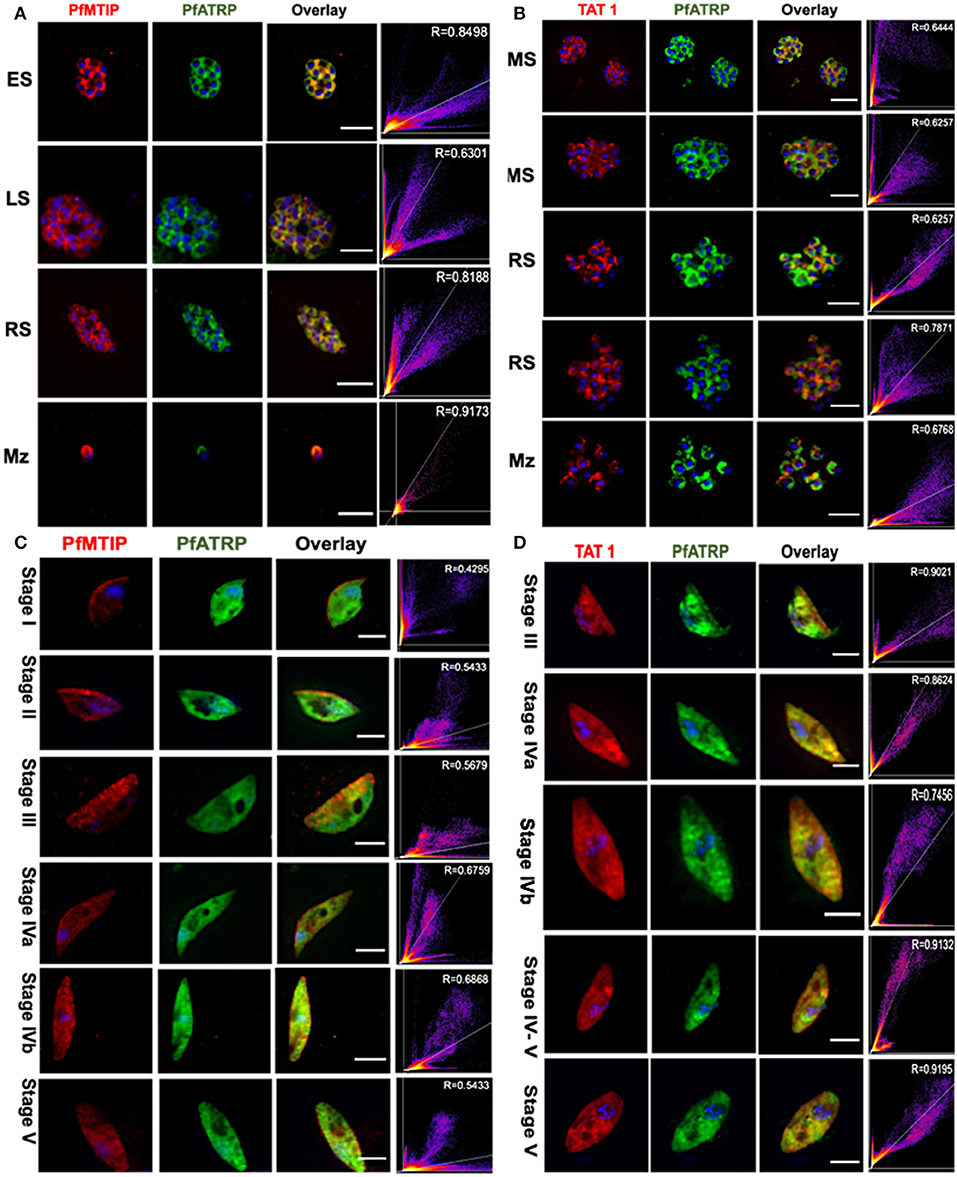
Figure 5. PfATRP probably exhibits IMC and microtubular localization pattern. Dual IFAs were performed for both asexual and sexual parasite stages using markers for different subcellular organelles. (A) Slides were prepared for asexual stage parasites (early schizonts (ES); late schizonts (LS); rupturing schizonts (RS) and free merozoites (Mz), and probed with α-PfATRP rabbit antibody (green); (1:100) and α-PfMTIP rat antibody (1:100); (red) which showed that PfATRP partly colocalized with PfMTIP in the inner membrane complex. (B) Similarly, dual IFAs were performed for matured schizonts (MS), rupturing schizonts (RS) and free merozoites (Mz). α-TAT1 mouse antibody (red); (1:10), the marker for microtubules and α-PfATRP rabbit antibody (green) (1:100) did not colocalize. (C) Gametocytes (stages I-V) were co-stained with α-PfMTIP rat antibody (red), (1:100) and α-PfATRP rabbit antibody (green), (1:100); and a restricted colocalization pattern was observed. (D) Co-staining of gametocytes with α-TAT-1 mouse antibody (red), (1:10) and α-PfATRP rabbit antibody (green); (1:100) showed colocalization which was largely cytoplasmic. White lines on IFA images represent scale bar. R signifies the colocalization coefficient.
PfATRP expression has also been reported in gametocytes (Tao et al., 2014) and based on annotated gene ontology component and predicted gene ontology function, the protein has been linked with microtubule motor activity which is associated with the dynein complex. In gametocytes, α-PfATRP rabbit antibody labeled gametocytes and a restricted colocalization pattern with α-PfMTIP rat antibody was observed (Figure 5C). Contrary to the observation in asexual stage parasites, α-PfATRP rabbit antibodies colocalizes with TAT1 (McRobert et al., 2008) that recognizes all tubulin isoforms suggesting an association with the microtubular network in gametocytes (Figure 5D). This is consistent with the crucial role of another ARM protein (PF16) which is expressed in male gamete flagellum, where it maintains the correct microtubule structure in the central apparatus of the axoneme (Straschil et al., 2010). Therefore, it is conceivable that the observed disparity in PfATRP subcellular localization during the asexual and sexual parasite stages could be developmentally regulated.
PfATRP Is Recognized by Antibodies From Individuals Naturally Exposed to P. falciparum Malaria
Malaria transmission intensity has been measured by the entomological inoculation rates and was highest in Kintampo (>250 infective bites/person per year), followed by Navrongo (<250 infective bites/person per year), and lowest in Accra (<50 infective bites/person per year)(Klinkenberg et al., 2008; Kasasa et al., 2013). The Samples used in this study were collected during the rainy seasons at the respective study sites between September 2011 and September 2013. The immunoreactivity of PfATRP in comparison with three other parasite antigens (PF3D7_1404900, PfMSP7 and PF3D7_0308300) were evaluated using plasma samples from malaria-infected children resident in three endemic areas within Ghana with varying transmission intensities (Klinkenberg et al., 2008; Kasasa et al., 2013).
PF3D7_1404900 (PF14_0046) is a novel protein that was localized at the parasite periphery typical of parasite plasma membrane (PPM), parasitophorous vacuole (PV) or parasitophorous vacuolar membrane (PVM) by green fluorescent protein (GFP)-tagging approach (Heiber et al., 2013). More recently, GFP-tagged PF3D7_1404900 protein was localized in merozoites revealing a cytosolic staining pattern with accumulation at the apical pole (Wilcke, 2018). Plasmodium falciparum Merozoite Surface Protein 7 (PfMSP7) is involved in erythrocyte invasion and the protein is currently under study as a potential malaria vaccine candidate (Raj et al., 2014). PF3D7_0308300 is another novel protein associated with merozoite invasion and was localized to the parasite surface (Hu et al., 2010) by expressing the GFP-tagged protein ectopically in P. falciparum (Treeck et al., 2006). Interestingly, PF3D7_0308300 protein is one of the P. falciparum antigens on the Malian protein microarray that met the inclusion criteria during the assessment of anti-malaria antibody responses (Helb, 2015).
