Abstract
Graft copolymers were prepared by copolymerization of acrylonitrile with poly(sodium styrene sulfonate) macromonomers
Macromonomers were prepared by stable free radical polymerization. Polymers containing different graft chain densities were solvent-cast to provide a series of membranes of varying ionic content. Membranes were protonated and characterized with respect to water content, ionic conductivity, and as a medium for the electrochemical reduction of oxygen. Membrane properties were compared to other solid polymer electrolytes containing poly(styrenesulfonic acid) graft chains. Compared to analogous membranes that contain the more hydrophobic polystyrene backbone, polyacrylonitrile-based membranes uptake more water for a given ion exchange capacity. This has the effect of lowering proton conductivity, due to dilution of ions; increasing the diffusion coefficient of oxygen; and decreasing the oxygen solubility in the membrane. Due to the offsetting effects of the increased diffusion coefficient and decreased solubility, the oxygen permeability through polystyrene- and polyacryonitrile-based membranes is similar for a given ion exchange capacity. © 2003 The Electrochemical Society. All rights reserved.
Export citation and abstract BibTeX RIS
Proton exchange membranes are employed as separators for the reactant gases in solid polymer electrolyte fuel cells and provide the required ionic path between the anode and the cathode. The performance of a fuel cell is directly affected by membrane properties such as water content, proton conductivity, mechanical strength, and chemical stability. The synthesis and characterization of novel membranes is an active area of research because development of cheaper and more versatile solid polymer electrolytes is necessary for the field to progress toward commercialization. Among methods to reduce the cost of polymer electrolytes is the use of hydrocarbon and partially fluorinated polymer membranes.1 2 3 These can be obtained via synthetic routes using functionalized monomers that contain an ionic group or a precursor to an ionic group, or by introducing the ionic group onto a preformed polymer by postfunctionalization.4 Proton exchange membranes with promising characteristics have also been produced by radiation-grafting, in which monomers are polymerized in prefabricated polymer films and subsequently functionalized.5 6 7 8 9 10 11 12
In order to assess the applicability of proton exchange membranes (PEMs) in fuel cells, it is necessary to undertake fundamental studies on solid polymer electrolytes. Many studies investigate the mechanism of proton transport and its relationship to polymer microstructure.13 14 15 16 17 18 Nafion® is the most widely studied membrane in this regard. Although its morphology is still under investigation,14 19 20 21 22 it is generally agreed that its phase-separated nature is responsible for its ability to serve as an electrolytic medium. On this basis, it has been suggested that proton conductivity through membranes is facilitated by small pores, good ionic connectivity, and high proton concentration.14 Research on the conductivity of different proton exchange membranes has shown that proton conductivity is correlated to the membrane's water content, ratio of water molecules to ionic sites (λ), and the overall proton concentration. These factors are determined by the composition and chemical structure of the membrane.23
In addition to ohmic losses caused by membrane resistance, fuel cell performance is also dependent on the electrochemical kinetics and oxygen mass transport properties at the electrode|membrane interface. This is because the oxygen reduction reaction (ORR) is the rate-limiting reaction in fuel cells. As a result, ORR kinetics in several polymer electrolyte membranes has been actively studied using microelectrode techniques under conditions that mimic the environment of PEM fuel cells.24
25
26
Recently, the synthesis of graft polymers of styrene and sodium styrene sulfonate was reported.27 28 Films of these polymers phase separate into hydrophilic and hydrophobic domains. By varying the length and number density of the ionic graft chains on the polymer, the degree of phase separation can be modified. The extent of phase separation has been shown to affect the membrane's water content and proton conductivity. Furthermore, it should be possible to modify the extent of phase separation by the choice of the polymer backbone. A more hydrophobic backbone may be expected to enhance phase separation, whereas a more hydrophilic backbone may reduce it. In this paper, we report the synthesis and characterization of a series of graft polymers prepared with poly(sodium styrene sulfonate) (PSSNa) graft chains attached to a polyacrylonitrile (PAN) main chain. Polyacrylonitrile is more hydrophilic than polystyrene, but in the solid state it is more crystalline because of its relatively polar nature. Membranes prepared from these polymers are protonated and characterized in terms of their morphology, water content, conductivity, and electrochemical ORR mass-transport properties. These membranes are compared to other poly(styrenesulfonic acid) graft membranes prepared by different routes. The structure of the various graft polymers used in this study are shown in Scheme I. Comparisons of these membranes provides a means for evaluating the role of polymer structure and morphology on the electrochemical properties of solid polymers electrolytes.
