Abstract
Tissue engineering offers a great potential in regenerative dentistry and to this end, three dimensional (3D) bioprinting has been emerging nowadays to enable the incorporation of living cells into the biomaterials (such a mixture is referred as a bioink in the literature) to create scaffolds. However, the bioinks available for scaffold bioprinting are limited, particularly for dental tissue engineering, due to the complicated, yet compromised, printability, mechanical and biological properties simultaneously imposed on the bioinks. This paper presents our study on the development of a novel bioink from carboxymethyl chitosan (CMC) and alginate (Alg) for bioprinting scaffolds for enamel tissue regeneration. CMC was used due to its antibacterial ability and superior cell interaction properties, while Alg was added to enhance the printability and mechanical properties as well as to regulate the degradation rate. The bioinks with three mixture ratios of Alg and CMC (2–4, 3–3 and 4–2) were prepared, and then printed into the calcium chloride crosslinker solution (100 mM) to form a 3D structure of scaffolds. The printed scaffolds were characterized in terms of structural, swelling, degradation, and mechanical properties, followed by their in vitro characterization for enamel tissue regeneration. The results showed that the bioinks with higher concentrations of Alg were more viscous and needed higher pressure for printing; while the printed scaffolds were highly porous and showed a high degree of printability and structural integrity. The hydrogels with higher CMC ratios had higher swelling ratios, faster degradation rates, and lower compressive modulus. Dental epithelial cell line, HAT-7, could maintain high viability in the printed constructs after 1, 7 and 14 d of culture. HAT-7 cells were also able to maintain their morphology and secrete alkaline phosphatase after 14 d of culture in the 3D printed scaffolds, suggesting the capacity of these cells for mineral deposition and enamel-like tissue formation. Among all combinations Alg4%–CMC2% and in a less degree 2%Alg–4%CMC showed the higher potential to promote ameloblast differentiation, Ca and P deposition and matrix mineralization in vitro. Taken together, Alg-CMC has been illustrated to be suitable to print scaffolds with dental epithelial cells for enamel tissue regeneration.
Export citation and abstract BibTeX RIS

Original content from this work may be used under the terms of the Creative Commons Attribution 4.0 license. Any further distribution of this work must maintain attribution to the author(s) and the title of the work, journal citation and DOI.
1. Introduction
Enamel is the hardest tissue in our body and tooth decay called also dental caries is one of the most common human infectious diseases [1]. Destruction of the enamel tissue results in dental pain and dental infection that can spread in different organs affecting systemic health. Among all dental tissues dental enamel is the most challenging to generate because dental epithelial cells disappear by apoptosis before tooth eruption and no dental epithelial stem cells survive in dental crown [2]. The current approach to treat tooth decay includes removing the parts of the tooth affected by dental caries and replacing them with artificial dental restoration materials. However, given the limitations associated with dental restoration materials such as inability to mimic the structural and functional characteristics of the native dental tissue, a great deal of attention has been attracted towards developing novel alternative approaches to treat dental caries [3].
Extended research is emerging in the field of enamel applying material-cell-based strategies. Among other strategies, bio-fillings that can be produced by dental stem cells could offer an ideal solution to biologically regenerate/restore the affected enamel tissue [3]. HAT-7 is a dental epithelial cell line generated by rat dental stem cells that has been used to study ameloblast differentiation and enamel formation [4]. Such epithelial stem cells could be used as a base for new promising approaches that would employ the three main elements of tissue regeneration i.e. stem cells, scaffolds and signaling factors with hopes to produce bioengineered enamel tissue to repair the damaged structures [5–7].
Among different scaffold fabrication methods, three-dimensional (3D) printing has a great potential to fabricate customized scaffolds, based on magnetic resonance imaging or computerized tomography scans taken from the patient, and offers the incorporation of living cells and growth factors in the printed scaffolds [8, 9]. During the process of scaffold fabrication using extrusion-based 3D printing (one of the currently available 3D printing techniques that dispenses materials by using a mechanical extruder), cells are incorporated into a biomaterial called bioinks, that are usually hydrogels, and then the cell-laden bioink is dispensed in a layer by layer fashion to fabricate constructs with the desired design [10, 11]. Development of suitable bioinks that possess printability, and mechanical and biological properties that support cell functions such as cell attachment, proliferation and migration and the formation of new tissue is a key element to fabricate 3D printed constructs to provide a suitable microenvironment for successful applications in tissue engineering [12, 13]. Bioinks also need to become crosslinked quickly, before or after printing, to retain their shape fidelity and have proper degradation rate that is ideally matched to the rate of new tissue formation, in vitro or in vivo and decent mechanical properties, to be able to hold the scaffold's shape during in vitro culture or in vivo transplantation and matched to the mechanical properties of the target tissue, in the case of enamel regeneration, the mechanical properties of the bioink should ideally be close to the mechanical properties of enamel organic matrix that was found to be a gel-like environment in which natural enamel development occurs [13–19]. Various hydrogels based on biomaterials of natural (e.g. alginate (Alg), agarose, hyaluronic acid, chitosan, dextran, collagen, gelatin and fibrin) and synthetic (e.g. polyethylene glycol (PEG), PEG diacrylate) origins have been used in dental tissue engineering applications [20]. However, there is a remarkable lack of hydrogel bioinks that have been primarily developed for bioprinting dental cells [21]. Thus, this study aimed to address this emerging need for bioinks for dental tissue engineering.