The basis for the use of these antigens with known subcellular localization as comparators for PfATRP is to determine how the intracellular localization of these antigens in released merozoites modulates their respective plasma immunoreactivity patterns (Dreyer et al., 2012). We assessed the levels of antibody responses against four different P. falciparum merozoite antigens (PfMSP7, PF3D7_0308300, PF3D7_1404900, and PfATRP) across areas of varying malaria transmission intensity (Kintampo>Navrongo>Accra); (Klinkenberg et al., 2008; Kasasa et al., 2013). The data showed that the immunoreactivity patterns for both PfMSP7 and PF3D7_1404900 varied significantly with transmission intensity when comparisons were made between Kintampo and Navrongo, Kintampo and Accra (Figures 6A,B). However, there were no significant differences in the response patterns for PfMSP7 and PF3D7_1404900 between Navrongo and Accra (Figures 6A,B). Also, there was no discernable transmission intensity effect on the levels of PF3D7_030800 and PfATRP plasma antibodies (Figures 6C,D) which indicates that both antigens are not likely to be considered as a potential biomarkers.
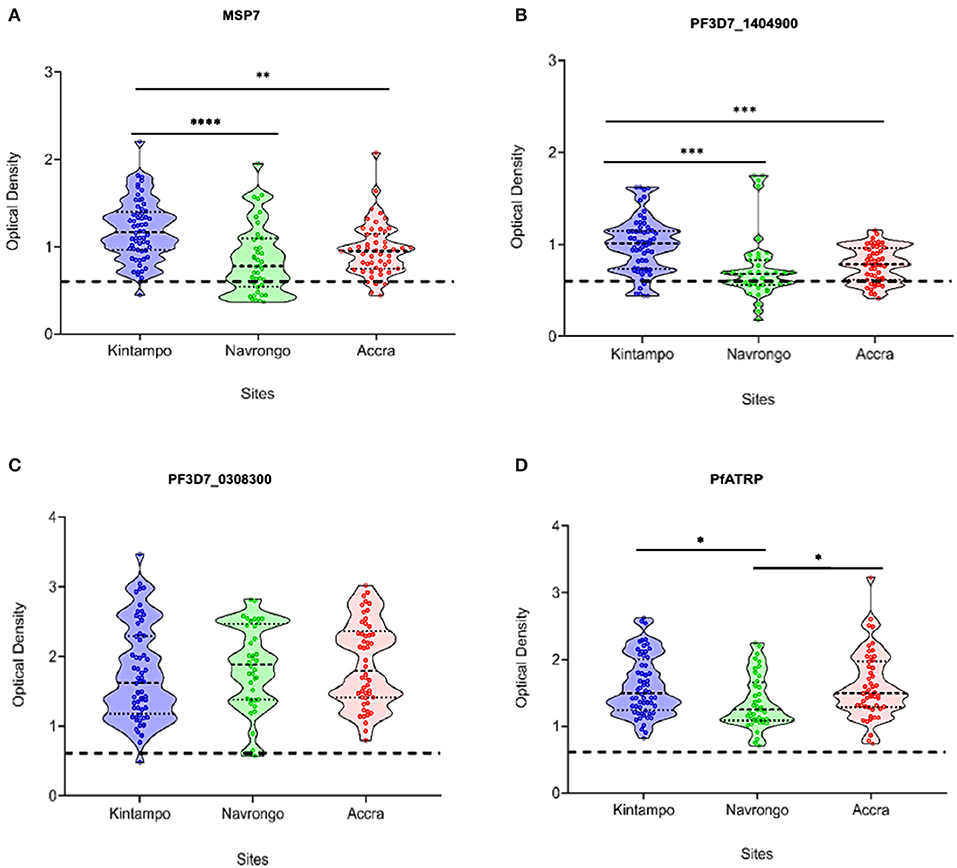
Figure 6. PfATRP is recognized by antibodies from individuals naturally exposed to P. falciparum malaria. (A–D) Violin plots showing the levels of antibody responses against the four different antigens (PfMSP7, PF3D7_1404900, PF3D7_0308300, and PfATRP) across areas of varying malaria transmission intensity (Kintampo>Navrongo>Accra). Statistical analyses were performed using One-way ANOVA and where significant, Tukey's multiple comparison test was used to compute for pairewise comparisons. All graphs were performed on GraphPad Prism v. 8.01. The black dotted-lines in the graphs depict the cut-off of seropositivity defined using the mean + 3 standard deviations of the OD values obtained for the naïve control. Significant statistical differences were shown. *p = 0.03, **p = 0.002, ***p < 0.001, ****p < 0.0001. ns: non-significant. Sample sizes: Kintampo (n = 60); Navrongo (n = 44); Accra (n = 57) and naïve control (n = 6). The ELISA data shown in this figure is a representation of the means of two independent experiments.
Consequently, the observed differences in the response patterns for these antigens is consistent with the proposition that differences in the rate of antibody acquisition to merozoite antigens differ due to differences in immunogenicity and protein subcellular localization (McCallum et al., 2017). Moreover, differences in the responses between the antigens could also be linked to the fact that different P. falciparum antigens elicit antibody responses with different magnitudes and kinetics (Elliott et al., 2014; Stanisic et al., 2015).
PfATRP Human Antibody Response Does Not Correlate Significantly With Age
In order to evaluate the applicability of α-PfATRP human antibody during serological screening of a population, we used age-stratified plasma samples across all sites for the analysis of PfMSP7, PF3D7_0308300, PF3D7_1404900, and PfATRP responses. The data showed that none of these antigens tested correlated significantly with age (Figures 7A–D). Furthermore, we substantiated the ELISA-based analysis for PfATRP response by performing immunoblotting experiments. The non-reactive control did not detect recombinant PfATRP (Figure S6i) while the reactive control plasma from malaria-exposed healthy adults (Figure S6ii) and the individual immunoreactive pools from children across the three malaria endemic sites detected recombinant PfATRP (Figures S6iii-v). Thus, our data on the immunoreactivity profiles of PfATRP presents it as an immunogenic antigen.
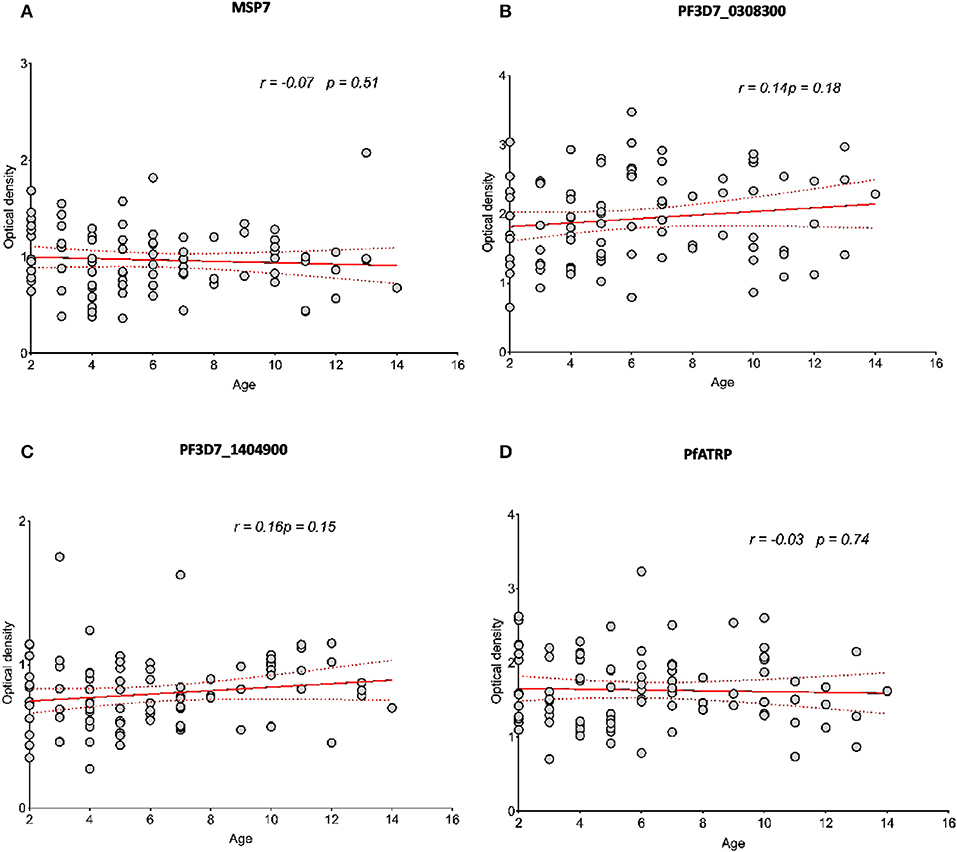
Figure 7. PfATRP human antibody response does not correlate significantly with age. (A–D) The scattered plots represents the pooled and age-matched data analysis for the antibody response patterns across the three malaria endemic sites for all antigens tested. The red lines depict the linear regression lines (plain lines) with their associated 95% confidence intervals (dotted lines). The Pearson correlation test was used to compute the coefficients of correlation between the measured variables. All statistical analyses were performed using GraphPad Prism v. 8.01.
