Abstract
This paper establishes the feasibility of a reusable biosensor that can be operated and stored at room temperature, for detection of small molecules in low resource settings. The sensor was fabricated using molecularly imprinted polymers (MIP) and cortisol was chosen as a model analyte. Cortisol imprinted polymer films were prepared by electropolymerizing pyrrole on an electrode surface in the presence of cortisol. Electrochemical over-oxidation of polypyrrole (PPy) was performed for the controlled release of cortisol templates and to create cortisol specific imprinting sites. Stepwise fabrication of imprinted sensors was characterized through cyclic voltammetry (CV) and scanning electron microscopy (SEM). The sensor exhibited a detection limit of 1 pM L−1 for cortisol. A unique feature of the sensor was that cross-reactivity with prednisolone (which has 100% interference in ELISA), was minimized to 18.3% compared to ELISA. The sensitivity of the sensor remained over 90% after 7 cycles of elution/rebinding, while the sensitivity decreased by 10% after 4 weeks of storage at room temperature, suggesting the sensor can be used multiple times and used with low overhead costs in low resource settings such as agricultural fields. The sensor was used for detection of cortisol in saliva samples of farm workers; benchmarking with ELISA showed excellent correlation. These findings indicate that such a sensor can be used for in-field measurements of small molecules (e.g. cortisol).
Export citation and abstract BibTeX RIS
Psychological stress is associated with increased risk of several health conditions, including cardio-vascular disease, autoimmune disorders, infectious disease, and mental illnesses.1 Many people experience medically relevant psychological stress in response to actual or perceived stressors, that can negatively impact health.2,3 Farmworkers, working in extreme environments that are exposed to fertilizers and chemicals are a population group generally considered at high risk.4 Numerous studies are underway to quantify the effect of environmental and psychological stressors on the health of farm workers5,6 One mechanism of linking psychological stress to disease is the biological response that is associated with stress, namely the production of cortisol. In its free form, cortisol plays an important role in the regulation of various physiological processes such as blood pressure, glucose levels, and carbohydrate metabolism.7,8 However, chronically elevated cortisol due to environmental or psychological distress is associated with neuroendocrine causal pathway linking stress to poor health outcomes.9 The quantification of cortisol, a small molecule, is thereby necessary for the assessment and management of physiological stress. The measurement of cortisol levels (and measurement of other similar metabolites) in the body is also an important diagnostic tool, both in clinical settings and during certain stress-intensive activities. Thus, the development of technologies that are easy to use and enable the quick detection of such molecules of interest (e.g. cortisol) have been the focus of research in recent years.
Currently, analytical methods such as enzyme-linked immunosorbent assay (ELISA) and electrochemical immunosensors are widely used to detect cortisol.10,11 A chemiluminescent-lateral flow immunoassay (LFIA) method integrated to a smartphone has been recently developed for cortisol assessment.12 These analytical techniques involve using cortisol specific antibodies as bio-recognition elements and providing selective detection of cortisol. However, there are many challenges to the commercialization of immunosensors and immunoassays for point-of-care (POC) applications. These include (1) the instability of antibodies in ambient environment, leading to (2) the need for refrigerated transport and storage, and (3) the high cost of producing immune-reagents. The standard research practice of collecting all samples before running the assays in a single session (to minimize variations) results in an inability to use the results of tests on patients/subjects to improve their health outcomes.