Chitosan is a cationic polysaccharide extracted from chitin that exhibits remarkable inherent properties such as antimicrobial and mucoadhesive characteristics, and biocompatibility, that makes it a suitable biomaterial for various biomedical applications [22]. In particular, the antimicrobial properties of chitosan offer promises for dental applications [22, 23]. In addition, the presence of positively charged amino groups in chitosan enables this polymer to interact with cell membrane and also to bind to growth factors, glycosaminoglycans and DNA [24–27]. Thus, chitosan has been reported to improve cell adhesion, proliferation, and differentiation and offers promises for biopriting and tissue engineering applications [28–30]. However, the application of chitosan hydrogels as bioinks for 3D printing applications is still under investigation [13]. Chitosan is soluble only in acidic solution that limits its application for bioprinting due to the incompatibility of cells with acidic environment [31, 32]. Carboxymethyl chitosan (CMC) is a water-soluble derivative of chitosan that can be used as a bioink for cell printing. Nevertheless, CMC itself is not considered a good candidate for bioink owing to its extremely high degradation rate [32]. In addition, CMC possess poor mechanical properties, which makes the fabrication of scaffold structures challenging [33]. Several studies have attempted to develop CMC-based bioinks by blending CMC with other polymers [32]. Alg is a non-toxic and non-immunogenic natural polymer [34] that has been used in various biomedical applications including peptide or protein delivery [35–37], wound dressing [38] and cell encapsulation [39–41]. Alg has been widely used in bioprinting [42–46] due to its fast gelation through ionic crosslinking in the presence of divalent ions [47]. However, Alg has shown slow degradation kinetics and limited cell interaction properties [34]. To address the limitations of Alg and CMC as a single component bioinks, we developed a novel two-component bioink composed of these two polymers with improved characteristics including good printability, proper degradation rate, enhanced mechanical properties, increased cell affinity and antibacterial properties [48]. Furthermore, similar to Alg, CMC is also able to become crosslinked with calcium ions [49].
While both Alg and CMC have been employed as bioinks alone [50, 51] or in mixture with other polymers [32, 52–61], the suitability of a combination of Alg and CMC as a bioink has not been studied for bioprinting, neither their applications for dental tissue engineering. To best of our knowledge, this is the first study investigating the suitability of Alg-CMC bioinks for both bioprinting and tooth tissue engineering applications. For this purpose, we examined the effect of various weight ratio of Alg and CMC (Alg4%–CMC2%, Alg3%–CMC3%, Alg2%–CMC4%) on structural and mechanical properties of 3D printed scaffolds as well as their swelling ability, degradation rate and biological properties for the encapsulation of HAT-7 cells, that could potentially facilitate cellcell interactions and guide dental epithelial cells towards ameloblast differentiation and the generation of bioengineered tooth with desired size and shape [62], and the potential application of the characterized bioink for enamel regeneration.
2. Materials and methods
2.1. Preparation of hydrogel solutions
Medium viscosity sodium Alg powder (Cat. no. A2033, Sigma Aldrich, St. Louis, Missouri, USA) and CMC powder (Cat. no. sc-358 091, Santacruz Biotechnology, Dallas, Texas, USA) were dissolved in distilled water and mixed under magnet stirring at room temperature to prepare 2% (w/v) Alg –4% (w/v) CMC (Alg2%-CMC4%), 3% (w/v) Alg –3% (w/v) CMC (Alg3%-CMC3%) and 4% (w/v) Alg –2% (w/v) CMC (Alg4%–CMC2%) solutions.