Discussion
The availability of published P. falciparum genome (Gardner et al., 2002), transcriptome (Bozdech et al., 2003) and proteomic datasets (Bowyer et al., 2011) makes the identification and functional characterization of novel parasite proteins feasible, yet most P. falciparum genes/proteins remain to be characterized functionally. Two recent large-scale genetic screens for blood-stage phenotypes in P. berghei and P. falciparum have added a first layer of functional data for most genes in the genome (Schwach et al., 2015), but while this represents a significant step forward, detailed localization, and biochemical studies of individual proteins will still be required to illuminate their functions. We identified a novel P. falciparum, Armadillo-Type Repeat Protein (PfATRP: PF3D7_0410600/PFD0525w) using bioinformatics portals, which revealed some predicted structural characteristics of the protein. The encoding gene, PfATRP, was suggested to be essential for blood-stage parasite growth in a recent piggyBac transposon saturation-level mutagenesis screen (Zhang et al., 2018). Generally, ARM proteins are known to be versatile in their functions, and the classification of ARM proteins has been quite challenging given that some of the current annotations of armadillo repeats are incomplete or may be incorrect (Gul et al., 2017), due in part to difficulties in distinguishing between armadillo repeat types and the high similarity with Huntingtin, elongation factor 3, protein phosphatase 2A, and the yeast kinase TOR1 (HEAT) repeats (Kippert and Gerloff, 2009). Detailed functional phenotyping of individual ARM proteins is therefore important.
We used α-PfATRP rabbit antibody during schizont arrest assays/in-solution or non-permeabilized IFAs and showed that PfATRP is not localized on the merozoite surface. Colocalization analysis performed during permeabilized IFAs in both asexual and sexual stages suggest that PfATRP may exhibit a dynamic subcellular localization. The identification of PfATRP that lacks membrane attachment motifs as a likely component of the IMC/microtubules in the parasite was unexpected because previously characterized ARM-containing proteins (Absalon et al., 2016; Jacot et al., 2016; Mitra et al., 2016) were all localized to the apical pole. However, a previous report supports the possibility that ARM repeats could mediate membrane association (Drechsel et al., 2010). Therefore, immuno-electron microscopy analysis will be required to determine the precise subcellular localization of PfATRP in the parasite.
PfATRP and other ARM proteins belong to the β-catenin family that interacts with dynein and appears to tether microtubules at adherens junctions in epithelial cells (Ligon et al., 2001). In gametocytes, we observed colocalization of PfATRP with TAT1 which supports an association with microtubules. Hence, the localization of PfATRP in the IMC and its associated microtubules may represent the poorly described subcellular organelle interplay in the parasite. Although, it is noteworthy that the IMC-microtubular interplay may have resulted during lateral expansion of the IMC around the girth of the parasite when it associates with microtubules (Schneider et al., 2017).
The IMC is a cisternal compartment that is assembled under the plasma membrane of Plasmodium parasites, in merozoites, sporozoites, ookinetes, and gametocytes (Schneider et al., 2017). The IMC plays an important structural role in the cellular remodeling events associated with gametocyte elongation (Dearnley et al., 2012). This is consistent with studies that indicated the roles which specific IMC proteins play in cell morphology (Tremp and Dessens, 2011). During gametocyte development, elongation is driven by a network of microtubules that assemble under the IMC (Schneider et al., 2017). Importantly, the gametocyte IMC has a stage-specific function that may involve a poorly defined set of proteins (Schneider et al., 2017) which have been classified based on their structural features into alveolins, non-alveolins, and multi-transmembrane proteins (Kono et al., 2012). However, a member of the glideosome assembly, PfGAP50 is known to be recruited to the periphery of gametocytes and appears to be coordinated with the laying down of microtubules (Dearnley et al., 2012). There are a number of parasite proteins that are recruited to the IMC via protein-protein interactions (Ramirez and Lowe, 2009; Kono et al., 2012; Schneider et al., 2017). Although putative PfATRP-interacting partner proteins have been reported previously by proteome-wide yeast-2-hybrid screens (LaCount et al., 2005), the relevance of this in the context of PfATRP membrane attachment has not been evaluated. PfATRP may not be palmitoylated based on previous studies on P. falciparum Palmitome (Jones et al., 2012) which leaves avenues for future studies since other parasite proteins have been shown to deploy lipid modification mechanisms for membrane attachment (Wetzel et al., 2015).
The identification of Plasmodium species-specific biomarkers is important in areas where multi-species infections occur. This provides information that will guide the evaluation of control measures that targets a single species, which might impact on the transmission and immunological profiles of other co-endemic species (Herman et al., 2018). The understanding of immunity to infections can be deduced from studies on human serological responses to different Plasmodium species. There is increasing interest in the use of antibodies specific for merozoite antigens as serological biomarkers of Plasmodium exposure or as biomarkers of protective immunity (Osier et al., 2014; Helb et al., 2015; Stanisic et al., 2015; van den Hoogen L. L et al., 2019). Although, immuno-epidemiological studies have recorded repeated conflicting data on responses to the same antigen in different areas (Riley et al., 1992; Høgh et al., 1995; Al-Yaman et al., 1996; Branch et al., 1998; Cavanagh et al., 2004), large-scale sero-epidemiological analysis performed on a microarray platform has always provided useful information. A previous microarray-based analysis of plasma samples revealed that antibodies to intracellular proteins were better viewed as biomarkers of past infection or indicators of enhanced parasite killing in protected individuals, and not as evidence for vaccine potential (Crompton et al., 2010). PfATRP may be an intracellular antigen that is immunogenic but its potential application as a biomarker is not supported by the data in this study. Therefore, we presume that the naturally acquired, α-PfATRP human antibody responses observed in malaria exposed children could be attributed to high biomass of P. falciparum parasites during advanced infections that results in the lysis of non-invasive merozoites which expose their contents to the immune system.
Altogether, this work presents the first cell biological and immunological assessment of PfATRP that presents new opportunities in targeting protein localization for anti-infective therapy. Further functional investigation on the structural determinant mediating the recruitment of PfATRP to membrane localization is required.
Materials and Methods
PfATRP Domain Identification and Homology Modeling
The amino acid sequence of PfATRP was submitted to the Eukaryotic Linear Motif (ELM) portal as described previously (Dinkel et al., 2015). This platform analyzes user-submitted protein sequences by scanning for matches to structural motifs that are already curated in the database. A predicted 3D-model for full length PfATRP was determined using both Phyre 2 and I-TASSER protein homology modeling platform for structural predictions (Roy et al., 2010; Kelley et al., 2015).
Parasite Culture and Synchronization
P. falciparum strains (3D7 and NF54) were cultured in normal human O+ erythrocytes based on the methods described previously (Jensen and Trager, 1978). The parasites were tightly synchronized by routine sorbitol treatments and Percoll-alanine gradient centrifugation (Kanaani and Ginsburg, 1989; Awandare et al., 2018).
Quantitative RT-PCR Analysis
Total RNA was extracted from 3D7 parasite pellets at 8-h intervals using the phenol-chloroform method after homogenization with TRIzol Reagent (Ambion/Life Technologies, Carlsbad, California). Quantitative mRNA transcript levels for the control, Erythrocyte Binding Antigen 175 (EBA-175) was determined using the primer set (forward primer (FP): TTCGTGATGAGTGGTGGAAA and reverse primer (RP): GGCAATAATCATCACCCCATT) (Lopaticki et al., 2011). PfATRP transcripts levels were also determined using the designed primers (FP: ACGAAATATGCAGACGGGACT and RP: CGAAGTTACGAACGGCTTCATT). Analysis was performed on a Quant Studio 5, Real-Time PCR system (Applied Biosystems) using the Luna Universal, One-Step RT-qPCR Kit (New England Biolabs, Inc.) following the manufacturer's protocol. The mRNA transcript levels were normalized to the expression of the 60S ribosomal protein L18 (2−ΔCt).