Thus our research has focused on the design and synthesis of artificial receptor systems, as stable mimics to the conventional antibodies with high selectivity. Such technologies would reduce the cost of assay, storage, handling, and transportation. One technique that is being increasingly adopted for the development of artificial bioreceptors is molecular imprinting.13,14 Molecular imprinting is used to create specific recognition sites in polymeric matrices that are structurally complementary to target molecules. Molecularly imprinted polymers (MIP) are synthetic polymers that have recognition sites that are selective toward the target analyte. They are considered to be versatile, stable, and cost-effective substitutes for the natural antibodies.15,16 MIP possess longer lifetimes than enzymes, antibodies, and other types of proteins. They also allow reversible binding with the target analyte, which makes the MIP sensors suitable for constructing reusable sensing technologies. MIP potentially can have a huge impact on applications in biosensor commercialization, due to their low cost, easy storage, and applicability in harsh conditions.17,18
Traditional polymerization techniques such as bulk polymerization, precipitation polymerization, and emulsion have been widely used for the synthesis of MIP. However, the controlled integration of MIP onto the transducer's surface remains a challenge. Electrochemical polymerization is an attractive method for patterning MIP directly onto the surface of biochips as it allows one to control the thickness, morphology, and reproducibility of MIP films by tuning experimental parameters such as the ratio/concentration of target/monomer, polymerization cycles, and applied voltage.19,20 To synthesize MIP by electropolymerization various electro-active monomers, such as pyrrole, aniline and o-phenylenediamine, have been explored as possible materials. Among them, polypyrrole (PPy)-based MIP was preferred because of its facile electropolymerization and stability in ambient conditions.21,22
Although examples of protocols for the synthesis of MIP for cortisol have been reported by several research groups,23–25 this paper is the first reported effort to obtain an in situ fabricated MIP for the electrochemical detection of cortisol. One of the key aspects in designing reproducible MIP biosensors for electrochemical detection is designing a controlled system to remove the template molecule from the polymer matrix. In order to remove the entrapped template molecule from the polymeric matrix, different strategies were explored. The most straightforward approach was extraction with an appropriate solvent. Other extraction attempts involving temperature, microwaves, or ultrasonics (to increase the rate of extraction) resulted in distorted imprinted cavities and, consequently, made the MIP less efficient in rebinding and selectivity.26
One of the major limitations of currently available commercial cortisol immunoassay kits and immunosensors is their cross-reactivity and interference with structural analogs of cortisol, namely, progesterone and prednisolone.27,28 As recently reviewed by Krasowski et al., commercially available cortisol immunoassay kits still have cross-reactivity of more than 100% with the analogs, especially prednisolone.29 This means that there is a real need to develop an assay for cortisol detection that has lower cross-reactivity with the analogs. In this study, an electrochemical elution approach was performed using cyclic voltammetry to extract the cortisol from the polymeric matrix. The effects of electrochemical extraction cycles on the template removal were also investigated. The fabricated biosensor first characterized using laboratory controls and was then used with field samples to detect human salivary cortisol levels of farm workers. The results of the sensor were correlated and validated with ELISA measurements.
Materials and Methods
Reagents
Pyrrole monomer (≥98%), potassium ferricyanide (K3[Fe(CN)6]), potassium ferrocyanide (K4[Fe(CN)6]), and potassium chloride (KCl) were purchased from Sigma-Aldrich Co., USA. Phosphate-buffered saline (PBS) solution (10 mM, pH 7.4) was prepared by dissolving a PBS tablet (2005.5 mg) in 200 mL of deionized (DI) water. Stock solution of cortisol was prepared by dissolving 1 mg of cortisol in 1 mL of ethanol. Cortisol working aliquots were prepared by diluting the stock solution with PBS. Standard stock solution of K3[Fe(CN)6])/ K4[Fe(CN)6] (5 mM) was prepared using PBS. A cortisol enzyme immunoassay kit was procured from Arbor Assays, MI and the recommended protocol was adapted to detect salivary cortisol. For the detection of cortisol, 50 μl of 4x diluted saliva was used.
Instrumentation
All electrochemical experiments were performed with the Autolab potentiostat/galvanostat (Eco Chemie, Netherlands). A planar screen-printed carbon electrode (SPCE) consisting of a carbon working electrode, a carbon counter electrode, and an Ag/AgCl reference electrode (CH Instruments, Inc., USA) was used to design the cortisol MIP sensor. Scanning electron microscopic images (SEM) of the imprinted films were taken using a JEOL (JSM-7000) electron microscope.
Formation of MIP and non-imprinted polymer (NIP) modified electrodes
The SPCE was pre-treated by cycling the potential between −1.5 V to 1.5 V at a scan rate of 100 mV/s in 0.1 M H2SO4 for 10 cycles. The SPCE was then washed with deionized water and dried at room temperature. The electro-synthesis of MIP films was performed using cyclic voltammetry by cycling the potential range from 0 V to 0.9 V at a scan rate of 50 mV/s for 10 cycles in PBS (10 mM, pH 7.4) containing 0.8 M pyrrole and 0.1 M KCl.30 Cortisol (10 mM) was added to the solution before the polymerization. As the polymer grows, cortisol molecules migrate toward the working electrode and get entrapped in the polymer matrix.