2.2. 3D printing of Alg-CMC hydrogel
Scaffolds were designed and sliced using Magics13 EnvisionTEC software (V13, Materialise NV, Leuven, Belgium) and Bioplotter RP software (V3, EnvisionTEC GmbH, Gladbeck, Germany). 31 layers of cuboid scaffolds with the latticed structure (10 mm × 10 mm × 5 mm) were fabricated by using a pneumatic 3D Bioplotter (EnvisionTEC GmbH, Gladbeck, Germany) with 1 mm distance between strands and 0°–90° strand orientation. Prior to printing, the wells of 12-well plates were treated with 0.1% (w/v) polyethyleneimine solution (PEI, M.W. 60 000 g mol−1, Cat. no. J61270, Alfa Aesar, Tewksbury, MA, USA) and incubated in the incubator at 37 °C for 24 h. Then, the PEI solution was removed, and 50 mM calcium chloride (CaCl2, Cat. no. 223 506, Sigma Aldrich) solution containing 0.1% (w/v) PEI was added to the wells. Hydrogel solutions were printed at a needle speed of 20 mm s−1 and at a certain extrusion pressure (Alg2%–CMC4% for 0.2 bar, Alg3%–CMC3% for 0.4 bar, Alg4%–CMC2% for 0.7 bar) through 27-gauge tapered needles with an inner diameter of in the crosslinker bath. After printing, the crosslinking solution was replaced with fresh 100 mM CaCl2. After 10 min, the calcium chloride solution was removed, and the scaffolds were rinsed with distilled water twice and kept in distilled water until further analysis. Strand size and pore size were measured using image J software (version 2.3.0/1.53f, NIH, Bethesda, Maryland, USA) as the strand diameter and the space between two adjacent strands. Strand printability and pore printability were also calculated using the following equations [63]:
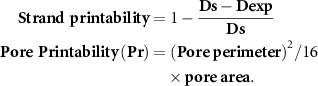
2.3. Swelling and degradation test
The ability of the 3D printed scaffolds to uptake water was assessed by the swelling test. The printed scaffolds (15 layers) were freeze-dried and then weighted to record the initial dry weight (Wdi). The scaffolds (n = 3) were immersed in phosphate buffer saline (PBS) and incubated at 37 °C and 5% CO2. At different time points (Day 1, 7, 14, 21), the samples were taken from PBS and blotted with a Kimwipe tissue paper (Kimberly-Clark Professional, Irving, Texas, USA) to eliminate the residual PBS and then the swollen weight was recorded (Ws). The scaffolds were freeze-dried and weighed to record the final dry weight (Wdf). For each time points, the swelling ratio (SR) and remaining weight were calculated using the following equations:


2.4. Mechanical test
The mechanical properties of the 3D printed scaffolds (13 layers, 10 mm × 10 mm × 2 mm) were assessed by uniaxial unconfined compression test using Biodynamic 5010 (Bose Corporation, Eden Prairie, Minnesota, USA) testing machine under speed rate of 0.01 mm s−1 after 24 h incubation in distilled water. The test was performed in a wet state at room temperature in the air without immersing in water during the experiment five times for each group (n = 5). Samples were compressed for the maximum displacement of 70% of the scaffolds thickness. The elastic modulus was calculated by measuring the slope of the linear part of the stress–strain curves for each scaffold.
2.5. Microstructural evaluation of the 3D printed hydrogel scaffolds
The microstructure of the 3D printed scaffolds was assessed by scanning electron microscopy (SEM). 3D printed scaffolds were freeze-dried and then sputter coated with a chromium film using a sputter coater (Q150T ES, Quorum Technologies, Laughton, East Sussex, UK) and then imaged under a field SEM (Hitachi High-Tech SU8010, Chiyoda, Tokyo, Japan).
2.6. 3D imaging using synchrotron radiation-based x-ray for visualizing 3D printed hydrogel scaffolds
External geometry and porous structure of the interior of the 3D printed scaffolds were visualized by Synchrotron Radiation-based x-ray imaging technique at the 05B1-1 beamline at the Canadian Light Source (CLS, University of Saskatchewan, Saskatoon, Canada). Hydrogel scaffolds (31 layers, 10 mm × 10 mm × 5 mm) were printed and kept in CaCl2 for four days and then immersed in distilled water in a 15 ml conical centrifuge tube and fixed with Styrofoam. Then, samples were imaged using filtered white beam with a detector placed 0.2 m from the sample using the Optique Peter White Beam microscope (Lentilly, France) with the pixel size of 3.6 μm. 3000 projections were acquired during each CT scan with an exposure time of 20 milliseconds per projection. Strand and pore size were measured using six different images for each group of the scaffolds.
2.7. Cell culture
The dental epithelial cell line originating from the cervical loop epithelium of a rat incisor HAT-7 (CVCL_RW36, kindly provided by Dr Hidemitsu Harada, Iwate Medical University, Iwate, Japan) [64] was cultured in DMEM/F12 (1:1), HEPES medium (Cat. no. 11 330-032, Gibco®, Invitrogen, Carlsbad, California, USA) supplemented with 10% fetal bovine serum (FBS, Cat. no. 12 483-020, Gibco®, Invitrogen) and 1% antibiotic (PenicillinStreptomycin 100X solution, 10 000 units ml−1 Penicillin/101 000 Streptomycin, Cat. no. SV30010, HycloneTM, Logan, Utah, US) and incubated at 37 °C and 5% CO2. Medium was changed every 2 d.
2.8. Bioprinting of HAT-7 cell-laden hydrogel scaffolds
Alg and CMC powders were sterilized by exposure to UV light for 90 min. Then, hydrogel solutions were prepared by mixing the Alg and CMC powders in saline solution under sterile conditions. 12-well cell culture plates were coated with autoclaved PEI solution (0.1% w/v) in the incubator 24 h before printing to enhance the surface adhesion to the ink. The main concept behind treating plates beforehand with PEI is to achieve improved attachment of Alg strands to the culture plate during bioprinting. PEI is a synthetic polymer, which is positively charged, while Alg is a negatively charged polymer; thus, addition of Alg to the plates treated with PEI causes a strong ionic interaction [65]. After two passages, cells were trypsinized, counted and cell pellets with a density of 6 × 106 cells ml−1 were made and then mixed in the sterile hydrogel solutions by using 1 ml pipets. The cells incorporated hydrogel solutions were loaded into the bioplotter syringe and 2–3 layers of cell-laden scaffolds were printed into the autoclaved 50 mM CaCl2 crosslinking bath containing 0.1% PEI. After printing, the printing bath was replaced with 100 mM CaCl2 solution to further crosslink scaffolds for another 10 min. Then, scaffolds were rinsed with culture medium three times and immersed in culture media containing 10% FBS and 1% antibiotics and cultured in the incubator at 37 °C and 5% CO2.