Experimental
Membranes.—
Nafion 117 was manufactured by Du Pont de Nemours. Ethylenetetrafluoroethylene and
membranes were provided by Cranfield University (UK). They were prepared by radiation grafting of styrene onto preformed ETFE (Du Pont) and polyvinylideneflouride (PVDF) (Nowofol) films and subsequently sulfonated, as previously described.25
membranes were synthesized in this laboratory by a two-step method in which pseudo-living poly(sodium styrene sulfonate) macromonomer chains
were emulsion copolymerized with styrene.28 The procedure for the synthesis of
membranes is described in the following sections.
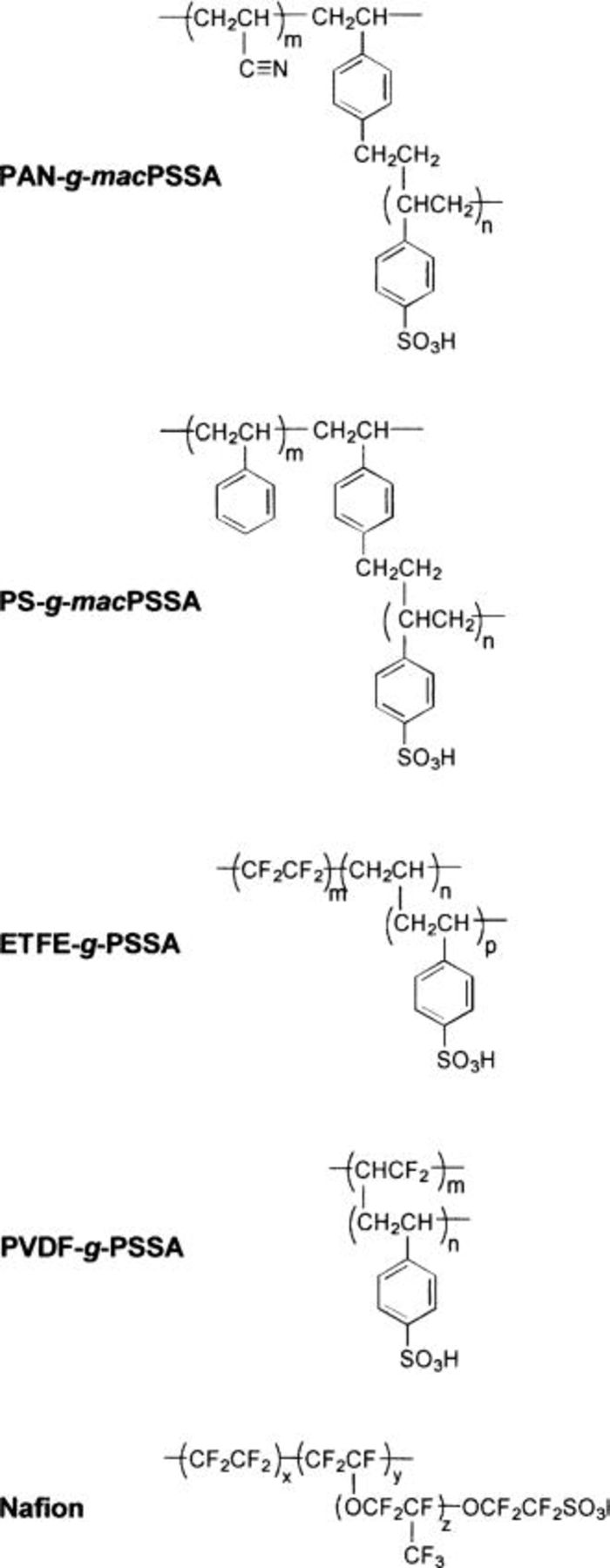
Scheme I. Polymer structures of membranes employed in this work.
Materials.—
Sodium styrene sulfonate (SSNa) and 2,2,6,6-tetramethyl-1-piperidinyloxy (TEMPO) were used as received (Aldrich). Divinylbenzene (Aldrich) was washed with NaOH (aq.) 10% and distilled over under reduced pressure. Ethylene glycol was distilled under reduced pressure. Acrylonitrile (Aldrich) was purified by distillation under reduced pressure.