After polymerization, the entrapped cortisol molecules were extracted from the conducting polymer matrix to produce a surfaces that are complimentary in shape and functionality to cortisol. Cortisol templates were extracted through the over-oxidation of the PPy by cycling the potential range between −0.2 and 0.8 V for 25 cycles in PBS. The same procedure (polymerization followed by over-oxidation) was used to fabricate NIP electrodes but without the cortisol template. MIP and NIP modified electrodes were then dried under nitrogen flow and stored at room temperature. The stepwise fabrication of the cortisol imprinted sensor is illustrated in Scheme 1.
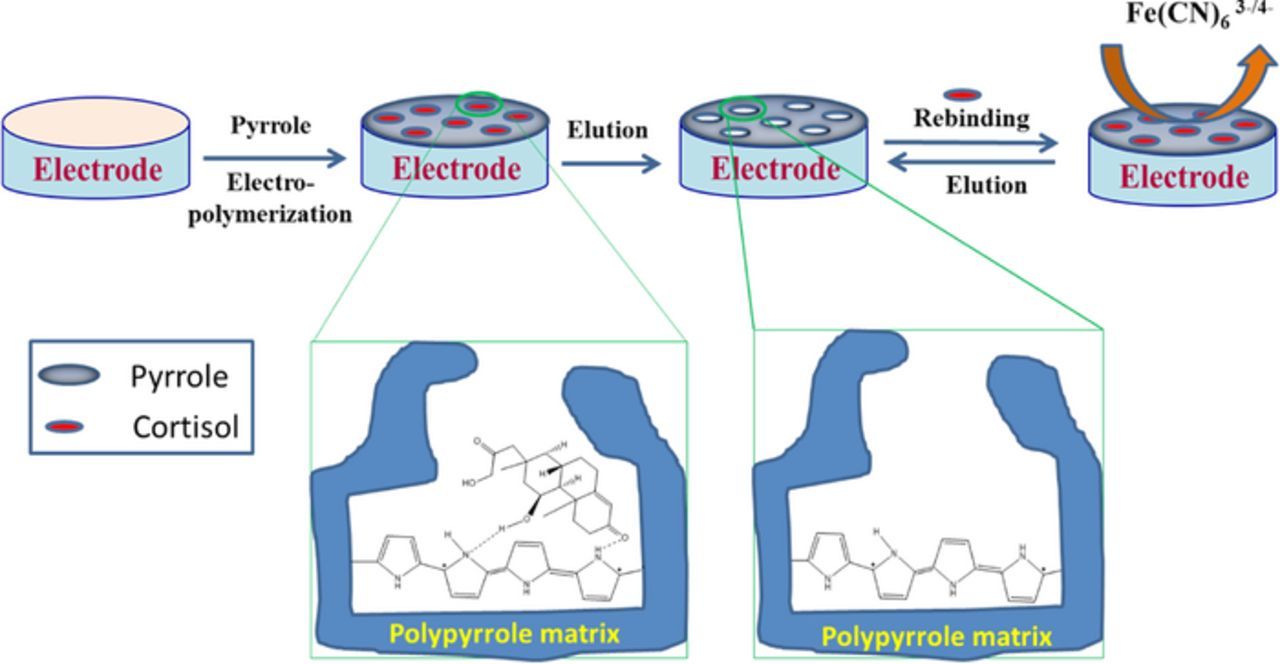
Scheme 1. Schematic representation of the stepwise fabrication of cortisol MIP biosensors.
Cortisol detection using MIP sensor
The electrochemical detection of cortisol by the MIP sensor was performed by placing 5 μL of appropriate concentration of cortisol solution at the working electrode for 15 min. The electrochemical measurements were carried out in the presence of 40 μL of 5 mM K3[Fe(CN)6]/K4[Fe(CN)6] solution containing PBS (10 mM, pH 7.4) at room temperature.
