Abstract
The effect of charge transport on the photovoltaic properties of dye-sensitized solar cells (DSCs) was investigated by the experimental results and the ion transport. The short current photocurrent density (Jsc) is determined by the electron transport in porous TiO2 when the diffusion limited current (Jdif) due to the transport is larger than the photo-generated electron flux (Jg) estimated from the light harvesting efficiency of dye-sensitized porous TiO2 and the solar spectrum. However, the Jsc value is determined by the ion transport in the electrolyte solution at Jdif < Jg. The J value becomes constant against light intensity, and is expressed as the saturated current (
). The
value depends on the thickness (d) of the TiO2 layer, the initial concentration (
), and the diffusion coefficient (
) of
. These suitable parameters were determined by using the ion transport.
Export citation and abstract BibTeX RIS

Original content from this work may be used under the terms of the Creative Commons Attribution 3.0 licence. Any further distribution of this work must maintain attribution to the author(s) and the title of the work, journal citation and DOI.
1. Introduction
Dye-sensitized solar cells (DSCs) have received much attention because of their high energy-conversion efficiency (η = 12%) and low cost of production [1–3]. A DSC as shown in figure 1 is composed of a dye adsorbed porous TiO2 film on transparent conductive oxide (TCO) glass, an electrolyte solution containing an I−/ redox couple, and a counter electrode (CE). After light absorption of a dye, the electron is injected from the excited state of the dye to the conduction band of TiO2. The oxidized dye is reduced by iodide ions (I−) in the electrolyte solution. The ions such as I− and
transport through the porous TiO2 and bulk phase of the electrolyte solution. The oxidized I− (
) is reduced to I− on the surface of the CE. The injected electron also diffuses through the porous TiO2 and reaches to the TCO glass.
Figure 1. Schematic structure of dye-sensitized solar cells (DSCs).
Download figure:
Standard image High-resolution imageThe leakage of the electrolyte solution is the main problem for the application of DSCs because the electrolyte solution is liquid. Many approaches for long time stability have been carried out by introduction of polymers, TiO2 particle, and non-volatile solvents [3–12]. Ionic liquids have been also utilized as the electrolyte in DSCs for improvement of the durability because of the properties of high thermal stability, very low vapor pressure and non-flammability [5–9]. However, the photovoltaic performance of DSCs based on these stabilization techniques of the electrolytes is lower than that based on the normal volatile electrolyte. Especially, the photocurrent density of DSCs based on ionic liquid is lower than that based on volatile solution. The photocurrent is dominated not only by the charge injection from a dye to TiO2, but also by the charge transports of electrons, I− and . The ion transport rate in ionic liquid is generally slower than that of volatile solution. The lower photocurrent must be due to the slower ion transport. Therefore, the charge transports need to be discussed to explain the mechanism of Jsc and to find the suitable condition.
2. Experimental
1-propyl-3-methylimidazolium iodide (MPImI) was used as ionic liquid electrolytes. All electrolytes were prepared by dissolving 0.6 M 1,2-dimethyl-3-propylimidazolium iodide (DMPImI), 0.03–0.26 M I2, 0.05 M LiI, and 0.3 M 4-tert-butylpyridine (TBP). The viscosity of the electrolytes was measured by Viscotech Co., Ltd A carbazole dye with hexylsubstituted oligothiophene, MK-2 (Soken Chemical & Engineering Co., Ltd, Tokyo, Japan) [13] was used as a sensitizer. Transparent nanocrystalline TiO2 films were prepared as follows. TiO2 films were formed by screen printing method. The TiO2 paste (Nanoxide-20N, JGC catalysts and Chemicals Ltd) was printed on a transparent conducting oxide (TCO) glass substrate (F-doped SnO2, sheet resistance 10 Ω cm−2, Nippon Sheet Glass), followed by sintering at 500 °C for 60 min in air. After cooling to 100 °C, the films were immersed in a 0.3 mM of MK-2 solution for 24 h. The resulting electrodes were rinsed with acetonitrile. After drying at room temperature, the porous electrode was covered by a Pt sputtered conducting glass. The current-voltage (J–V) characteristics of the DSCs were measured with a WXS-90S-L2 Super Solar Simulator (Wacom, Tokyo, Japan) under air mass 1.5 simulated solar illuminations at 100 mW cm−2 [14]. The light intensity from 650 nm laser was controlled by applied bias to measure time course of photocurrent density.
The diffusion coefficient () of
in the electrolyte of MPImI was measured to be 3.5 × 10−7 cm2 s−1 by using the reference method [15]. The electrolyte is sandwiched by the Pt counter electrode. The diffusion coefficient is estimated from the saturated current of I–V measurement. The diffusion coefficient (DOX) in porous TiO2 is also measured by the using the porous TiO2 sandwiched by the Pt vapor deposition.
3. Results and discussion
3.1. Current profile of DSCs based on ionic liquid