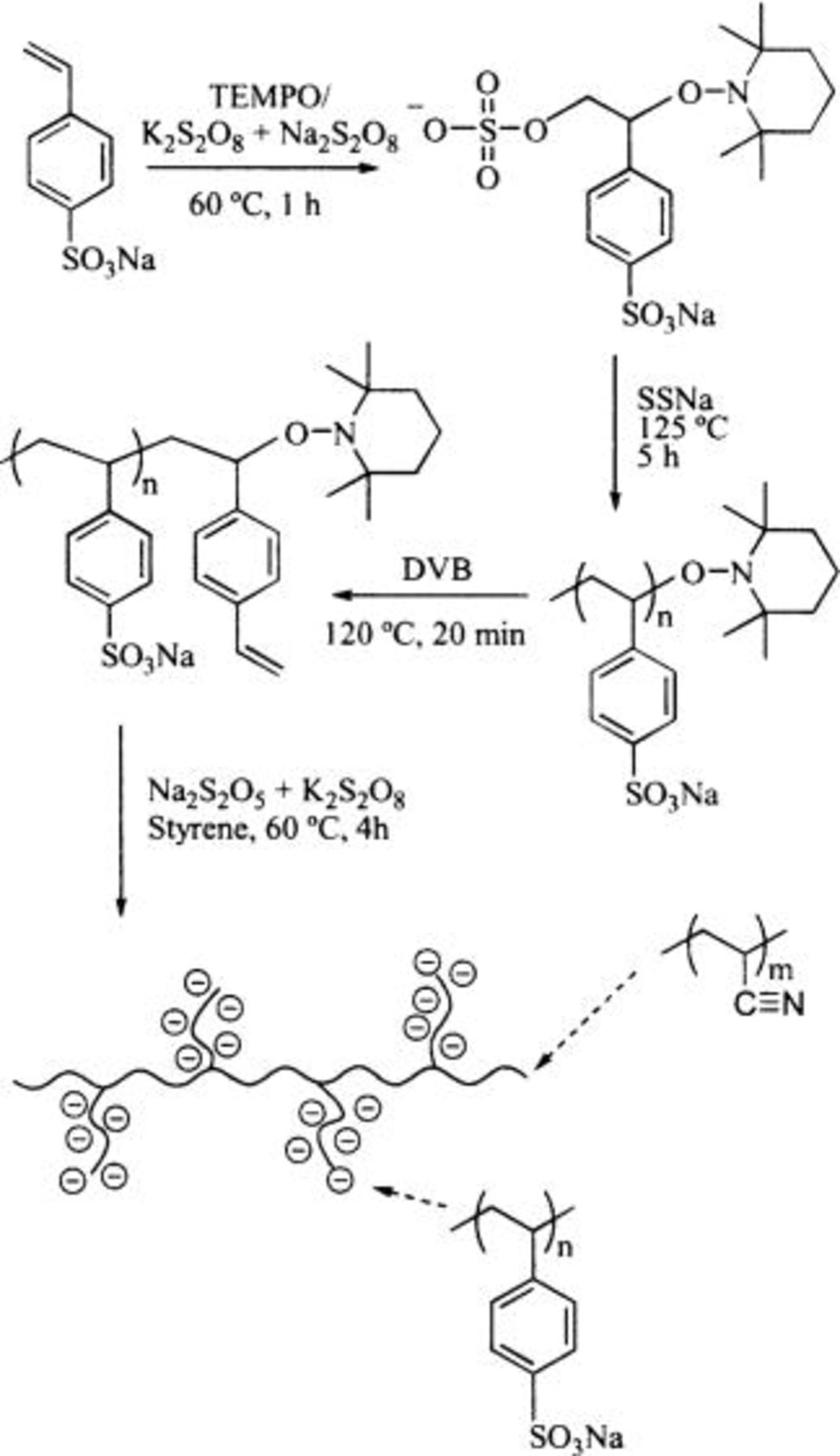
Scheme II. Synthesis of graft copolymers.