Results and Discussion
Fabrication of cortisol MIP and NIP electrodes
The formation of cortisol specific MIP films on the electrode surface was achieved by electrochemical polymerization of pyrrole in the presence of cortisol. The polymer growth was monitored via changes in the voltammograms obtained during the electropolymerization process. Figure 1A shows the voltammograms obtained during electropolymerization of pyrrole in the absence (dotted lines) and in the presence of cortisol (solid lines), from 0 V to 0.9 V at 50 mV/s using 0.1 M KCl as a supporting electrolyte. In both cases, the oxidation currents were observed to increase at each scan cycle, implying a controlled growth of the polymer. This suggests that the thickness of the polymer film can be controlled by regulating the scan cycles. During polymerization, cortisol molecules diffuse toward the electrode and bind to the PPy matrix through hydrogen bonding (Scheme 1). Although the two voltammograms look similar in shape as showed in Fig. 1A, the capacitive currents observed for the electropolymerization in the presence of cortisol (solid lines) is significantly higher than those obtained in its absence (dotted lines). The increased capacitive current of polymer film in the presence of cortisol confirms the binding of cortisol, an electro-inactive molecule, with the polymer.
Figure 1. (A) Cyclic voltammograms taken during the electro-polymerization of pyrrole onto the SPCE both with (solid lines) and without (dotted lines) the presence of 10 mM cortisol. Scan rate: 50 mV/s. (B) Recorded voltammograms during the elution of cortisol from the PPy matrix in PBS (10 mM, pH 7.4). Scan rate of 50 mV/s.
Removal of cortisol templates from the polymeric matrix was necessary to form complementary imprinted sites for the subsequent rebinding. Electrochemical over-oxidation process was used to remove cortisol molecules from the PPy matrix. The PPy was over-oxidized by scanning the potential in a range of 0 V to +0.8 V for 25 cycles in PBS at a scan rate of 50 mV/s (Fig. 1B). During the elution scans, a broad irreversible voltammetric peak was observed at ∼0.3 V; this is due to the over-oxidation of PPy.31 As can be seen in Fig. 1B, no cathodic peak can be observed on the reverse scan, which indicates that the over-oxidation of PPy is electrochemically irreversible. The electrochemical over-oxidation causes the removal of cortisol from the PPy matrix while oxygen-containing groups, such as carboxyls, are inserted into the backbone of the polymer. Subsequent scan cycles performed in the study exhibited a decreasing current peak as indicated by an arrow. This is due to a decrease in conductivity of the PPy film as a result of over-oxidation. For clarity, the initial five scans of the total 25 are shown in Fig. 1B. Since over-oxidation was performed here under neutral pH conditions, the degradation of the PPy matrix was avoided. The NIP fabrication process follows the same protocol used to fabricate MIP (polymerization followed by over-oxidation), but without cortisol as a template.
Electrochemical characterization
The electrochemical behavior of the stepwise fabrication of the cortisol MIP sensor was studied in 5 mM K4[Fe(CN)6]/K3[Fe(CN)6] solution containing PBS. The results are shown in Fig. 2. The CVs of [Fe(CN)6]3−/[Fe(CN)6]4− redox probe was chosen as a marker to investigate the changes in the electrode behavior after each step of sensor assembly. As shown in Fig. 2a, a couple of well-defined reversible redox peaks for the [Fe(CN)6]3−/[Fe(CN)6]4− redox couple were observed with bare SPCE. The PPy modified SPCE showed an obvious increase in the peak current for the redox probe along with an increase in capacitive currents (Fig. 2b). This was attributed to the increase in effective surface area by the conducting PPy matrix on the SPCE surface. The permeability of the porous PPy film is also responsible for the increased electron transport kinetics. [Fe(CN)6]3−/[Fe(CN)6]4− is an anion; due to electrostatic interactions, the [Fe(CN)6]3−/[Fe(CN)6]4− ions insert easily into the positively charged PPy film and are thus involved in rapid electron transfer kinetics. The peak current response observed for the cortisol bound PPy matrix (Fig. 2c) was much lower than the one observed for the PPy matrix without cortisol (Fig. 2b). This further confirms that cortisol was effectively loaded onto the PPy matrix and hinders the electrochemical response of [Fe(CN)6]3−/[Fe(CN)6].4− It should be noted that the removal of cortisol from the PPy matrix drastically decreases the current response (Fig. 2d). The voltage-induced over-oxidation makes the PPy insulated by introducing carbonyl and carboxyl groups in the polymer backbone. The inset of Fig. 2 depicts the enlarged version of curve d, showing the redox currents observed with the over-oxidized PPy matrix.