Gene Synthesis and Sub-cloning
A codon harmonized, full-length gene of 981 bp, encoding 326 amino acids (Met-1 to Gly-326) of PfATRP from P. falciparum with a C-terminal Hexa-histidine (6x His) tag was synthesized and sub-cloned by Bio Basic (Canada) into a T7 promoter Escherichia coli (E. coli) expression vector (pET-24b) with Nde1 and Xho1 restriction sites to obtain PfATRP plasmid for enhanced expression in E. coli. Also codon optimized genes for PF3D7_1404900 (84-894 bp), PfMSP7 (93-1056 bp), and PF3D7_0308300 (1-1014 bp), encoding 269 amino acids (Asn-29 to Leu-297); 351 amino acids (Asn-32 to Met-351) and 337 amino acids (Met-1 to Asn-337), respectively were synthesized and subcloned in pET-24b vector with Nde1 and Xho1 restriction sites for expression in E. coli.
Recombinant Protein Production
PfATRP, plasmid was transformed into BL21-CodonPlus (DE3)-RIPL E. coli competent cell and cultures were induced at an optical density of 0.6 with 1 mM Isopropyl β-D-1-thiogalactopyranoside (IPTG) at 25°C for 12–18 h. The expressed recombinant protein was purified by immobilized metal affinity chromatography (IMAC) using Ni-NTA resin (Qiagen, USA). The purified protein was buffer-exchanged against PBS using 10 kDa cut-off centrifugal filters, then further purified using size exclusion chromatography (GE, Superdex-200 increase 10/300 GL column). The purity of the recombinant protein was assessed by SDS-PAGE and staining with Coomassie brilliant blue dye and the identity of the protein was confirmed by Liquid Chromatography-Mass Spectrometry (LC-MS). Similarly, the control antigens (PF3D7_1404900, PfMSP7 and PF3D7_0308300 used in this study were produced in an ongoing research project under WACCBIP, University of Ghana's recombinant protein production initiative.
Immunoblotting
New Zealand rabbits were immunized with the purified rPfATRP protein by Bio Basic, Canada and PfATRP-specific rabbit antibody was purified from the crude sera obtained on day 70 post immunization, as described previously (Boyle et al., 2014). The specificity of α-PfATRP rabbit antibody was confirmed by immunoblotting using 3D7 schizont lysates.
Schizont Arrest Assays
We performed schizont arrest assays as described previously (Raj et al., 2014). Briefly, 3D7 cultures were subjected to two rounds of sorbitol synchronization at the ring stage (4% parasitemia). The parasites were grown to segmented schizonts and were individually incubated with α-PfATRP rabbit antibody (100 μg/ml) and E64 (10 μM) for 12 h. We scored the effect of egress inhibition by measuring the number of newly formed rings instead of residual schizonts and made comparison with the untreated control.
Immunofluorescence Staining of Parasites
Smears from synchronized P. falciparum 3D7 and NF54 cultures were made on glass slides and fixed in pre-chilled methanol (−20°C). The slides were air-dried and blocked using 3% BSA in PBS overnight at 4°C. The slides were incubated at room temperature (~25°C) for an hour with different antibodies at the following dilutions: α-PfATRP rabbit antibody (1:100); BEI Resources MRA-897A anti-P. falciparum apical merozoite antigen-1 (α-PfAMA1) mouse monoclonal antibody (1:100), anti-P. falciparum merozoite surface protein 1 (α-PfMSP1) mouse monoclonal antibody (1:100); (Guevara Patiño et al., 1997), anti-P. falciparum myosin tail interacting protein (α-PfMTIP) rat antibodies (1:100); (Jones et al., 2006), BEI Resources MRA-316A anti-Plasmodium falciparum 48/45-kDa Gamete Surface Protein (Pfs48/45) mouse antibody (1:100); (Miller, L. H. and A. Saul, Personal Communication) and α-Tubulin acetyl transferase 1 (α-TAT 1) mouse antibody (1:10). The details for these marker antibodies have been described in the acknowledgment section. After the incubation period, the slides were washed and incubated with secondary antibodies conjugated with FITC, Alexa Fluor 488 or Alexa Fluor 594. The slides were washed and mounted with VECTASHIELD mounting medium (Burlingame, CA) with 4′, 6′-diamidino-2-phenylindole (DAPI) and were viewed on a fluorescence microscope (Olympus BX-41, Hamamatsu Photonics K.K, Japan). The IFA images captured were processed using the Fiji-Image J software (National Institutes of Health, USA).
In-solution IFA was performed by co-labeling non-permeabilized segmented schizonts with α-merozoite surface protein 1 mouse antibody (PfMSP-1) and α-PfATRP rabbit antibody (1:100) as described previously (Raj et al., 2014). The nulcei were stained with Hoechst (ThermoFisher Scientific) and the labeled parasites were mounted on glass slides using VECTASHIELD mounting medium (Burlingame, CA) and images were captured and processed as described above.
Human Plasma Samples and Ethical Approval
Ethical approval was obtained from the ethics committees of the Noguchi Memorial Institute for Medical Research, University of Ghana, the Ghana Health Service, Navrongo Health Research Center, and Kintampo Health Research Center and all research was performed in accordance with the prescribed guidelines/regulations. Plasma samples were collected after obtaining informed consent from parents/guardians of the participating children. Blood used in this study for culturing was obtained from healthy donors with informed consent.
The human plasma samples used in this study were obtained from children (2–14 years) resident in Kintampo, Navrongo and Accra and the samples were collected during the rainy seasons between September 2011 and September 2013 (Mensah-Brown et al., 2015, 2017). The ages of children recruited in Kintampo were not significantly different from those in Accra and Navrongo (P = 0.10 and P = 0.09, respectively); (Mensah-Brown et al., 2019). Also, children recruited in Accra were significantly older than those recruited in Navrongo (P = 0.002), (Mensah-Brown et al., 2019). Malaria transmission intensity in the selected sample sites have been measured by the entomological inoculation rates and was highest in Kintampo (>250 infective bites/person per year), followed by Navrongo (<250 infective bites/person per year), and lowest in Accra (<50 infective bites/person per year); (Klinkenberg et al., 2008; Owusu-Agyei et al., 2009; Kasasa et al., 2013).
Enzyme Linked Immunosorbent Assay
Ten micrograms (10μg) of PfATRP, PF3D7_1404900, PfMSP7 and PF3D7_0308300 soluble recombinant proteins in phosphate buffered saline, pH 7.2 were individually coated between 96-well microtiter plates and incubated overnight at 4°C. The plates were washed thrice with Phosphate Buffered Saline (PBS) containing 0.05% Tween 20 (PBST), and blocked with 3% BSA (Bovine Serum Albumin) in PBS overnight at 4°C. After the washing steps, the plates were incubated with plasma samples (1:50 dilution) from anonymized malaria-infected children for an hour at 37°C. The washing steps were repeated, and the plates were incubated with goat anti-Human IgG (H+L) horseradish peroxidase conjugated Secondary antibody (ThermoFisher Scientific #31410), (1:5000 dilution) at 37°C for an hour. After the incubation period, the plates were washed five times with PBST and PBS, and 3, 3′, 5, 5′-tetramethylbenzidine (TMB) was used to develop the reaction during a 20 min incubation in the dark. Optical Density was read at 450 nm using a VARIOSKAN LUX multi-mode microplate reader (Thermo Fischer Scientific, USA). Malaria-naïve European donor samples were used as experimental controls. The cut-off value for ELISA was calculated based on the readouts for the naïve control.
Data Availability Statement
All datasets generated for this study are included in the article/Supplementary Material.
Ethics Statement
The studies involving human participants were reviewed and approved by Ethical approval was obtained from the ethics committees of the Noguchi Memorial Institute for Medical Research, University of Ghana, the Ghana Health Service, Navrongo Health Research Center, and Kintampo Health Research Center and all research was performed in accordance with the prescribed guidelines/regulations. The samples were collected after obtaining informed consent from parents/guardians of the participating children. Blood used in this study for culturing was obtained from healthy donors with informed consent. Written informed consent to participate in this study was provided by the participants' legal guardian/next of kin.
Author Contributions
EA and GA designed the study with contributions from JR. EA, PI, GO, PN, EQ, LT, MA, RA-D, OA, and HM-B performed experiments, analyzed data, and contributed during the preparation of figures. EA and GA wrote the manuscript.