Synthesis of
.—
Three series of graft polymer membranes comprised of a polyacrylonitrile (PAN) backbone and graft chains of poly(sodium styrene sulfonate) (PSSNa) were prepared by a two-step method analogous to the synthesis of membranes previously reported.27
28
The first step is a pseudo-living free radical polymerization of sodium styrene sulfonate (SSNa), which is terminated with divinylbenzene (DVB) to form macromonomers of PSSNa The synthetic procedure and characterization of these macromonomers have been described in detail elsewhere and summarized as follows.28 An initiator system
is injected into a solution of SSNa in an ethylene glycol/water mixture with a stable free radical agent (2,2,6,6-tetramethyl-1-piperidinyloxy). The solution is stirred and heated to reflux (120-125°C). The molecular weight of the polymer increases with polymerization time. When the reaction is complete, DVB is coupled to the polymer to provide a vinylic terminus for subsequent grafting. The degree of polymerization of the macromonomer
is adjusted by varying the relative amounts of monomer to initiator used in the reaction. Three series of macromonomers with SSNa chain lengths of 16, 32, and 106 repeating units, and polydispersity indexes of
were synthesized.
The second step is the synthesis of the graft polymer by means of an emulsion polymerization of acrylonitrile and The polymerization was carried out in deionized
at 60°C. Polymers with different sulfonate content were obtained by varying the ratio of
to acrylonitrile in the feed. These ratios were 3/3, 3/4.5, 3/6, 3/9, 3/12, and 3/18
acrylonitrile). The volume of aqueous solvent used was 30, 35, 40, 50, 70, and 90 mL, respectively. In a typical polymerization, 3.0 g of
were dissolved in 27 mL of
and the system was purged with
3.0 mL of acrylonitrile and 50 mg of
in 3 mL of
were added to the system. The solution was heated to 60°C with stirring for 3.5 h. The polymer was precipitated into ether/methanol (60/40 vol %) mixture, filtered, and air dried. To remove low molecular weight oligomers and homopolymers of
the polymer was washed with cold water. After filtration the polymer was dried under vacuum. The copolymers are designated
(#), where # represents the degree of polymerization (DP) of the PSSNa graft chain. Acidified polymers are designated
(#), where PSSA represents polystyrene sulfonic acid.
Membrane fabrication.—
Membranes were prepared by solution casting from dimethylsulfoxide (DMSO) solutions containing 2% Polymer suspensions having a concentration
wt % were prepared at room temperature and heated to 150°C with stirring to form a clear solution. The solution was cast onto a glass plate and dried at 110°C for 10 h. A uniform, semitransparent film was released from the glass by immersion in water. Sodium ions were exchanged for protons by soaking the membranes in 0.5 M
for 2 days, followed by rinsing and storing in deionized water.
Water content and ion exchange capacity.—
To determine the ion exchange capacity (IEC) of membranes, samples in their acidic form were immersed in 50 mL of 2.0 M NaCl solution for 2 h and titrated with NaOH (0.025 M) to a phenolphthalein end point. After titration the membranes were placed for 1 h in 0.1 M HCl, rinsed with distilled water, and dried under vacuum at 80°C to constant weight. The membrane mass was measured using a Sartorius Basic BA21S balance. IEC was calculated using Eq. 1

The dimensions of the membranes were measured using a digital micrometer and caliper (Mitutoyo). Water contents were calculated as a volume percentage according to Eq. 2

Conductivity.—
Conductivity measurements were performed by ac impedance spectroscopy with a Hewlett-Packard 8753A network analyzer.29 Measurements were performed on the acidic form of the membranes, stored in Milli-Q water for 24 h prior to use. Impedance spectra between 300 kHz and 1 GHz were obtained under ambient conditions using a coaxial probe (2 mm inner diameter (id) central disk surrounded by a ring of id 6.5 mm) to which a gold film was evaporated onto the surface. The conductivity obtained for Nafion 117 (0.06-0.08 S/cm) is consistent with published results.
Transmission electron microscopy (TEM).—
Membranes were immersed in a saturated solution of for 24 h in order to enhance the contrast in electron density between ionic and nonionic domains. The samples were rinsed with
and dried under vacuum. Samples were embedded in Spur resin (Canemco) and sectioned along the normal direction to yield
thick slices using an ultramicrotome (Reichert OM U3). The slices were picked up with 100 mesh copper grids for TEM analysis. Micrographs were taken using a Zeiss microscope operated at 80 kV in the Electron Microscopy Laboratory, Bioscience Facilities of the University of British Columbia.