Figure 2. Cyclic voltammograms of 5 mM K4[Fe(CN)6]/K3[Fe(CN)6] solution at (a) bare SPCE, (b) PPy-SPCE, (c) Cortsiol-PPy-SPCE and (d) MIP-PPy-SPCE. Scan rate of 50 mVs−1. (Inset: Enlarged version of curve d).
Surface morphological characterization
Surface morphologies of MIP and NIP modified electrodes were obtained at 30,000X magnification and are shown in Fig. 3. Fig. 3a depicts the SEM image of PPy deposited SPCE. After electro-polymerization, the surface of the SPCE is coated with micro-porous PPy matrix, with a cauliflower-like structure constituted by micro-spherical grains. The average diameter of the grain is 343.47 nm. When pyrrole was polymerized in the presence of cortisol, an increase in grain size was observed (Fig. 3b). The average diameter of the grains was increased to 616.32 nm, suggesting the incorporation of cortisol molecules into the polymer matrix. Over-oxidation of PPy matrix resulted in decreased grain size with increased porosity (Fig. 3c) due to the removal of cortisol from the matrix. The average diameter of the grain size was 342.83 nm. For comparison, the SEM image of NIP modified electrode is given in Fig. 3d. As can be seen from the SEM images, the surface of the MIP modified electrode is rough due to imprinted cavities of cortisol, whereas the surface of the NIP modified electrode is smooth without the imprinting cavities.
Figure 3. SEM images of (a) PPy-SPCE, (b) Cortisol-PPy-SPCE, (c) MIP-PPy-SPCE, and (d) NIP-PPy-SPCE.
Optimization of experimental parameters
The thickness of the MIP film is an important factor that affects the film's recognition ability and reproducibility of the sensor. It can be controlled by tuning the concentration of monomers and the number of polymerization cycles.
Effects of pyrrole monomer concentration
To investigate the effect of monomer concentrations on the electrochemical response of the MIP sensor, the cortisol MIP electrodes were prepared in solutions with a constant concentration of cortisol (10 mM) and varying concentrations of pyrrole (from 0.2 M to 1.2 M) (Fig. 4A). The polymerization and elution procedure to form MIP electrodes was followed as previously described in fabrication of cortisol MIP and NIP electrodes section. The fabricated cortisol MIP electrodes were characterized using cyclic voltammetry in 5 mM K4[Fe(CN)6]/K3[Fe(CN)6] solution containing PBS. The current response of the [Fe(CN)6]3−/[Fe(CN)6]4− redox probe increased with the increase in monomer concentration and reached a maximum value at 0.8 M, after which the current response decreased with further increases in the concentration of pyrrole monomer. This behavior can be attributed to the formation of a very thick layer of MIP at higher concentrations of monomer, which in turn affects the electron transfer of the [Fe(CN)6]3−/[Fe(CN)6]4− redox couple. Thus, the optimized 0.8 M pyrrole monomer concentration was chosen for the electrochemical polymerization to obtain the highest sensitivity for the determination of cortisol.
Figure 4. (A) Effect of the monomer concentrations on the response of the sensor to cortisol; (B) Effect of the different cycles of the electropolymerization of MIP, and (C) Effect of electrochemical extraction cycles on the current response.