Funding
This work was supported by funds from a World Bank African Centers of Excellence grant (ACE02-WACCBIP: GA) and a DELTAS Africa grant (DEL-15-007: GA). GO and EQ were supported by WACCBIP-DELTAS Masters fellowship while PI, OA, PN, RA-D, and LT were supported by WACCBIP-World Bank ACE Masters/Ph.D. fellowships, respectively. HM-B was supported by UK Royal Society Leverhule African award scheme (grant AA110050 to GA and David Conway). EA is currently a Crick African Network (CAN) Fellow and the research was supported by WACCBIP-DELTAS postdoctoral fellowship. The DELTAS Africa Initiative is an independent funding scheme of the African Academy of Sciences (AAS)'s Alliance for Accelerating Excellence in Science in Africa (AESA) and supported by the New Partnership for Africa's Development Planning and Coordinating Agency (NEPAD Agency) with funding from the Wellcome Trust (107755/Z/15/Z: GA) and the UK government. The views expressed in this publication are those of the author(s) and not necessarily those of AAS, NEPAD Agency, Wellcome Trust or the UK government. Funding from the National Institute of Allergy and Infectious Diseases, National Institutes of Health (grant 1R01AI102848 to GA) is also acknowledged.
Conflict of Interest
The authors declare that the research was conducted in the absence of any commercial or financial relationships that could be construed as a potential conflict of interest.
Acknowledgments
We thank Dr. Aniweh Yaw for sourcing BEI Resources marker antibodies and α-TAT1 mouse antibody from collaborators. Dr. Anthony Holder at Francis Crick Institute, UK is acknowledged for providing α-MSP119 antibody. MRA-897A monoclonal Anti-Plasmodium Apical Membrane Antigen 1 was contributed by Alan W. Thomas through the National Institute for Allergy and Infectious Diseases (NIAID) BEI Resources. MRA-316A monoclonal Antibody IIC5B-10-1 anti-Plasmodium falciparum 48/45-kDa Gamete Surface Protein (Pfs48/45), (produced in vitro), was obtained through BEI Resources, NIAID, NIH: contributed by Louis H. Miller and Alan Saul. Anti-MTIP mouse antibody was generously provided by JR. MA-R provided plasma samples from malaria-exposed adults in Accra. Mr. Kofi B. Prempeh-Fordjour rendered technical assistance. The control recombinant antigens (PF3D7_1404900, PfMSP7 and PF3D7_0308300) were sourced from an ongoing research project under WACCBIP, University of Ghana's recombinant protein production initiative. Mr. Femi Adepoju and Mr. Stanley Iro assisted in manuscript typesetting.
Supplementary Material
The Supplementary Material for this article can be found online at: https://www.frontiersin.org/articles/10.3389/fcimb.2020.00114/full#supplementary-material
Figure S1. Homology-based modeling for the prediction of PfATRP structure. 3D-model for the full length PfATRP from (A,B) Phyre and I-TASSER protein structure prediction portals.
Figure S2. Codon-optimized gene sequences. PfATRP (i) and the control genes, PF3D7_1404900 (ii), MSP7 (iii) PF3D7_0308300 (iv) provided by BioBasic, Canada and GeneScript, USA.
Figure S3. Expression and purification of recombinant PfATRP. (A) PfATRP induced and control lysates were analyzed on SDS-PAGE. Red arrow shows induced band (32 kDa). (B) Purification by Ni-NTA chromatography and Size Exclusion Chromatography (SEC) showing the monomeric PfATRP. The protein samples were ran along side with color pre-stained protein standard (P7712S) broad range (11–245 kDa).
Figure S4. Purification of control recombinant proteins. the control antigens, (A) PfMSP7 (50 kDa), (B) PF3D7_0308300 (40 kDa), and (C) PF3D7_1404900 (30 kDa) were expressed and purified to apparent homogeneity. The identities of the control recombinant proteins were confirmed by immunoblotting using α-6xHistidine mouse monoclonal antibody.
Figure S5. Co-immunostaining of anti-PfATRP with surface marker antibodies. Dual IFAs for PfATRP were performed in both asexual and sexual parasite stages using surface marker antibodies. (A) Slides were prepared for early/late schizont stage and probed with α-PfATRP rabbit antibody (red); (1:100) and α-PfMSP1 mouse antibody (1:100); (green) which showed that PfATRP does not colocalize with PfMSP1 on the merozoite surface. (B) Similarly, dual IFAs were performed for gametocytes using α-PfATRP rabbit antibody (1:100), (green) and α-Pfs48/45 mouse antibody (red); (1:100) which showed that PfATRP is not localized on the surface of gametocytes. White lines on IFA images represent scale bar.
Figure S6. Anti-PfATRP antibody from malaria-exposed children detects recombinant PfATRP. (i) Immunoblots showed that the non-reactive control plasma (NHS) did not detect recombinant PfATRP. (ii–v) The reactive control plasma from malaria-exposed healthy adults, and the individual immunoreactive pools from children across the three endemic sites detected recombinant PfATRP.
Table S1. The identity of recombinant PfATRP was confirmed by LC-MS analysis. Purified recombinant PfATRP was excised from SDS-PAGE gel and subjected to trypsin digestion followed by LC-MS analysis. The tryptic peptides obtained were blasted against Plasmodium falciparum database and the unique peptides, molecular weight, sequence coverage and MS/MS counts obtained confirm the identity of the PfATRP (Q9U0I2).
References
Absalon, S., Robbins, J. A., and Dvorin, J. D. (2016). An essential malaria protein defines the architecture of blood-stage and transmission-stage parasites. Nat. Commun. 7:11449. doi: 10.1038/ncomms11449
Ahlborg, N., Iqbal, J., Björk, L., Ståhl, S., Perlmann, P., and Berzins, K. (1996). Plasmodium falciparum: differential parasite growth inhibition mediated by antibodies to the antigens Pf332 and Pf155/RESA. Exp. Parasitol. 82, 155–163. doi: 10.1006/expr.1996.0020
Aikawa, M., Miller, L. H., Rabbege, J. R., and Epstein, N. (1981). Freeze-fracture study on the erythrocyte membrane during malarial parasite invasion. J. Cell Biol. 91, 55–62. doi: 10.1083/jcb.91.1.55
Al-Yaman, F., Genton, B., Kramer, K. J., Chang, S. P., Hui, G. S., Baisor, M., et al. (1996). Assessment of the role of naturally acquired antibody levels to Plasmodium falciparum merozoite surface protein-1 in protecting Papua New Guinean children from malaria morbidity. Am. J. Trop. Med. Hyg. 54, 443–448. doi: 10.4269/ajtmh.1996.54.443
Assossou, O., Besson, F, Rouault, J. P, Persat, F, Brisson, C., Duret, L., et al. (2003). Subcellular localization of 14-3-3 proteins in Toxoplasma gondii tachyzoites and evidence for a lipid raft-associated form. FEMS Microbiol. Lett. 224, 161–168. doi: 10.1016/S0378-1097(03)00479-8