Solid-state electrochemistry.—
The solid-state electrochemical cell used to perform the experiments at 100% relative humidity, 3 atm pressure, and different temperatures has been described previously.30 Cyclic voltammetry, slow sweep voltammetry, and chronoamperometry were performed using a 50 μm radius Pt microdisk working electrode (Bioanalytical Systems), a Pt gauze counter electrode, and a custom-made dynamic hydrogen electrode (DHE). The reference electrode and the counter electrodes were brought into contact with a piece of Nafion 117 that acted as the backing membrane and worked as the electrolyte. The membrane of interest was held in place at the other side of the backing membrane by the spring-loaded working electrode. All experiments were conducted using a computer driven EG&G PAR model 283 potentiostat.
Prior to an experiment, the surface of the Pt microelectrode was pretreated by potential cycling (30 scans, 1.5 to 0.3 V). A detailed description of the surface pretreatment for the Pt microdisk electrode is given in Ref. 30, together with a description of the operation of the DHE. Potentials are stated relative to the DHE, unless otherwise indicated. Slow sweep voltammograms were performed at 5 mV/s, between 1.5 and 0.3 V. Chronoamperometry was conducted by holding the potential of the microelectrode at 1.2 V for 20 s, before stepping to a potential at which oxygen reduction is diffusion controlled (0.4 V) for 5 s. The current flowing at the microelectrode is given by the modified Cottrell equation

where is the geometric area of the electrode and all the other symbols have their usual meaning. The oxygen diffusion coefficient,
and oxygen solubility,
in a particular membrane may be obtained from the slope and intercept of an
vs.
plot provided that, in the time window studied, linear- and spherical-type diffusion both contribute to the overall current.31 The permeability of oxygen in each membrane was calculated from the product
Electrochemical parameters were determined only for
and
membranes with sufficiently high IEC to obtain reproducible data.
Results and Discussion
Water content, TEM, and conductivity.—
Three series of polymers with graft chain lengths of 16, 32, and 106 repeating units were synthesized. Polymers were solution-cast to produce flexible and semitransparent films of uniform thickness and good mechanical strength even when hydrated. Membranes were protonated as described in the Experimental section. A summary of the physicochemical properties of these membranes is given in Table I. IECs ranged from 0.45 to 1.85 meq/g, representing SSA mol % values of 2.6 to 13.7. Polymers with higher IECs than those listed were soluble in water, while polymers with lower IECs produced membranes with very low ionic conductivities. Water content ranged from 10 wt % for the lowest IEC membrane to 58% for the highest. Weight percent water contents were generally slightly lower than the volume percents. The averaged proton concentration ranged from 0.40 to 1.01 M in the hydrated membranes. These correspond to λ
values of 16 to 36. For comparison, the proton concentration and λ value of Nafion 117 was 1.05 M and 21, respectively.
Table I.
Properties of ![]() | ||||||
---|---|---|---|---|---|---|
IEC(meq/g) | SSAcontent(mol %) | Watercontent(wt %) | Watercontent(vol %) |
![]() |
![]() | σ(S/cm) |
![]() | ||||||
1.54 | 10.7 | 47 | 53 | 0.93 | 35 | 0.084 |
1.15 | 7.4 | 35 | 37 | 0.78 | 29 | 0.031 |
0.96 | 6.0 | 29 | 32 | 0.76 | 27 | 0.018 |
0.71 | 4.3 | 19 | 15 | 0.47 | 20 | 0.003 |
0.45 | 2.6 | 10 | 10 | 0.40 | 16 | - |
![]() | ||||||
1.76 | 12.8 | 50 | 56 | 0.97 | 36 | 0.100 |
1.39 | 8.5 | 42 | 48 | 0.87 | 32 | 0.055 |
1.17 | 7.6 | 36 | 42 | 0.88 | 29 | 0.037 |
0.98 | 6.1 | 28 | 33 | 0.90 | 25 | 0.013 |
0.81 | 4.9 | 22 | 23 | 0.66 | 20 | 0.006 |
![]() | ||||||
1.85 | 13.7 | 51 | 58 | 1.01 | 36 | 0.098 |
1.51 | 10.4 | 43 | 47 | 0.95 | 31 | 0.049 |
1.18 | 7.6 | 32 | 31 | 0.80 | 24 | 0.026 |
1.13 | 7.2 | 24 | 28 | 0.81 | 22 | 0.013 |
0.67 | 3.9 | 15 | 17 | 0.64 | 16 | 0.003 |
Figure 1 plots the water content of membranes as a function of ion exchange capacity. Water content increases with ionic content but is independent of graft chain length. Similarly, proton conductivity of (see Fig. 2a and b) while a strong function of both IEC and λ
is independent of graft chain length. This is in contrast to the case of
membranes,32 where it has been shown that water content and proton conductivity is strongly correlated to both IEC and graft chain length. An observation from Fig. 2a and b is the exponential growth of conductivity with IEC and λ, in contrast to the near-linear relationship between water content and IEC. These membranes can thus be classified as water-poor32 for which the uptake of additional water by the increase in IEC causes a significant enhancement of proton conductivity.