Effect of electropolymerization cycle repetition and rate
Since the thickness of the polymer matrix can also be tuned via controlling the number of electro-polymerization cycles, the number of scan cycles used for MIP synthesis was optimized. A series of MIP electrodes was fabricated by following the experimental procedures mentioned in Formation of MIP and non-imprinted polymer (NIP) modified electrodes section; however, a different number of polymerization cycles were used to find out the optimal thickness. The electrochemical elution of the cortisol template from the polymeric matrix was performed using cyclic voltammetry as detailed in Fabrication of cortisol MIP and NIP electrodes section. As shown in Fig. 4B, the current response of the redox probe [Fe(CN)6]3−/[Fe(CN)6]4− reached a maximum after the 10th cycle of polymerization, and then decreased as the number of polymerization cycles increased. MIP films that were formed with less than 10 scan cycles were found to be thin and less stable at the working electrode, whereas films formed with greater than 10 cycles formed thicker sensing layers that hinder the electron transfer of [Fe(CN)6]3−/[Fe(CN)6]4− redox probe. These results indicate that 10 is the optimum number of polymerization cycles
Optimization of extraction cycle
The efficient and controlled removal of the target molecules from the polymeric matrix is essential to fabricating reproducible MIP biosensors. An effective method is needed to regenerate the imprinting sites after the binding events to make the MIP sensors reusable. In this study, to create imprinting sites, the controlled removal of template molecules was performed by voltage-induced over-oxidation. The electrochemical elution of the cortisol template from the polymeric matrix was performed using cyclic voltammetry as detailed in Fabrication of cortisol MIP and NIP electrodes section. In order to optimize the extraction cycles, varying numbers of extraction cycles (5–40) were performed on the cortisol embedded PPy-SPCE. The MIP sensors were characterized after each cycle of target removal, using the redox probe [Fe(CN)6]3−/[Fe(CN)6].4− As the number of elution cycles increased, the current response of the MIP sensor also increased, indicating the controlled release of cortisol molecules. The maximum current response of the electrode was obtained at the 25th cycle (Fig. 4C). Thus, the extraction cycles were optimal at the 25th iteration for complete extraction of the template from the polymeric matrix.
Calibration curve of the sensor
The typical CV scans obtained for several concentrations of cortisol in PBS containing 5 mM [Fe(CN)6]3−/[Fe(CN)6]4− using the cortisol MIP sensor at 50 mV/s are shown in Fig. 5A. The figure depicts the magnitude of the electrochemical current response, which decreases as a function of increasing cortisol concentration when incubated for 15 min. Decreases in current response are attributed to the binding of cortisol molecules to the MIP sites that hinder electron transport of [Fe(CN)6]3−/[Fe(CN)6]4− redox probe. A calibration curve between the magnitudes of current response and the logarithm of cortisol concentration has been plotted (inset, Fig. 5A). The cathodic peak current observed at −0.35 V was used to plot calibration curves. The current responses obtained with the MIP sensor were proportional to the logarithmic value of cortisol concentrations in the range of 1 pM to 10 μM (R2 = 0.9925), with a detection limit of 1 pM.
Figure 5. (A) Electrochemical response of MIP-PPy-SPCE as a function of increasing cortisol concentration from 1 pM to10 uM (a-i) using 5 mM K4[Fe(CN)6]/K3[Fe(CN)6] solution, Inset: calibration curve between magnitudes of response current and logarithm of cortisol concentrations. (B) The change in peak current values observed at cortisol MIP (blue) and NIP (red) electrode in the presence of cortisol and other interferents individually each at 100 nM. (C) Molecular structure of cortisol and its structural analogs prednisolone, progesterone, and lactate.