Awandare, G. A., Nyarko, P. B., Aniweh, Y., Ayivor-Djanie, R., and Stoute, J. A. (2018). Plasmodium falciparum strains spontaneously switch invasion phenotype in suspension culture. Sci. Rep. 8:5782. doi: 10.1038/s41598-018-24218-0
Baum, J., Gilberger, T.-W., Frischknecht, F., and Meissner, M. (2008). Host-cell invasion by malaria parasites: insights from Plasmodium and Toxoplasma. Trends Parasitol. 24, 557–563. doi: 10.1016/j.pt.2008.08.006
Baum, J., Richard, D., Healer, J., Rug, M., Krnajski, Z., Gilberger, T.-W., et al. (2006). A conserved molecular motor drives cell invasion and gliding motility across malaria life cycle stages and other apicomplexan parasites. J. Biol. Chem. 281, 5197–5208. doi: 10.1074/jbc.M509807200
Bergmann-Leitner, E. S., Duncan, E. H., and Angov, E. (2009). MSP-1p42-specific antibodies affect growth and development of intra-erythrocytic parasites of Plasmodium falciparum. Malar. J. 8:183. doi: 10.1186/1475-2875-8-183
Bowyer, P. W., Simon, G. M., Cravatt, B. F., and Bogyo, M. (2011). Global profiling of proteolysis during rupture of Plasmodium falciparum from the host erythrocyte. Mol. Cell Proteomics 10:M110.001636. doi: 10.1074/mcp.M110.001636
Boyle, M. J., Langer, C., Chan, J.-A., Hodder, A. N., Coppel, R. L., Anders, R. F., et al. (2014). Sequential processing of merozoite surface proteins during and after erythrocyte invasion by Plasmodium falciparum. Infect. Immun. 82, 924–936. doi: 10.1128/IAI.00866-13
Bozdech, Z., Llinás, M., Pulliam, B. L., Wong, E. D., Zhu, J., and DeRisi, J. L. (2003). The transcriptome of the intraerythrocytic developmental cycle of Plasmodium falciparum. PLoS Biol. 1:e5. doi: 10.1371/journal.pbio.0000005
Branch, O. H., Udhayakumar, V., Hightower, A. W., Oloo, A. J., Hawley, W. A., Nahlen, B. L., et al. (1998). A longitudinal investigation of IgG and IgM antibody responses to the merozoite surface protein-1 19-kiloDalton domain of Plasmodium falciparum in pregnant women and infants: associations with febrile illness, parasitemia, and anemia. Am. J. Trop. Med. Hyg. 58, 211–219. doi: 10.4269/ajtmh.1998.58.211
Cavanagh, D. R., Dodoo, D., Hviid, L., Kurtzhals, J. A., Theander, T. G., Akanmori, B. D., et al. (2004). Antibodies to the N-terminal block 2 of Plasmodium falciparum merozoite surface protein 1 are associated with protection against clinical malaria. Infect. Immun. 72, 6492–6502. doi: 10.1128/IAI.72.11.6492-6502.2004
Coates, J. C. (2003). Armadillo repeat proteins: beyond the animal kingdom. Trends Cell Biol. 13, 463–471. doi: 10.1016/S0962-8924(03)00167-3
Cowman, A. F., Tonkin, C. J., Tham, W.-H., and Duraisingh, M. T. (2017). The molecular basis of erythrocyte invasion by malaria parasites. Cell Host Microbe 22, 232–245. doi: 10.1016/j.chom.2017.07.003
Crompton, P. D., Kayala, M. A., Traore, B., Kayentao, K., Ongoiba, A., Weiss, G. E., et al. (2010). A prospective analysis of the Ab response to Plasmodium falciparum before and after a malaria season by protein microarray. Proc. Natl. Acad. Sci. U.S.A. 107, 6958–6963. doi: 10.1073/pnas.1001323107
Das, S., Hertrich, N., Perrin, A. J., Withers-Martinez, C., Collins, C. R., Jones, M. L., et al. (2015). Processing of Plasmodium falciparum merozoite surface protein activates a spectrin-binding function enabling parasite egress from RBCs. Cell Host Microbe 18, 433–44. doi: 10.1016/j.chom.2015.09.007
Dearnley, M. K., Yeoman, J. A., Hanssen, E., Kenny, S., Turnbull, L., Whitchurch, C. B., et al. (2012). Origin, composition, organization and function of the inner membrane complex of Plasmodium falciparum gametocytes. J. Cell Sci. 125, 2053–2063. doi: 10.1242/jcs.099002
Dinkel, H., Van Roey, K., Michael, S., Kumar, M., Uyar, B., Altenberg, B., et al. (2015). ELM 2016–data update and new functionality of the eukaryotic linear motif resource. Nucleic Acids Res. 44, D294–D300. doi: 10.1093/nar/gkv1291
Douglas, A. D., Williams, A. R., Knuepfer, E., Illingworth, J. J., Furze, J. M., Crosnier, C., et al. (2013). Neutralization of Plasmodium falciparum Merozoites by Antibodies against PfRH5. J. Immunol. 192, 24–258. doi: 10.4049/jimmunol.1302045
Drechsel, G., Bergler, J., Wippel, K., Sauer, N., Vogelmann, K., and Hoth, S. (2010). C-terminal armadillo repeats are essential and sufficient for association of the plant U-box armadillo E3 ubiquitin ligase SAUL1 with the plasma membrane. J. Exp. Bot. 62, 775–785. doi: 10.1093/jxb/erq313
Dreyer, A. M., Matile, H., Papastogiannidis, J. K., Favuzza, P., Voss, T. S., Wittlin, S., et al. (2012). Passive immunoprotection of Plasmodium falciparum-infected mice designates the CyRPA as candidate malaria vaccine antigen. J. Immunol. 188, 6225–6237. doi: 10.4049/jimmunol.1103177
Elliott, S. R., Fowkes, F. J., Richards, J. S., Reiling, L., Drew, D. R., and Beeson, J. G. (2014). Research priorities for the development and implementation of serological tools for malaria surveillance. F1000prime rep. 6:100. doi: 10.12703/P6-100
Fu, H., Subramanian, R. R., and Masters, S. C. (2000). 14-3-3 proteins: structure, function, and regulation. Annu. Rev. Pharmacol. Toxicol. 40, 617–647. doi: 10.1146/annurev.pharmtox.40.1.617
Gardner, M. J., Hall, N., Fung, E., White, O., Berriman, M., Hyman, R. W., et al. (2002). Genome sequence of the human malaria parasite Plasmodium falciparum. Nature 419, 498–511. doi: 10.1038/nature01097
Gasteiger, E. G. A., Duvaud, S., Wilkins, M. R., Appel, R. D., and Bairoch, A. (2005). “Protein Identification and Analysis Tools on the ExPASy Server,” in The Proteomics Protocols Handbook, ed J. M. Walker (Totowa, NJ: Humana Press), 571–607.
Goodyer, I. D., Pouvelle, B., Schneider, T. G., Trelka, D. P., and Taraschi, T. F. (1997). Characterization of macromolecular transport pathways in malaria-infected erythrocytes. Mol. Biochem. Parasitol. 87, 13–28. doi: 10.1016/S0166-6851(97)00039-X
Guevara Patiño, J. A., Holder, A. A., McBride, J. S., and Blackman, M. J. (1997). Antibodies that inhibit malaria merozoite surface Protein-1 processing and erythrocyte invasion are blocked by naturally acquired human antibodies. J. Exp. Med. 186, 1689–1699. doi: 10.1084/jem.186.10.1689
Gul, I. S., Hulpiau, P., Saeys, Y., and Van Roy, F. (2017). Metazoan evolution of the armadillo repeat superfamily. Cell. Mol. life Sci. 74, 525–541. doi: 10.1007/s00018-016-2319-6
Heiber, A., Kruse, F., Pick, C., Grüring, C., Flemming, S., Oberli, A., et al. (2013). Identification of new PNEPs indicates a substantial non-PEXEL exportome and underpins common features in Plasmodium falciparum protein export. PLoS Pathog. 9:e1003546. doi: 10.1371/journal.ppat.1003546
Helb, D. A. (2015). Anti-malarial antibody responses and application for assessing malaria exposure (Ph.D. dissertation), University of California, Berkeley, CA, United States.