2.9. Assessment of cell viability using live/dead assay
Viability of HAT-7 cells within the 3D printed scaffolds were evaluated after 1, 7 and 14 d of culture using the LIVE/DEAD Cell imaging kit (Cat. no. R37601, Invitrogen, Thermo Fisher Scientific, USA) according to the manufacturer's instructions. Briefly, cell-laden scaffolds were stained with the mixed green/red dye and incubated for 15 min in the incubator at 37 °C and 5% CO2, followed by imaging using fluorescent microscope (EVOS M5000 Cell Imaging System, Thermo Fisher Scientific). The number of live (green) and dead (red) cells were measured by using image J and viability was calculated with the following equation:

2.10. Observation of cell morphologies in 3D printed scaffolds
After 14 d of culture, the morphology of the HAT-7 cells within the 3D printed hydrogel scaffolds was observed using SEM. Cell incorporated scaffolds were washed with PBS twice and immersed in 2.5% glutaraldehyde solution prepared in PBS for 2 h. The constructs were then rinsed with PBS and distilled water three times each. Afterwards, the scaffolds were lyophilized, and sputter coated with a thin layer of chromium and imaged using the same field SEM as previously mentioned.
2.11. Assessment of gene expression
Scaffolds were washed with PBS and immersed in 0.5 M EDTA (Sigma, Cat no E6511-100g) solution in PBS with agitation at 150 rpm for 5 min. Then cells in suspension were pelleted at 1000 rpm for 5 min. Total RNA was extracted using RNeasy Mini Kit (Cat. no. 74106, QIAGEN, GmbH, Hilden, Germany), followed by cDNA synthesis using the High-Capacity cDNA Reverse Transcription Kit (Cat. no. 4368814, Applied Biosystems™, Foster City, California, US) according to the manufacturer's instructions. mRNA expression levels of dentin sialophosphoprotein (DSPP), ameloblastin (AMBN), enamelin (ENAM), kallikrein-4 (KLK4), calcium release-activated calcium channel protein 1 (ORAI1), alkaline phosphatase (ALP) genes were measured by quantitative Reverse Transcription Polymerase Chain Reaction (qRT-PCR) using Power SYBR™ Green PCR Master Mix (Cat. No. 4367659, Thermo Fisher Scientific, Invitrogen) and designed primers (table 1). The relative expression was calculated using DD C method and GAPDH as a housekeeping gene.
Table 1. Sequence of primers used for qRT-PCR.
Primers | Forward | Reverse |
---|---|---|
DSPP | GTGCTAAAGAACGCGAGGAC | GTCTCCATTCTGGCTCGTGT |
AMBN | CAGAAGGCTCTCCACTGCAA | CCCCAAGGGTGTGGTAACAT |
ENAM | GATGCCCATGTGGCCTCCACCA | GCCAAATGGTGGGAATGGCTGA |
KLK4 | CTGGGGTACCTCATCCTTGA | CCACGGTGTAGGAGTCCTGT |
ORAI1 | GCTCTGCTGGGTGAAGTTCT | AAGTGAACGGCAAAGACGAT |
ALP | TGATCACTCCCACGTTTTCA | CTGGGCCTGGTAGTTGTTGT |
GAPDH | AACAGCAACTCCCACTCTTC | TGGTCCAGGGTTTCTTACTC |
2.12. ALP staining
After 14 d of culture, 3D printed cell-laden constructs were rinsed with PBS twice, and cells within the constructs were fixed and permeabilized using BD Cytofix/cytopermTM fixation/permeabilization kit (Cat. no. 554714, BD Biosciences, San Jose, California, USA). In brief, constructs were incubated in the fixation and permeabilization solution for 30 min at 4 °C, followed by washing with 1× BD Perm/WashTM buffer twice and rinsing with PBS twice. Then, ALP expression in the cell-laden constructs was detected using the SIGMAFASTTM BCIP®/NBT detection kit (Cat. no. B5655, Sigma-Aldrich). The staining reagent was prepared according to the manufacturer's instructions, added to the constructs, and incubated for 24 h at 37 °C under dark conditions. Then, constructs were imaged using a microscope light microscope (EVOS M5000 Cell Imaging System, Thermo Fisher Scientific).
2.13. SEM and energy-dispersive x-ray spectrometry (EDX) for evaluating cell morphology, Ca and P matrix content and mineral deposition in 3D cell culture models
Cells incorporated in scaffolds were washed with PBS and fixed with 2.5% glutaraldehyde at RT for 2 h, followed by three washes with PBS and distilled water. Afterward, scaffolds were placed at −80 overnight, followed by subsequent freeze-drying. Samples were coated with a thin layer of chromium using a sputter coater (Q150T ES Quorum Technologies, Laughton, East Sussex, UK) and imaged under a field SEM (SU8010, Hitachi, Chiyoda, Tokyo, Japan) with an accelerating voltage of 3 kV. EDX (EDX Ultim Max) was done using a voltage of 8 kV.
2.14. Statistical analysis
All data are given as mean standard deviation. Statistical analysis was performed using one-way ANOVA followed by the post-Tukey test using GraphPad Prism 5 (GraphPad Software, San Diego, California, USA). A p value < 0.05 was considered statistically significant.