Selectivity, reproducibility, and repeatability
Earlier, Ramstorm et al., evaluated the application of synthetic polymers capable of the molecular recognition of cortisol with reduced cross-reactivity.24 The MIP prepared by this method showed only 18.3% cross reactivity with the analogue prednisolone. In this work, the selectivity assay for cortisol MIP sensor was carried out using three interfering analytes: progesterone, prednisolone, and lactate. The change in peak current values observed at MIP and NIP electrodes in the presence of cortisol and other interferents, each at 100 nM, are shown in Fig. 5B. The imprinting factor (β), a measure of the strength of interaction between the target molecule and MIP sensor, was calculated according to the following equation, where Ipa is the cathodic peak current:

The values of β were 10.25, 1.27, 1.33 and 1.41 for cortisol, lactate, progesterone, and prednisolone, respectively. Based on the values obtained, it is clear that the MIP sensor shows higher binding capability toward cortisol (maximum β value) over other interferents. The above results revealed that the MIP sensor exhibited higher recognition selectivity toward cortisol. The cross-reactivity percentage was also calculated using the current values. It showed that lactate has very negligible interference (1.5%) while progesterone (11.4%) and prednisolone (18.3%) have slightly higher cross-reactivity with cortisol detection. The chemical structures of cortisol and other interferents are shown in Fig. 5C. Prednisolone and progesterone have chemical structures very similar to cortisol and thus interfere with cortisol measurement. But the percentage of cross-reactivity of the present MIP sensors is much lower than the conventional immunoassay. This cross-reactivity of the cortisol MIP sensors will be addressed in the future work by using computational modeling.32
To investigate the reproducibility, five sensors were freshly prepared and used to detect the same concentration of cortisol (100 nM). Based on the current responses obtained, the relative standard deviation (RSD) was calculated to be 4.16%, confirming that the sensor has good reproducibility (Table I). The RSD was determined by five successive measurements of a 100 nM cortisol solution using the same cortisol MIP electrode (i.e., used each time after elution process) and was estimated to be 4.81% (Table II). After the binding event, sensors were regenerated by electrochemical elution and were reused to detect cortisol. The experimental results demonstrated that the cortisol MIP sensor could be regenerated seven times; after that, the adhesion of the polymer to the electrode weakens. The stability of the sensor was examined by monitoring the current response for a 10 nM cortisol solution at regular intervals of 2 days for a period of four weeks. After four weeks, the sensor retained 90% of its current response. This suggests that the proposed sensor has acceptable storage stability.
Table I. Reproducibility experiments of the MIP-PPy-SPCE sensor.
Cortisol MIP sensors | 1 | 2 | 3 | 4 | 5 | RSD |
---|---|---|---|---|---|---|
Currenta (μA) | 30.11 | 29.61 | 28.24 | 30.39 | 28.59 | 4.16% |
Table II. Repeatability experiments of the cortisol MIP-PPy-SPCE sensor.
Cortisol solutions | 1 | 2 | 3 | 4 | 5 | RSD |
---|---|---|---|---|---|---|
Currenta (μA) | 28.15 | 29.10 | 29.85 | 30.98 | 31.80 | 4.81% |
Analytical applications
To further investigate the feasibility of the newly developed cortisol MIP sensor for usage in clinical analyses, salivary cortisol concentrations of farmworkers were tested using the MIP sensor and reference ELISA. The protocol to detect cortisol using the sensor and ELISA was adopted from our previous work.33 In short, 5 μL of each saliva sample were incubated on the biosensor for 15 min to ensure proper binding, followed by a wash using PBS (10 mM, pH 7.4) to remove any unbound saliva. Next, cyclic voltammetry scans were recorded in 5 mM K4[Fe(CN)6]/K3[Fe(CN)6] solution containing PBS. The results obtained using the MIP cortisol sensors are comparable to the results obtained by ELISA (Table III). It was observed that a factor of 2.4 was applied to all the CV calculations in comparison to ELISA. These results confirm the suitability of the electrode for human sample applications.
Table III. Comparison of saliva cortisol estimated using ELISA and cortisol MIP sensor.
Saliva | Salivary Cortisol | Salivary Cortisol |
---|---|---|
samples | (ELISA) (μg/dL) | (MIP sensor) (nM/L) |
Sample 1 | 0.237 | 6.75 |
Sample 2 | 0.155 | 4.32 |
Sample 3 | 0.144 | 4.13 |
Sample 4 | 0.072 | 2.16 |
Conclusions
In summary, a novel electrochemical sensor for the determination of cortisol was designed using a molecular imprinting technique. The cortisol MIP sensor was created by electro-polymerization of pyrrole monomers in the presence of a cortisol template, followed by the removal of cortisol under optimized conditions. The sensor response was linear (normalized from logarithmic curve) to the cortisol concentration in the range of 1 pM to 10 μM (R2 = 0.9925), with a detection limit of 1 pM. The sensor exhibited high sensitivity, short response time, and regeneration for seven uses. In addition, the sensor showed good repeatability, stability and selectivity, and was effective in the detection of cortisol in human saliva samples.
Acknowledgments
This work was supported by the National Science Foundation (NSF) ASSIST Nanosystems ERC (EEC-1160483). Authors acknowledge AMERI@FIU for research facilities.