Helb, D. A., Tetteh, K. K. A., Felgner, P. L., Skinner, J., Hubbard, A., Arinaitwe, E., et al. (2015). Novel serologic biomarkers provide accurate estimates of recent Plasmodium falciparum exposure for individuals and communities. Proc. Natl. Acad. Sci. U.S.A. 112, E4438–E4447. doi: 10.1073/pnas.1501705112
Herman, L. S., Fornace, K., Phelan, J., Grigg, M. J., Anstey, N. M., William, T., et al. (2018). Identification and validation of a novel panel of Plasmodium knowlesi biomarkers of serological exposure. PLoS Negl. Trop. Dis. 12:e0006457. doi: 10.1371/journal.pntd.0006457
Hill, D.L., Eriksson, E.M., Carmagnac, A.B., Wilson, D.W., Cowman, A.F., Hansen, S.D., et al. (2012). Efficient measurement of opsonizing antibodies to Plasmodium falciparum merozoites. PLoS ONE. 7:e51692. doi: 10.1371/journal.pone.0051692
Høgh, B., Marbiah, N. T., Burghaus, P. A., and Andersen, P. K. (1995). Relationship between maternally derived anti-Plasmodium falciparum antibodies and risk of infection and disease in infants living in an area of Liberia, west Africa, in which malaria is highly endemic. Infect. Immun. 63, 4034–4038. doi: 10.1128/IAI.63.10.4034-4038.1995
Hu, G., Cabrera, A., Kono, M., Mok, S., Chaal, B. K., Haase, S., et al. (2010). Transcriptional profiling of growth perturbations of the human malaria parasite Plasmodium falciparum. Nat. Biotechnol. 28, 91–98. doi: 10.1038/nbt.1597
Hülsken, J., Birchmeier, W., and Behrens, J. (1994). E-cadherin and APC compete for the interaction with beta-catenin and the cytoskeleton. J. Cell Biol. 127, 2061–2069. doi: 10.1083/jcb.127.6.2061
Jacot, D., Tosetti, N., Pires, I., Stock, J., Graindorge, A., Hung, Y.-F., et al. (2016). An apicomplexan actin-binding protein serves as a connector and lipid sensor to coordinate motility and invasion. Cell Host Microbe 20, 731–743. doi: 10.1016/j.chom.2016.10.020
Jensen, J. B., and Trager, W. (1978). Plasmodium falciparum in culture: establishment of additional strains. Am. J. Trop. Med. Hyg. 27, 743–746. doi: 10.4269/ajtmh.1978.27.743
Jones, M. L., Collins, M. O., Goulding, D., Choudhary, J. S., and Rayner, J. C. (2012). Analysis of protein palmitoylation reveals a pervasive role in Plasmodium development and pathogenesis. Cell Host Microbe 12, 246–258. doi: 10.1016/j.chom.2012.06.005
Jones, M. L., Kitson, E. L., and Rayner, J. C. (2006). Plasmodium falciparum erythrocyte invasion: a conserved myosin associated complex. Mol. Biochem. Parasitol. 147, 74–84. doi: 10.1016/j.molbiopara.2006.01.009
Kanaani, J., and Ginsburg, H. (1989). Metabolic interconnection between the human malarial parasite Plasmodium falciparum and its host erythrocyte. Regulation of ATP levels by means of an adenylate translocator and adenylate kinase. J. Biol. Chem. 264, 3194–3199.
Kasasa, S., Asoala, V., Gosoniu, L., Anti, F., Adjuik, M., Tindana, et al. (2013). Spatio-temporal malaria transmission patterns in Navrongo demographic surveillance site, northern Ghana. Malar J. 12:63. doi: 10.1186/1475-2875-12-63
Kelley, L. A., Mezulis, S., Yates, C. M., Wass, M. N., and Sternberg, M. J. (2015). The Phyre2 web portal for protein modeling, prediction and analysis. Nat. Protoc. 10, 845–858. doi: 10.1038/nprot.2015.053
Kippert, F., and Gerloff, D. L. (2009). Highly sensitive detection of individual HEAT and ARM repeats with HHpred and COACH. PLoS ONE 4:e7148. doi: 10.1371/journal.pone.0007148
Klinkenberg, E., McCall, P., Wilson, M. D., Amerasinghe, F. P., and Donnelly, M. J. (2008). Impact of urban agriculture on malaria vectors in Accra, Ghana. Malar J. 7:151. doi: 10.1186/1475-2875-7-151
Koike, M., Kose, S., Furuta, M., Taniguchi, N., Yokoya, F., Yoneda, Y., et al. (2004). Mechanism of signal transduction-β-Catenin shows an overlapping sequence requirement but distinct molecular interactions for its bidirectional passage through nuclear pores. J. Biol. Chem. 279, 34038–34047. doi: 10.1074/jbc.M405821200
Kono, M., Herrmann, S., Loughran, N. B., Cabrera, A., Engelberg, K., Lehmann, C., et al. (2012). Evolution and architecture of the inner membrane complex in asexual and sexual stages of the malaria parasite. Mol. Biol. Evol. 29, 2113–2132. doi: 10.1093/molbev/mss081
LaCount, D. J., Vignali, M., Chettier, R., Phansalkar, A., Bell, R., Hesselberth, J. R., et al. (2005). A protein interaction network of the malaria parasite Plasmodium falciparum. Nature 438, 103–107. doi: 10.1038/nature04104
Lee, S. J., Imamoto, N., Sakai, H., Nakagawa, A., Kose, S., Koike, M., et al. (2000). The adoption of a twisted structure of importin-β is essential for the protein-protein interaction required for nuclear transport. J. Mol. Biol. 302, 251–264. doi: 10.1006/jmbi.2000.4055
Ligon, L. A., Karki, S., Tokito, M., and Holzbaur, E. L. (2001). Dynein binds to β-catenin and may tether microtubules at adherens junctions. Nat. Cell Biol. 3, 913–917. doi: 10.1038/ncb1001-913
Lopaticki, S., Maier, A. G., Thompson, J., Wilson, D. W., Tham, W.-H., Triglia, T., et al. (2011). Reticulocyte and erythrocyte binding-like proteins function cooperatively in invasion of human erythrocytes by malaria parasites. Infect. Immun. 79, 1107–1117. doi: 10.1128/IAI.01021-10
McCallum, F. J., Persson, K. E., Fowkes, F. J., Persson, K. E., Fowkes, F. J., Reiling, L., et al. (2017). Differing rates of antibody acquisition to merozoite antigens in malaria: implications for immunity and surveillance. J. Leukoc. Biol. 101, 913–925. doi: 10.1189/jlb.5MA0716-294R
McCrea, P. D., Turck, C. W., and Gumbiner, B. (1991). A homolog of the armadillo protein in Drosophila (plakoglobin) associated with E-cadherin. Science 254, 1359–1361. doi: 10.1126/science.1962194
McRobert, L., Taylor, C. J., Deng, W., Fivelman, Q. L., Cummings, R. M., Polley, S. D., et al. (2008). Gametogenesis in malaria parasites is mediated by the cGMP-dependent protein kinase. PLoS Biol. 6:e139. doi: 10.1371/journal.pbio.0060139
Meng, M., He, S., Zhao, G., Bai, Y., Zhou, H., Cong, H., et al. (2012). Evaluation of protective immune responses induced by DNA vaccines encoding Toxoplasma gondii surface antigen 1 (SAG1) and 14-3-3 protein in BALB/c mice. Parasit Vectors. 5:273. doi: 10.1186/1756-3305-5-273
Mensah-Brown, H. E., Abugri, J., Asante, K. P., Dwomoh, D., Dosoo, D., Atuguba, F., et al. (2017). Assessing the impact of differences in malaria transmission intensity on clinical and haematological indices in children with malaria. Malar. J. 16:96. doi: 10.1186/s12936-017-1745-8
Mensah-Brown, H. E., Amoako, N., Abugri, J., Stewart, L. B., Agongo, G., Dickson, E. K., et al. (2015). Analysis of erythrocyte invasion mechanisms of Plasmodium falciparum clinical isolates across 3 malaria-endemic areas in Ghana. J. Infect. Dis. 212, 1288–1297. doi: 10.1093/infdis/jiv207
Mensah-Brown, H. E., Aspeling-Jones, H., Delimini, R. K., Asante, K. P., Amlabu, E., Bah, S., et al. (2019). Antibody reactivity to merozoite antigens in ghanaian adults correlates with growth inhibitory activity against Plasmodium falciparum in culture. Open Forum Infec. Dis. 6:ofz254. doi: 10.1093/ofid/ofz254
Meszoely, C. A., Erbe, E. F., Steere, R. L., Trosper, J., and Beaudoin, R. L. (1987). Plasmodium falciparum: freeze-fracture of the gametocyte pellicular complex. Exp. Parasitol. 64, 300–309. doi: 10.1016/0014-4894(87)90040-3