Figure 1. Plot of water content vs. ion exchange capacity for membranes.
Figure 2. Conductivity vs. (a) ion exchange capacity and (b) ratio for
membranes.
Figures 3
4
5 show TEM micrographs of membranes ultramicrotomed edge-on and possessing different ionic content (mol% SSA). The micrographs indicate the membranes have biphasic morphologies. Isolated ionic domains (dark regions) are evident for membranes possessing the lowest ionic content. However, the TEMs show that increasing the IEC by increasing the number density of graft chains, causes these domains to coalesce into a continuous ionic network. Phase separation is expected since the constituent polymer is comprised of ionic graft chains attached to a nonionic polyacrylonitrile backbone.
Figure 3. TEM micrographs of (16) membranes. SSA mol %: (a) 2.6, (b) 6.0, and (c) 10.7.
Figure 4. TEM micrographs of (32) membranes. SSA mol %: (a) 4.9, (b) 7.6, and (c) 12.8.
Figure 5. TEM micrographs of (106) membranes. SSA mol %: (a) 3.9, (b) 7.6, and (c) 13.7.
Comparison of TEM micrographs of and
membranes (see Ref. 32) shows that the extent of phase separation and connectivity of the ionic domains are similar even though the dielectric constant of polyacrylonitrile is much higher than polystyrene. TEM micrographs, by necessity, are obtained for dehydrated polymers. Upon hydration the hydrophilic domains are expected to swell and become considerably larger than the 10-25 nm size domain observed. In fact, the isolated ionic domains observed in the low ion content samples must coalesce upon swelling in order to support the proton conductivity values observed. The hydrophilic character of the polyacrylonitrile domains has a strong bearing on water uptake.
membranes therefore imbibe a much larger quantity of water than their polystyrene-based analogues. This is demonstrated in Fig. 6, which shows water content as a function of ionic content for
and
membranes comprised of polymers with similar PSSA graft chain length. Water sorption and proton conductivity values for other graft poly(styrenesulfonic acid) membranes are summarized in Table II and plotted in Fig. 6 and 7. The data show that within a series of membranes water content increases with IEC. The upper limit of IEC for
and Nafion-type membranes is determined by their dissolution in water. The ETFE- and PVDF-based membranes employed in this comparative study are prepared from preformed hydrophobic films. They can therefore be fabricated with much higher IECs, and they imbibe larger quantities of water without dissolution.
Figure 6. Water content vol % vs. ion exchange capacity for various membranes.
Table II.
Conductivity and water content for various PSSA grafted membranes. Nafion 117 included for comparison. | ||||||
---|---|---|---|---|---|---|
IEC(meq/g) | Watercontent(wt%) | Watercontent(vol%) |
![]() |
![]() | σ(S/cm) | Ref. |
![]() | ||||||
1.68 | 34 | 37 | 0.92 | 19 | 0.15 | 32 |
1.51 | 32 | 38 | 1.01 | 18 | 0.09 | |
1.29 | 21 | 14 | 1.00 | 12 | 0.04 | |
1.01 | 15 | 18 | 0.86 | 10 | 0.02 | |
0.81 | 11 | 10 | 0.71 | 8 | 0.01 | |
0.62 | 9 | 8 | 0.58 | 8 | - | |
![]() | ||||||
3.27 | 61 | 74 | 1.32 | 28 | 0.30 | 23, 25 |
2.56 | 49 | 66 | 1.42 | 23 | 0.22 | |
2.45 | 47 | 64 | 1.76 | 22 | 0.20 | |
2.13 | 42 | 53 | 1.52 | 22 | 0.19 | |
![]() | ||||||
3.06 | 61 | 82 | 1.55 | 28 | 0.32 | This work |
2.69 | 51 | 72 | 1.89 | 21 | 0.25 | |
2.24 | 44 | 68 | 1.87 | 19 | 0.20 | |
1.82 | 38 | 53 | 1.79 | 19 | 0.15 | |
1.37 | 29 | 50 | 1.52 | 17 | 0.10 | |
Nafion 117 (E)a | ||||||
0.91 | 26 | 39 | 1.05 | 21 | 0.10 | 23 |
a Expanded form. |
Figure 7. Conductivity vs. (a) ion exchange capacity and (b) ratio for various membranes.