3. Results
3.1. Comparative analysis of 3D printed scaffolds at different ratios of Alg to CMC based on the assessment of the structural features
To investigate the influence of Alg and CMC concentrations in hydrogel solution on printability, three different compositions including Alg2%–CMC4%, Alg3%–CMC3%, Alg4%–CMC2%, were printed using 200 μm needle at the same needle speed of 20 mm s−1 (figure 1). The total polymer concentration in the hydrogel solutions was kept constant at 6% (w/v). In addition, the crosslinker concentration and crosslinking time was kept at 100 mM and 10 min, respectively. It was observed that the Alg2%–CMC4% hydrogel solution required lowest extrusion pressure (0.2) to be dispensed as strands as compared to Alg3%–CMC3% and Alg4%–CMC2% hydrogel solutions that needed 0.4 and 0.7 bar dispensing pressure, respectively. This showed that the concentration of Alg polymer in the Alg-CMC blend hydrogels has a larger impact on the viscosity of the ink and thereby on the extrusion pressure required to dispense ink out of the nozzle.
Figure 1. Characterization of alginate-carboxymethyl chitosan (Alg-CMC) printability. (a) Macroscopic view of 31 layers (10 mm × 10 mm × 5 mm) of Alg-CMC scaffolds. (b), (c) Macroscopic and microscopic images of extruded strands printed using different pressure (0.2 bar for Alg2%–CMC4%, 0.4 bar for Alg3%–CMC3%, 0.7 bar for Alg4%–CMC2%) at the same needle speed of 20 mm s−1. (d) Comparative analysis of strand size (d), pore size (e), strand printability (f), and pore printability (g) in 3D printed scaffolds. The X-axis in the graphs shows different groups of hydrogels with varying concentrations of Alg and CMC (Alg2%–CMC4%, Alg3%–CMC3%, Alg4%–CMC2%). Error bars represent the mean ± SD of three experimental replicates. (*in the graphs indicates the statistical significance between three different groups of scaffolds, ***p < 0.001).
Download figure:
Standard image High-resolution imageThe strand size, pore size, strand printability and pore printability of the printed strands were also determined after printing two layers (figure 1). The strand size was found to be significantly higher (p < 0.001) in Alg3%–CMC3% (0.570 0.035 mm) and Alg4%–CMC2% (0.588
0.029 mm) scaffolds as compared to Alg2%–CMC4% (0.340
0.060 mm). However, no significant difference was observed in the strands size of Alg3%–CMC3% and Alg4%–CMC2% scaffolds. Likewise, significantly higher pore sizes (p < 0.001) were observed in Alg3%–CMC3% (0.492
0.037 mm) and Alg4%–CMC2% (0.453
0.029 mm) scaffolds as compared to Alg2% CMC4% (0.716
0.054 mm). Although strand printability in all groups was higher than the acceptable range which was previously reported to be around 0.9–1.1 [63], their pore printability was in this range (figure 1).
3.2. Microstructural evaluation of the 3D printed hydrogel scaffolds
The microstructure of the 3D printed hydrogels was observed under SEM (figure 2) and indicated that the scaffolds were printed as highly porous structures with well-defined intersections as designed. It was observed that scaffolds with higher ratios of CMC had smaller filament size and were able to maintain the pores with better square shaped morphology and large sizes during the printing step.
Figure 2. The scanning electron microscopy (SEM) of the 3D printed hydrogels at three different alginate–carboxymethyl chitosan weight ratios showed the highly porous structure of the scaffolds (a-1, b-1, c-1) and well-defined intersections (a-2, b-2, c-2). (1) Image's magnification is 1.00 mm. (2) Image's magnification is 500 µm.
Download figure:
Standard image High-resolution image3.3. 3D visualization of the scaffolds using synchrotron-based x-ray imaging
The 3D structure of the 3D printed hydrogels was visualized with synchrotron-based x-ray imaging. As shown in supplemental figure 1, the multilayers of the hydrogel scaffolds were successfully printed with high structural integrity and stability. Our data also revealed the internal structure lattice of the scaffolds. Hydrogel scaffolds with higher percentage of CMC (Alg2%–CMC4% and Alg3%–CMC3%) could maintain the square-shape of pores better than Alg4%–CMC2% hydrogels which is in agreement with the SEM images. Alg4%–CMC2% hydrogels showed a significantly larger strand size compared to Alg2%–CMC4% and Alg3%–CMC3%, which occupy a larger space between strands and resulted in significantly smaller pore size (supplemental figure 1).