Typical current-voltage (J–V) curves of DSCs based on ionic liquid are shown in figure 2. The voltage is scanned from −0.1 V to 0.8 V at a scan rate of 185 mV s−1. These J–V curves are very strange and depend on the concentration of in the electrolyte solution. In case of the concentration below 0.26 M, the decrease of current against voltage is enhanced with decrease of the concentration of
. In other words, the J–V curves became normal shape with increase of the concentration of
. The currents seem to decrease with applied bias. However, the current does not depend on the applied bias below 0.26 M as mentioned later. The information of J–V curves includes the time because the voltage is swept with scan rate. It takes about 5 s for scan from −0.1 V to 0.8 V. The current decreases with time below 5 s at lower concentration of
.
Figure 2. Current–voltage curve of dye-sensitized solar cells (DSCs) based on ionic liquid. The voltage is scanned from −0.1 V to 0.8 V at scan rate of about 185 mV s−1. The concentration of in the electrolyte is 0.05 M (····), 0.1 M (---), 0.15 M (-·-·), and 0.26 M (—).
Download figure:
Standard image High-resolution imageThe time course of the photocurrent density (Jsc) at short circuit is shown in figure 3 and is measured under 650 nm laser illumination. The time-course of photocurrent of DSCs at the concentration of 0.03 M with various light intensities is shown in figure 3(a). The degree of the current decay was enhanced with increase of the light intensity. The current decreases in 5 s over 2.7 mW cm−2 of the light intensity. On the other hand, the current becomes constant against time below 2.7 mW cm−2 of the light intensity. The current decay is strongly related to the J–V curve in figure 2. During the sweep of voltage, the current decrease with time. Therefore, the strange J–V curve is observed. Time-course of current of DSCs at 25.9 mW cm−2 of 650 nm laser with various concentrations of is also shown in figure 3(b). The decay of current against time is also observed. The current becomes constant against time with increase of the concentration of
The results are in good agreement with the relationship between the I–V curve and the concentration. These I–V curves became normal shape with increase of the concentration of
because current does not depend on the time.
Figure 3. (a) Time-course of current of DSCs at the concentration of 0.05 M with various light intensities. The 650 nm laser is used for the measurements. (b) Time-course of current of DSCs at 25.9 mW cm−2 of 650 nm laser with various concentration of .
Download figure:
Standard image High-resolution imageIn the measurement of figure 3(a), the orange color of around counter electrode disappeared after each measurement over 2.7 mW cm−2. The phenomenon means that the concentration of
becomes 0.
The concentration of I− is 0.65 M and is larger than that of . Therefore, the ion transport I– can be neglected. The concentration of
around counter electrode decreases with increase of light intensity. The lack of the
around counter electrode induces the decay of current against time. The ion transport of
from TiO2 to the counter electrode influences on the time course of photocurrent when the concentration is 0 around the counter electrode. On the other hand, the lack of
can be suppressed by the high initial concentration of
as shown in figure 3(b). The ion transport limited current is induced by the strong light intensity or lower concentration of
. The detailed model is shown in section 3.2.
The relationship between current density at short circuit and light intensity is shown in figure 4. The Jsc linearly increases with increase of the light intensity at high concentration of . The Jsc is constant against time. The Jsc at 0 s is also linearly related to the light intensity. However, the Jsc at 30 s is saturated over a light intensity at lower concentration. The current decays against time at lower concentration. The light intensity in photon flux corresponds to the amount of photons per time. The slow ion transport rate cannot cover the incident speed of photons. The saturated Jsc as shown figure 4 corresponds to the value at 30 s from 9.8 mW cm−2 to 25.9 in figure 3(a). The consistency shows the saturated Jsc is due to the ion transport in these DSCs.
Figure 4. The relationship between current density at short circuit and light intensity at the concentration of 0.05 M (●), 0.1 M (♦), 0.15 M (▲), and 0.26 M (■). The current is detected at 30 s after illumination of the white light. The 100 mW cm−2 of white light corresponds to the solar light (AM 1.5).
Download figure:
Standard image High-resolution image3.2. Charge transport in DSCs
3.2.1. Model of charge transport
The schematic structure of DSCs is shown in figure 1. The Jg is expressed as the photo-generated electron flux in TiO2 including charge injection from dye to TiO2, electron transfer from I− to oxide dye, the electron diffusion in TiO2. The Jdif is expressed as the diffusion limited current including transports of I− and I3−. The reaction rate at counter electrode also influences Jdif. However, the rate can be neglected because it is faster than ion transports. The thicknesses of spacer for DSCs and the TiO2 film are defined as T and d, respectively.
In the case of Jdif > Jg, the current in DSCs is explained by the diffusion equation of the electrons in TiO2 as the following equation [16–20]