Plots of conductivity vs. IEC and (λ) are shown in Fig. 7. They show that the conductivity within a series of membranes is dependent on the ionic content and water content. An explanation of the high conductivity of the
membranes has been previously reported, and is related to the high
in these membranes.25 The same explanation applies to
membranes. The plots illustrate that with these radiation-grafted membranes, conductivity increases with increasing λ, indicating that additional water assists proton conduction. Two anomalies deserve explanation. The first is that
membranes exhibit lower conductivities than ETFE and PVDF radiation-grafted membranes even though they possess similar values of λ. This is due to the lower IECs of
membranes which limit their water uptake and result in lower proton concentrations. Calculated values of
are presented in Tables I and II. Second, even though the
series of membranes possess similar IECs and
but much larger λ values than the
series, proton conduction through
membranes is smaller for a given ion content. This discrepancy is assumed to be due to water that swells the partially hydrophilic PAN domains in addition to sulfonic acid domains, and that this water does not assist proton conduction to the same degree. This is discussed in more detail in the next section in conjunction with the electrochemical characterization.
Oxygen mass-transport properties.—
Chronoamperometry was used to determine ORR mass transport properties in the membranes at the Pt/membranes interface. Oxygen diffusion coefficients and oxygen solubilities were obtained by linear regression analysis of vs.
plots. Table III summarizes the data. All data were obtained using the same instrumentation and under identical conditions (323 K, 3 atm
pressure, 100% RH).
Table III.
Oxygen mass transport parameters for various membranes at 323 K and 3 atm ![]() | |||||
---|---|---|---|---|---|
IEC(meq/g) | Watercontent(vol %) | Solubility
![]() ![]() | Diffusioncoefficient
![]() | Permeability
![]() ![]() | Ref. |
![]() | |||||
1.85 | 58 | 1.8 | 15.6 | 28 | This work |
1.76 | 56 | 2.3 | 17.8 | 41 | |
1.54 | 53 | 2.0 | 11.2 | 22 | |
1.51 | 47 | 2.1 | 7.3 | 15 | |
1.39 | 48 | 1.8 | 7.0 | 12 | |
1.15 | 37 | 1.9 | 3.7 | 7 | |
![]() | |||||
1.68 | 34 | 9.6 | 1.7 | 16 | This work |
1.55 | 33 | 9.2 | 2.6 | 24 | |
1.38 | 29 | 8.3 | 4.0 | 33 | |
1.31 | 22 | 7.9 | 1.7 | 13 | |
1.24 | 21 | 10.3 | 1.8 | 18 | |
1.04 | 9 | 14.1 | 0.8 | 11 | |
![]() | |||||
3.27 | 74 | 3.3 | 16.6 | 54 | 24 |
2.56 | 66 | 4.1 | 12.2 | 50 | |
2.45 | 64 | 4.4 | 9.3 | 40 | |
2.13 | 53 | 3.8 | 8.3 | 32 | |
![]() | |||||
3.06 | 82 | 3.4 | 17.9 | 61 | This work |
2.69 | 72 | 2.9 | 17.2 | 49 | |
2.24 | 68 | 2.9 | 14.4 | 43 | |
1.82 | 53 | 3.7 | 9.2 | 34 | |
1.37 | 50 | 3.2 | 4.7 | 15 | |
Nafion 117 (E)a | |||||
0.91 | 39 | 5.5 | 9.3 | 51 | 24 |
a Expanded form. |
Figure 8 plots the effect of IEC on oxygen mass-transport properties. diffusion coefficients increase with increasing IEC because water content increases with IEC and oxygen diffusion occurs largely through aqueous domains.31 In contrast, oxygen solubility remains relatively constant within a series. Oxygen solubility is favored in hydrophobic zones and surprisingly is not affected within a series to a great extent by variations in IEC and water content. As a consequence, the increase in oxygen permeability with increasing IEC follows the same trend as the diffusion coefficient.