3.4. Swelling and degradation test and assessment of the effect of the concentrations of Alg and CMC on mechanical properties of the 3D printed scaffolds
The ability of 3D printed hydrogel scaffolds to uptake water was characterized by measuring the SR at different time points. As shown in figure 3, the swelling ratio of Alg2%–CMC4% (28.12 1.282) was significantly higher (p < 0.01) than Alg3%-CMC3% (23.47
1.249) and Alg4%–CMC2% (23.50
0.319) at day 1. At day 7, the SR of all three groups (Alg2%–CMC4%: 40.25
3.742, Alg3%–CMC3%: 40.96
1.975, Alg4%–CMC2%: 39.94
1.065) was significantly increased compared to that of the same groups at day 1 (p < 0.01 for Alg2%–CMC4% and p < 0.001 for Alg3%–CMC3% and p < 0.001 Alg4%–CMC2%). However, there was no significant difference between the SRs of all three groups at day 7 (P > 0.05). At day 14, the SR of Alg2%–CMC4% (35.69
1.643) was significantly lower than Alg3%–CMC3% (43.16
1.079, p < 0.01) and Alg4%–CMC2% (39.44
1.520, p < 0.05). The difference between SRs of Alg3%–CMC3% (43.16
1.079) and Alg4%–CMC2% (39.44
1.520) was also significant (p < 0.05). At day 21, Alg4%–CMC2% scaffolds showed a significantly higher (p < 0.05) SR (43.58
0.713) compared to Alg2%CMC4% (30.38
6.723). However, no significant difference (P > 0.05) was observed between SR of Alg3%–CMC3% (35.49
0.339) and those of two other groups: Alg4%–CMC2% (43.58
0.713) and Alg2%–CMC4% (30.38
6.723). Both Alg2%–CMC4% and Alg3%–CMC3% reached to their maximum SR at day 7, however, the SR of Alg4%–CMC2% was at its highest level at day 21.
Figure 3. Characterization of mechanical properties of the 3D printed hydrogels at three different alginate–carboxymethyl chitosan weight ratios: (a) swelling ratio, (b) remaining weight of 3D printed scaffolds with varying concentrations of alginate and carboxymethyl chitosan (Alg2%–CMC4%, Alg3%–CMC3%, Alg4%–CMC2%). (c) Images of the 3D printed scaffolds after being crosslinked in CaCl2 solution for 10 min (Day 0) and degraded scaffolds after 21 d incubation in PBS. (d) Characterization of mechanical behaviour of 3D printed scaffolds with three different concentrations of alginate and carboxymethyl chitosan (Alg2%–CMC4%, Alg3%–CMC3%, Alg4%–CMC2%) obtained from uniaxial compression test with the Y-axis representing compressive stress and the X-axis showing the strain. (e) compressive modulus (Y-axis) calculated from the slope of the linear region of the stress–strain curves showing an increase in mechanical properties of the scaffolds with an increase in the concentration of alginate to carboxymethyl chitosan. The X-axis in the graphs shows different groups of hydrogels with varying concentrations of alginate and carboxymethyl chitosan (Alg2%–CMC4%, Alg3%–CMC3%, Alg4%–CMC2%). Error bars represent the mean ± SD of five experimental replicates. (* in the graphs indicates the statistical significance between three different groups of scaffolds, ***p < 0.001, assessed using one-way ANOVA followed by the post-Tukey test).
Download figure:
Standard image High-resolution image
In vitro degradation of scaffolds was assessed after 1-, 7-, 14- and 21 d incubation in PBS (figure 3). At all-time points of day 1, 7 and 14, the remaining weight in Alg2%–CMC4% was lower compared to Alg3%–CMC3% and Alg4%–CMC2% except at day 1 when no significant difference was observed between Alg2%–CMC4% and Alg3%–CMC3%. The remaining weight of Alg2%–CMC4% scaffolds was significantly decreased from 94.1 2.33 at day 1 to 68.42
4.946 (p < 0.01) at day 14 and 69.60
8.273 at day 21 (p < 0.01). No significant difference was observed between remaining weight at day 1 and day 7 (82.55
4.930). Likewise, the remaining weight of Alg3%–CMC3% scaffolds was significantly decreased at day 21 (86.97
4.201) compared to the three other time points: day 1 (101.8
3.149, p < 0.001), day 7 (94.68
1.723, p < 0.05) and day 14 (95.23
0.204, p < 0.05). The weight of Alg4%–CMC2% scaffolds remained unchanged during 21 d of degradation test with no significant difference (P > 0.05) between their remaining weights recorded at different time points (117.6
5.678 for day 1, 114.2
5.209 for day 7, 108.5
5.752 for day 14 and 109.3
0.976 for day 21). It can be concluded that the higher concentration of CMC lead to higher degradation rate. Figure 3 shows that Alg3%–CMC3% and Alg4%–CMC2% scaffolds maintained their shape in PBS until day 21; however, Alg2%–CMC4% scaffolds were partially degraded and lost their shape to some extent.
To investigate the effect of Alg and CMC concentrations on mechanical performance of the 3D printed scaffolds, uniaxial mechanical test was performed. The stress–strain curves shown in figure 3 indicated that the compressive stress enhanced as the content of the Alg to CMC in the ink increased. In addition, the Alg2%–CMC4% scaffolds showed the lowest Young's modulus of 6.320 1.339 kPa (figure 3). By increasing the concentration of Alg and decreasing the concentration of CMC, the Young's modulus of the scaffolds significantly increased to 26.02
3.663 kPa for Alg3%–CMC3% (P < 0.001) and 57.08
6.162 kPa for Alg4%–CMC2% (P < 0.001). Thus, it was observed that Alg4%–CMC2% (57.08
6.162 kPa) exhibited the highest elastic modulus among all three groups with 2.2- and 9-fold increase as compared to Alg3%–CMC3% (26.02
3.663 kPa) and Alg2%–CMC4% (6.320
1.339 kPa), respectively.