The current of DSCs is dominated by Jg. The fast ion transports can compensate the ions for electron transfer at the interface. The Jsc is generally linear related to the light intensity below 1 sun. The response of the Jsc depends on the electron transport.
In the case of Jg > Jdif, the surface concentration of I− and I3− at interface becomes 0 because the ion transport cannot compensate the ions for electron transfer at the interface. The current depends on the ion transports. The distribution of ions in DSCs has to be considered. Papageorgiou et al [6, 7] had investigated the relationship between the charge (electron, I− and I3−) transport in the electrolyte and the photovoltaic performance by the ion transport model. The ion transport model [6, 7, 9] was modified by the followings to explain against physical parameters in DSCs and determined the suitable physical parameters for favorable ion transport in ionic liquid. (i) The light absorption profile is introduced into the ion diffusion equation. (ii) The diffusion coefficient (DOX) in porous TiO2 is different from that (
) in bulk electrolyte solution. The
can be expressed by βDOX. The β value of in the electrolyte of MPImI was 5 when the diffusion limited currents of TiO2 sandwiched by Pt electrode were measured. (iii) The current is influenced by the distribution of the concentration of
since the concentration of I− is ten times larger than that of
. (iv) The decrease of
due to charge recombination in porous TiO2 can be neglected. (v) the concentration of
at T needs to be 0 or over.
In porous TiO2 (), the concentration of
(COX) can be expressed by

where x is distance from TCO, t is time, ρ is porosity of porous TiO2 I0 is the incident photon flux corrected for reflection loss, ϕ is the electron transfer and transport yield, and α is the absorption coefficient. In the bulk electrolyte solution layer of DSCs (), the concentration (COX) of
can be expressed by

The flux (Jflux) of ion transport can be expressed by the followings. The flux () in porous TiO2 (
) is

The flux () in the bulk electrolyte solution (
) is

The concentration gradient of COX at TCO equals 0 as the boundary condition at x = 0

I assumed that two boundary condition can be fixed at x = d. Because the electrolyte solution is continuous at x = d, the COX value calculated from equation (2) must equal to that from equation (3) at x = d

The flux of equation (4) must be same as that of equation (5) at x = d

The total amount of has to be conserved in DSCs

In steady state, the left side of equations (2) and (3) equals 0. The distribution of COX can be solved from equations (2)–(9). The COX is expressed as follows