Mitra, P., Gupta, E. D., Sahar, T., Pandey, A. K., Dangi, P., Reddy, K. S., et al. (2016). Evidence for the nucleo-apical shuttling of a beta-catenin like Plasmodium falciparum armadillo repeat containing protein. PLoS ONE 11:e0148446. doi: 10.1371/journal.pone.0148446
Mueller, C., Klages, N., Jacot, D., Santos, J. M., Cabrera, A., Gilberger, T. W., et al. (2013). The Toxoplasma protein ARO mediates the apical positioning of rhoptry organelles, a prerequisite for host cell invasion. Cell Host Microbe 13, 289–301. doi: 10.1016/j.chom.2013.02.001
Mueller, C., Samoo, A., Hammoudi, P.-M., Klages, N., Kallio, J. P., Kursula, I., et al. (2016). Structural and functional dissection of Toxoplasma gondii armadillo repeats only protein. J. Cell Sci. 129, 1031–1045. doi: 10.1242/jcs.177386
Osier, F., Feng, G., and Boyle, M. J. (2014). Opsonic phagocytosis of Plasmodium falciparum merozoites: mechanism in human immunity and a correlate of protection against malaria. BMC Med. 12:108. doi: 10.1186/1741-7015-12-108
Otto, T. D., Wilinski, D., Assefa, S., Keane, T. M., Sarry, L. R., Böhme, U., et al. (2010). New insights into the blood-stage transcriptome of Plasmodium falciparum using RNA-Seq. Mol. Microbiol. 76, 12–24. doi: 10.1111/j.1365-2958.2009.07026.x
Owusu-Agyei, S., Asante, K. P., Adjuik, M., Adjei, G., Awini, E., Adams, M., et al. (2009). Epidemiology of malaria in the forest-savanna transitional zone of Ghana. Malar J. 8:220. doi: 10.1186/1475-2875-8-220
Raj, D. K., Nixon, C. P., Nixon, C. E., Dvorin, J. D., DiPetrillo, C. G., Pond-Tor, S., et al. (2014). Antibodies to PfSEA-1 block parasite egress from RBCs and protect against malaria infection. Science 344, 871–877. doi: 10.1126/science.1254417
Ramirez, I. B.-R., and Lowe, M. (2009). Golgins and GRASPs: holding the Golgi together. Semin. Cell Dev. Biol. 20, 770–779. doi: 10.1016/j.semcdb.2009.03.011
Riley, E., Allen, S., Wheeler, J., Blackman, M., Bennett, S., Takacs, B., et al. (1992). Naturally acquired cellular and humoral immune responses to the major merozoite surface antigen (Pf MSP1) of Plasmodium falciparum are associated with reduced malaria morbidity. Parasite Immunol. 14, 321–337. doi: 10.1111/j.1365-3024.1992.tb00471.x
Roy, A., Kucukural, A., and Zhang, Y. (2010). I-TASSER: a unified platform for automated protein structure and function prediction. Nat. Protoc. 5, 725–738. doi: 10.1038/nprot.2010.5
Schechtman, D., Winnen, R., Tarrab-Hazdai, R., Ram, D., Shinder, V., Grevelding, C. G., et al. (2001). Expression and immunolocalization of the 14-3-3 protein of Schistosoma mansoni. Parasitology 123, 573–582. doi: 10.1017/S0031182001008769
Schneider, M. P., Liu, B., Glock, P., Suttie, A., McHugh, E., Andrew, D., et al. (2017). Disrupting assembly of the inner membrane complex blocks Plasmodium falciparum sexual stage development. PLoS Pathog. 13:e1006659. doi: 10.1371/journal.ppat.1006659
Schwach, F., Bushell, E., Gomes, A. R., Anar, B., Girling, G., Herd, C., et al. (2015). PlasmoGEM, a database supporting a community resource for large-scale experimental genetics in malaria parasites. Nucleic Acids Res. 43, D1176–D1182. doi: 10.1093/nar/gku1143
Soldati, D., Foth, B. J., and Cowman, A. F. (2004). Molecular and functional aspects of parasite invasion. Trends Parasitol. 20, 567–574. doi: 10.1016/j.pt.2004.09.009
Stanisic, D. I., Fowkes, F. J., Koinari, M., Javati, S., Lin, E., Kiniboro, B., et al. (2015). Acquisition of antibodies against Plasmodium falciparum merozoites and malaria immunity in young children and the influence of age, force of infection, and magnitude of response. Infect. Immun. 83, 646–660. doi: 10.1128/IAI.02398-14
Straschil, U., Talman, A. M., Ferguson, D. J., Bunting, K. A., Xu, Z., Bailes, E., et al. (2010). The Armadillo repeat protein PF16 is essential for flagellar structure and function in Plasmodium male gametes. PLoS ONE 5:e12901. doi: 10.1371/journal.pone.0012901
Tao, D., Ubaida-Mohien, C., Mathias, D. K., King, J. G., Pastrana-Mena, R., Tripathi, A., et al. (2014). Sex-partitioning of the Plasmodium falciparum stage V gametocyte proteome provides insight into falciparum-specific cell biology. Mol. Cell. Proteomics 13, 2705–2724. doi: 10.1074/mcp.M114.040956
Tewari, R., Bailes, E., Bunting, K. A., and Coates, J. C. (2010). Armadillo-repeat protein functions: questions for little creatures. Trends Cell Biol. 20, 470–481. doi: 10.1016/j.tcb.2010.05.003
Treeck, M., Struck, N. S., Haase, S., Langer, C., Herrmann, S., Healer, J., et al. (2006). A conserved region in the EBL proteins is implicated in microneme targeting of the malaria parasite Plasmodium falciparum. J. Biol. Chem. 281, 31995–32003. doi: 10.1074/jbc.M606717200
Tremp, A. Z., and Dessens, J. T. (2011). Malaria IMC1 membrane skeleton proteins operate autonomously and participate in motility independently of cell shape. J. Biol. Chem. 286, 5383–5391. doi: 10.1074/jbc.M110.187195
van den Hoogen, L. L, Walk, J., Oulton, T., Reuling, I. J., Reiling, L., Beeson, J. G., et al. (2019). Antibody responses to antigenic targets of recent exposure are associated with low-density parasitemia in controlled human Plasmodium falciparum infections. Front. Microbiol. 10:3300. doi: 10.3389/fmicb.2018.03300
Wetzel, J., Herrmann, S., Swapna, L. S., Prusty, D., Peter, A. T. J., Kono, M., et al. (2015). The role of palmitoylation for protein recruitment to the inner membrane complex of the malaria parasite. J. Biol. Chem. 290, 1712–1728. doi: 10.1074/jbc.M114.598094
Wilcke, L. (2018). Identification and characterization of invasion-related proteins of the malaria parasite Plasmodium falciparum (Welch, 1892) (Ph.D. thesis dissertation), University of Hamburg, Hamburg, Germany.
Yeoman, J. A., Hanssen, E., Maier, A. G., Klonis, N., Maco, B., Baum, J., et al. (2011). Tracking Glideosome-associated protein 50 reveals the development and organization of the inner membrane complex of Plasmodium falciparum. Eukaryot. Cell. 10, 556–564. doi: 10.1128/EC.00244-10
Keywords: PfATRP, recombinant protein, immunolocalization, immunoreactivity, serosurveilance
Citation: Amlabu E, Ilani P, Opoku G, Nyarko PB, Quansah E, Thiam LG, Anim M, Ayivor-Djanie R, Akuh O, Mensah-Brown H, Rayner JC and Awandare GA (2020) Molecular Characterization and Immuno-Reactivity Patterns of a Novel Plasmodium falciparum Armadillo-Type Repeat Protein, PfATRP. Front. Cell. Infect. Microbiol. 10:114. doi: 10.3389/fcimb.2020.00114
Received: 25 October 2019; Accepted: 02 March 2020;
Published: 20 March 2020.
Edited by:
Ricardo Ataide, Burnet Institute, AustraliaReviewed by:
Takafumi Tsuboi, Ehime University, JapanKevin Marsh, Oxford University, United Kingdom
Danny Wilson, University of Adelaide, Australia
Copyright © 2020 Amlabu, Ilani, Opoku, Nyarko, Quansah, Thiam, Anim, Ayivor-Djanie, Akuh, Mensah-Brown, Rayner and Awandare. This is an open-access article distributed under the terms of the Creative Commons Attribution License (CC BY). The use, distribution or reproduction in other forums is permitted, provided the original author(s) and the copyright owner(s) are credited and that the original publication in this journal is cited, in accordance with accepted academic practice. No use, distribution or reproduction is permitted which does not comply with these terms.
*Correspondence: Emmanuel Amlabu, eamlabu@ug.edu.gh; amlabu.e@ksu.edu.ng; Gordon A. Awandare, gawandare@ug.edu.gh
†These authors have contributed equally to this work