3.5. Assessment of viability of HAT-7 cells in the 3D printed hydrogels using live/dead assay
To investigate the compatibility of cells with hydrogel compositions and printing process, live/dead staining was carried out after 1, 7 and 14 d of in vitro culture. The viability of HAT-7 cells bioprinted in the Alg-CMC hydrogel scaffolds and representative live/dead images are shown in figure 4. HAT-7 cells cultured in all three groups of scaffolds revealed a high cell viability (>80%) at all time points. High percentage of cell viability in all groups of bioinks on day 1 suggests the negligible cytotoxic impact of printing process. In addition, viability of cells increased over time which can be due to the proliferation of cells in 3D printed scaffolds.
Figure 4. Fluorescent images of HAT-7 cells in 3D printed scaffolds with varying concentrations of alginate and carboxymethyl chitosan (Alg2%–CMC4%, Alg3%–CMC3%, Alg4%–CMC2%) after 1 (a), 7 (b) and 14 (c) days of in vitro culture examined by LIVE/DEAD Cell imaging kit with Calcein AM for staining live cells and BOBO-3 for staining dead cells (image's magnification is 100 µm). (d) The quantitative analysis of cell viability in printed constructs at different time points. HAT-7 cells maintained a percentage of cell viability at all timepoints on days 1, 7 and 14. Error bars represent the mean ± SD of three experimental replicates. (* in the graphs indicates the statistical significance between three different groups of scaffolds, ***p < 0.001, *p < 0.05).
Download figure:
Standard image High-resolution image3.6. Evaluation of the ALP and ameloblast gene expression as markers of ameloblast differentiation and Ca and P detection by SEM-EDX as markers of ameloblast mineralization
To evaluate the capacity of HAT-7 cells for mineralization, ameloblast differentiation and enamel formation when cultured in 3D printed Alg-CMC constructs, ALP staining was performed. As shown in figure 5, a strong expression of ALP was detected in HAT-7 cell-laden constructs (Alg4%–CMC2%) when cultured for 14 d.
Figure 5. Alkaline phosphatase staining of HAT-7 cells in 3D printed alginate4%-carboxymethyl chitosan2% scaffolds after 14 d of culture detected using SIGMAFASTTM BCIP®/NBT detection kit. (a-1) and (a-2) shows the macroscopic images of cell laden scaffolds before and after staining, respectively. (b) shows the microscopic image of the cell laden constructs.
Download figure:
Standard image High-resolution imageTo evaluate the capacity of HAT-7 cells for ameloblast differentiation, mineralization, and enamel formation when cultured in 3D printed Alg-CMC constructs, qRT-PCR and SEM-EDX were performed and compared to ALP staining. As shown in figure 6(a), the expression of key ameloblast differentiation markers, such as DSPP, AMEL, ENAM, KLK4, ORAI1, and ALP, determined by qRT-PCR, suggests the beginning of HAT-7 cell differentiation to ameloblasts. Interestingly, the expression of some differentiation markers is statistically significant in 4%Alg–2%CMC 3D-scaffolds cultured for two days compared to other types of scaffolds. Moreover, an intense ALP staining reflected in figure 6(c) was also detected in HAT-7 cell-laden constructs (Alg4%–CMC2%) cultured for 14 d. In addition, a higher mineralized trend (calcium and phosphorus content %) evaluated by SEM-EDX was observed in cells incorporated in Alg4%–CMC2% and 2%Alg–4%CMC 3D scaffolds cultured for 14 d, indicating that these scaffolds favor the progression of differentiation of HAT-7 cells to mineralization stage (figures 6(b) and (d)).
Figure 6. Evaluation of HAT-7 cell differentiation to ameloblasts. (a) Relative gene expression of ameloblast differentiation markers in cells incorporated in scaffolds cultured for two days. Error bars represent the mean ± SD of three experimental replicates. (* in the graphs indicates the statistical significance between three different groups of scaffolds, **—P < 0.01, ***—P < 0.001, ****—P < 0.0001, assessed by using a t-test). Statistical analysis is done using a t-test that compares the means between two biological duplicates. (b) Mineralization of HAT-7 cells incorporated in scaffolds (Calcium content %). (c) Alkaline phosphatase staining of HAT-7 cells contained in the scaffolds. (d) Mineralization of HAT-7 cells incorporated in the scaffolds (Phosphorus content %).
Download figure:
Standard image High-resolution image3.7. Observation of cell morphology and mineral deposition under SEM
After 14 d of culture, cell morphology and mineral deposition in 3D printed scaffolds were assessed by SEM. As shown in figure 7, HAT-7 cells exhibited their normal round morphology in 3D printed scaffolds at all three different concentrations of Alg and CMC. In addition, the amorphous globular structures were observed on the cell surface, indicating the initiation of mineralization and ameloblast differentiation.
Figure 7. Scanning electron microscopy (SEM) images of HAT-7 cells in the 3D printed scaffolds after 14 d of in vitro culture done in duplicates. Representative scaffold structure with incorporated HAT-7 cells observed under magnification of 1.00 mm (1), 100 μm (2), and 50.00 μm (3). Cell morphology and mineral deposition is observed (4). Blue and yellow arrows indicate various amorphous globular structures observed on the surface of cells cultured for 14 d (magnification is 5 μm), indicating the initiation of mineralization and ameloblast differentiation. SEM images of the scaffold surfaces without cells (magnification is 5.00 μm) (5). Blue and orange arrows show the globular- and ribbon-like structures on the cell surface.