at , and
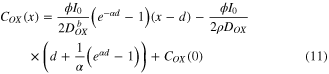
at .
When is defined as the initial concentration of
, COX(0) can be expressed by the following equation
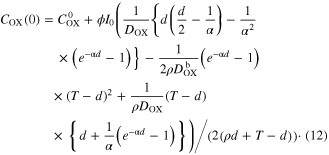
The concentration distribution (COX(0)) from TCO to the counter electrode at various light intensity is shown in figure 5(a). The T is 30 μm, d is 13 μm, ϕ is 0.65, is 0.03 M,
is 3.5 × 10−7 cm2 s−1, β is 5, α at 650 nm is 1200 cm−2, respectively. As the light intensity increases, the COX(0) increases and COX(T) decreases.
Figure 5. (a) The calculated concentration distribution (COX(x)) of in a DSC at the concentration of 0.05 M with the light intensity of 2.8 mW cm−2 (····), 3.7 mW cm−2 (‐·‐·), 4.6 mW cm−2 (‐‐‐) and 5.5 mW cm−2 (—). The red solid line is the COX(x) at 9.2 mW cm−2 and becomes minus at x = 30 μm. The right side is TCO, and the left side is the counter electrode. The T is 30 μm, d is 13 μm, ϕ is 0.65,
is 0.05 M,
is 3.5 × 10−7 cm2 s−1, β is 5, α at 650 nm is 1200 cm−2, respectively. (b) The relationship between calculated Jsc and the light intensity at the same condition of (a). The black circle is due to this model. The Jsc is constant when COX(T) becomes 0. The white circle is the value calculated from equation (13) when COX(T) < 0 is permitted in this model.
Download figure:
Standard image High-resolution imageIn the case of Jdif > Jg, the photocurrent density of DSCs can be expressed as ion flux at x = T
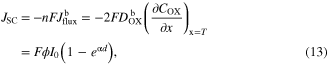
where n is electrons per this reaction and F is Faraday constant, respectively.
The linear relationship between photocurrent and light intensity can be explained by equation (13).
COX(T) becomes minus at light intensity of 3.1 mW cm−2 as shown in figure 5(a). In a real device, COX(T) of equation (11) has to be 0 or over as described as (v). The model needs to be modified to explain the ion transport limitation. When COX(T) is calculated to be under 0, the COX(T) must keep being 0, and equation (8) is neglected. This assumption means that (dCOX/dx)x=T becomes constant against I0. JSC is dominated by the diffusion of and becomes constant against I0 defined as diffusion limited current (
). The constant
is due to (dCOX/dx)x=T of equation (13). The relationship between the calculated Jsc and the light intensity (I0) is shown in figure 5(b). The calculated
from figure 5(b) was 1.3 mA cm−2, corresponding to the experimental results in figures 3(a) and 4.
3.2.2. Saturated photocurrent versus diffusion coefficient of
or initial concentration
has to be over 30 mA cm−2 which is calculated from the external quantum efficiency (EQE) of 95% from 400 nm to 900 nm in solar light. Otherwise, the solar to energy conversion efficiency of DSCs is limited by the ion transport. The diffusion limited current density (Jdif) can be generally expressed by the following formula [15]