Figure 8. Effect of ion exchange capacity on (a) diffusion coefficients, (b) solubilities, and (c) permeabilities of oxygen for various membranes. 323 K, 3 atm pressure, 100% RH.
Other pertinent observations include the high permeability of oxygen in Nafion compared to other membranes with similar IEC, and the relatively low diffusion coefficients but high oxygen solubility associated with the membranes. More insight into these results is obtained from comparisons of oxygen mass-transport properties plotted as a function of water content (Fig. 9). Figure 9a clearly demonstrates that
diffusion coefficients increase with increasing water content, and explains why
and
membranes allow higher
diffusion coefficients than Nafion 117. The
membranes support a higher diffusion coefficient for a given water content compared to
and
This is because the λ values are larger for
membranes and therefore, the water within the membrane is less coordinated to ionic groups. In the case of
membranes, the water contents and λ values are much lower than in
and consequently
diffusion coefficients are much lower.
Figure 9. Effect of water content on (a) diffusion coefficients, (b) solubilities, and (c) permeabilities of oxygen for various membranes. 323 K, 3 atm pressure, 100% RH.
In contrast to the diffusion coefficients, oxygen solubility in membranes is much higher than in
and
membranes because the former are relatively hydrophobic and their water contents lower. The
membranes solubilize less oxygen than the
and
membranes for a given water content because the polyacrylonitrile backbone is more hydrophilic than ETFE or PVDF. The high solubility of oxygen in Nafion is due to the nonpolar fluorocarbon matrix.31 The increase in oxygen permeability as a function of increasing water content is largely dominated by the increase in diffusion coefficient. The radiation-grafted membranes are highly permeable because of their large IEC and correspondingly high water content. Nafion 117 exhibits an unusually large permeability to oxygen given its modest water content. This is because the
diffusion coefficient is higher than expected based on the trends of other membranes (Fig. 9b) and because the oxygen solubility is significantly higher. This is likely a direct consequence of phase separation, which provides a continuous hydrophilic network for
diffusion and hydrophobic domains for oxygen solubility.
Since mass-transport limiting currents for the ORR are directly related to oxygen permeability, one might conclude that those materials possessing high oxygen permeability would be more suitable for fuel cell operation.24 However, for purposes of preventing crossover of reactant gases, low permeability may be required. Notwithstanding issues associated with membrane stability and processability, it would appear worthwhile to investigate mulitilayered PEMs or graded composition PEMs that are highly permeable to oxygen at, or near, the catalyst/membrane interface, and less permeable in the bulk of the membrane.
Conclusions
The ionic conductivity of proton exchange membranes is determined by several interrelated factors. For instance, water is necessary for proton transport, but a very high water content lowers the concentration of the sulfonic acid group and compromises conductivity. Obviously a continuous ionic pathway is required for appreciable conductivity. Phase separation, which is a function of polymer structure, enhances the connectivity of the ionic domains in low IEC membranes.
The water content of membranes has a marked effect on the oxygen mass-transport properties at membrane/electrode interfaces. Comparison of the electrochemical properties for various poly(styrenesulfonic acid) grafted membranes, prepared by living polymerization and by radiation grafting, show that oxygen diffusion coefficients increase with increasing water content within a series, while oxygen solubility decreases. However, significant differences exist in the conductivity and ORR mass-transport properties between different series of membranes. These differences can be readily explained on the basis of polymer structure, extent of phase separation, and the relative hydrophobicity/hydrophilicity of the membrane. Finally, it should be stated that the stability of hydrocarbon-based and partially fluorinated proton exchange membranes is compromised compared to perfluorinated materials such as Nafion. This may render them unsuitable for the majority of fuel cell applications. Nevertheless, the information presented provides insight into the role of structure and morphology on the electrochemical properties that may influence the design of more chemically robust membranes for fuel cells and other electrochemical applications.
Acknowledgments
This work was financially supported by the Natural Sciences and Engineering Research Council of Canada (NSERC), Ballard Power Systems, Inc., and British Gas Holdings (Canada), Ltd. We thank Dr. E. Humphrey for access to the Electron Microscopy Laboratory, Bioscience Facilities, University of British Columbia. We also thank Dr. Jackie Horsfall and Dr. K. V. Lovell (Cranfield University, UK) for preparing radiation-grafted membranes.