Jdif depends on the diffusion coefficient of ions (D), carrier density (C), and the distance (d) of the ion transport, which respectively corresponds to the diffusion coefficient() of
, the
value of
, and the d value of the TiO2 film. Therefore, each parameter needs to be discussed to improve
.
The relationship between the calculated and
or
is shown in figure 6.
increases with increase of
as shown in figure 6(a). The relationship between
and
is related to the
. The
also increases with increase of
as shown in figure 6(b). In case of
= 0.05 M, the
needs to be over 1 × 10−5 cm2 s−1. The typical
value is 0.05 M in the electrolyte solution based on acetonitrile. The
value of this electrolyte solution is about 1 × 10−4 cm2 s−1. Therefore, the electrolyte solution based on acetonitrile can fully compensate the photon flux of the solar light. On the other hand, the
based on a typical ionic liquid is 5 × 10−7 cm2 s−1, about 100 times smaller than that of the electrolyte solution based on acetonitrile.
becomes about 1% of the electrolyte solution based on acetonitrile.
Figure 6. (a) The calculated saturated current density () versus the diffusion coefficient (
) at the initial concentration (
) of 0.05 M (●), 0.1 M (△), 0.15 M (■), 0.2 M (○), and 0.25 M (◆). (b) The plot of calculated
versus
at the
of 5 × 10−6 (●), 2 × 10−6 (△), 1.5 × 10−6 (□),1 × 10−6 (▲), 5 × 10−7 (■), 3.5 × 10−7 (○) and 1 × 10−7 cm2 s−1 (◆). The other parameters of these calculations are same as figure 5.
Download figure:
Standard image High-resolution imageThe electrolyte solution with large value needs to be prepared to improve
. In the case of MPImI, the
value has to be over 0.26 M. However, the I2 cannot be dissolved over 0.26 M in this electrolyte. And the strong light absorption of
reduces the light harvesting efficiency of the dye on TiO2 in DSCs. The way to increase
is limited by the solubility and the light absorption of
.
Molecular design has been used to try to decrease the viscosity of an ionic liquid [3, 5] because increases with decrease of viscosity. A suitable
value for DSCs needed to be shown for the design of the ionic liquid.
needs to be over 1.5 × 10−6 cm2 s−1 from figure 6(b) and the
needs to be over 0.1 M to certainly enhance
. The high solubility (>0.3 M) and low visible absorption properties of a redox mediator also need to be designed for DSCs.
3.2.3. Saturated photocurrent influenced by meso-porous layer of TiO2
The effect of porous TiO2 on the ion transport also needs to be discussed to improve . The plots of calculated
versus
or
again are shown in figure 7 to compare with the each diffusion limited current density (Jdif) of the porous TiO2 film and the bulk electrolyte in the DSC. The solid line in figure 7(a) is due to Jdif of the porous TiO2 layer and is calculated from equation (14) at C =
= 0.05 M, d = 13 μm, and D = DOX, respectively. The dashed line is due to Jdif of the bulk electrolyte and is calculated from equation (14) at C =
= 0.05 M, d = 17 μm, and D =
, respectively. The slope of
versus
is similar to that of Jdif versus
of the porous TiO2 layer. The solid line in figure 7(b) is due to Jdif of the porous TiO2 layer and is calculated from equation (14) at C =
, d = 13 μm, and D = DOX = 7 × 10−8 cm2 s−1, respectively. The dashed line is due to Jdif of the bulk electrolyte and is calculated from equation (14) at C =
, d = 17 μm, and D =
= 3.5 × 10−7 cm2 s−1, respectively. The slope of
versus
is also similar to that of Jdif versus
of the porous TiO2 layer. The results show that the ion transports in the porous TiO2 layer influence the total ion transport in DSCs.
Figure 7. (a) The plot of calculated versus
at the C0OX of 0.05 M (●). The solid line is calculated from equation (14) at C =
= 0.05 M, d = 13 μm, and D = DOX, respectively. The dashed line is calculated from equation (14) at C =
= 0.05 M, d = 17 μm, and D =
, respectively. (b) The plot of calculated
versus
at the
of 3.5 × 10−7 cm2 s−1(●). The solid line is calculated from equation (14) at C =
, d = 13 μm, and D = DOX = 7 × 10−8 cm2 s−1, respectively. The dashed line is calculated from equation (14) at C =
d = 17 μm, and D =
= 3.5 × 10−7 cm2 s−1, respectively. The other parameters of these calculations are the same as in figure 5.
Download figure:
Standard image High-resolution imageThe β is defined as . The DOX is usually smaller than
(β > 1). The mechanism of β > 1 is under investigation. The β value seems to depend on the viscosity. The ions can move more randomly when the viscosity is low. Ions easily collide with the surface of porous structure. Therefore, the diffusion rate of ions slows down in porous TiO2. On the other hand, the β value seems to decrease with increase of viscosity of the electrolyte. In the case of high viscosity, ions cannot quickly move in the electrolyte solution. The probability of the collision between ions and the surface of porous structure must become smaller.
The relationship between the calculated and thickness (d) of TiO2 is shown in figure 8. The results can be explained by using equation (14). The ion can be easily supplied with decrease of the distance.
is exponentially influenced by the d value. The thin TiO2 films are favorable for the ion transport, especially in the case of small
. However, the light harvesting efficiency of dye on TiO2 decreases with decrease of d. The high light absorption ability of dyes needs to be designed for DSCs based on ionic liquid. In the case of
= 0.05 M,
needs to be 5 × 10−5 cm2 s−1, corresponding to the condition of figure 6(a).
Figure 8. The calculated versus thickness (d) of TiO2 at the
of 5 × 10−5 (●), 5 × 10−6 (△), 1 × 10−6 (■), 5 × 10−7 (□), 1 × 10−7 cm2 s−1 (◆). The other parameters of these calculations are the same as in figure 5.
Download figure:
Standard image High-resolution image4. Conclusion
The effect of transport in the liquid electrolyte on the photovoltaic properties of dye-sensitized solar cells (DSCs) was investigated by the ion transport model and experimental results. The Jsc becomes constant against light intensity when the concentration (COX(T)) of
at the counter electrode becomes 0. The saturated Jsc is named as the saturated current (
) and depends on the ion transport in the electrolyte solution of DSCs. The
value has to be over 30 mA cm−2 which is calculated from EQE of 95% from 400 nm to 900 nm in solar light. The
value can be determined by the thickness (d) of the TiO2 layer, the initial concentration (
), and the diffusion coefficient (
) of
. The
value can increase with increase of
and
and with decrease of d. In the case of
= 0.05 M,
needs to be 5 × 10−5 cm2 s−1. Otherwise, the d value has to be below 10 μm. The
value can increase with increase of the
value. Because I2 cannot dissolve over 0.26 M in the ionic liquid, the
needs to be over 1.5 × 10−6 cm2 s−1 to keep over 30 mA cm−2 of
.
Acknowledgments
This work was supported by Precursory Research for Embryonic Science and Technology (PRESTO) of Japan Science and Technology agency. The authors gratefully acknowledge the kind support for using the evaluation machine or the measurement systems of devices by Dr Liyuan Han.
Footnotes
- *
Invited talk at the 7th International Workshop on Advanced Materials Science and Nanotechnology IWAMSN2014, 2-6 November, 2014, Ha Long, Vietnam.