Download figure:
Standard image High-resolution image4. Discussion
Loss of dental tissues or the whole tooth caused by factors such as tooth decay, trauma, genetic and periodontal diseases is a major dental health care issue that has serious physiological and psychological impacts on patients [66]. Regeneration of dental tissues with aesthetic and functional properties close to native tissues that can provide a permanent solution for tooth damage or loss is the ultimate goal of the regenerative dentistry. Tissue engineering could offer such promising solution to regenerate damaged dental tissues including enamel [1]. Scaffolds are one of the main elements for tissue engineering that need to possess desirable physical (swelling, degradation, mechanical) and biological and functional characteristics to support the new tissue formation [67].
Extrusion based 3D bioprinting has been broadly used to create 3D tissue constructs that allows spatially controlled patterning of biomaterials and cells [68]. One of the essential factors for the successful tissue regeneration is the selection of the scaffold materials. In this study, we used Alg-CMC as a hydrogel bioink for 3D extrusion bioprinting to combine the good printability of Alg with antibacterial and biological properties of CMC that have been shown to be desirable for dental tissue applications [34, 69]. Another benefit of the binary blend of Alg-CMC as the bioink is the formation of dual crosslinked hydrogels. In fact, both polymers can form hydrogel via physical crosslinking in the presence of calcium ions. In addition, the amino group of the CMC is able to form ionic bonds with carboxyl group of Alg [53, 70]. The interpenetrating polymer network is formed in the dual crosslinked Alg-CMC hydrogels due to the entanglement of two polymer networks leading to the retention of both hydrogel components in the bioink [71]. The mixture ratio of these two components in the bioink was altered to find the suitable concentration in terms of printability, degradation, swelling, mechanical and biological properties. Different mixture ratios of the ink required different amount of pressure to overcome the surface tension of the hydrogel solution owing to their varying viscosities. The hydrogel solutions with higher viscosity was found to provide better extrusion uniformity and allows the printed structures to retain their shape and have higher mechanical stability, which is more favorable for printing tall and complex structures; nevertheless, they can cause increase in shear stress that can negatively influence cell survival and functions [72, 73]. On the other hand, hydrogel solutions with low viscosity can cause low printability, non-uniform cell distribution and fast cell settling [73].
Among three different groups of hydrogel solutions assessed in this study, the one with the lowest concentration of Alg (Alg2%–CMC4%) showed the lowest viscosity and thereby needed lowest extrusion pressure. Alg2%–CMC4% group also showed a better strand printability, smaller strand size and larger pore size which it might be due to the fact that all groups of hydrogel solutions were printed with the same speed. The speed could be adjusted for each solution to prevent extra deposition of the hydrogel and have the strands with the same range of strand diameter, pore size and strand printability. However, all groups of hydrogel solutions showed the acceptable pore printability. As discussed previously, to find the proper printing parameters, both strand and pore printability, and not only one of them, are required to be considered [63].
Another important parameter that needs to be taken into consideration when fabricating complex constructs using 3D printing is the ability to print multiple layers [51]. In our study, the Alg-CMC hydrogels with all three mixture ratios showed the ability to be printed as multilayered structures with relatively straight strands and adequate porous structures. The SEM images of 3D printed scaffolds as well as synchrotron-based x-ray imaging confirmed the porous structure of our scaffolds, suggesting that these pores may allow easy exchange of gases and nutrients, proteins, and metabolites between the incorporated cell population and the surrounding culture medium. Our microstructural observation of the scaffolds is in agreement with another study showing that the addition of CMC to Alg /gelatin makes constructs with better well-defined pores [53].
In addition, the 3D structure of the full thickness of the scaffolds visualized by synchrotron-based x-ray imaging showed the structural stability and integrity of the Alg-CMC scaffolds; scaffolds with higher concentration of CMC (Alg2%–CMC4% and Alg3%–CMC3%) showed smaller strand size and larger pore size with square shaped pores as compared with Alg4%–CMC2% scaffolds.
Swelling behavior, degradation rate and mechanical properties are the essential physical characteristics of the scaffolds that are important for successful tissue regeneration. Hydrogel solutions with higher concentration of CMC showed the higher SR, after 1 d incubation in PBS, and a faster degradation rate and lower mechanical properties. Swelling behavior of the hydrogels is a detrimental parameter for the exchange of nutrients and metabolic wastes in the hydrogel matrix [74]. The increased SR by increasing the amount of CMC in the hydrogel that was observed in this study could be attributed to the lower crosslinking density, as a result, the mobility of the polymer chains is less restricted leading to a higher level of water retention [75]. Hydrogel solutions with a higher concentration of CMC showed a higher SR after one-day incubation in PBS and a faster degradation rate, and lower mechanical properties. In addition, the presence of amino groups in the constructs with higher concentration of CMC could increase the swelling capacity of the hydrogel [76]. Degradation rate of the scaffolds needs to be long enough to provide supports for cell growth and extracellular matrix secretion and then the hydrogels need to be gradually degraded after tissue formation [53, 77]. It was also previously reported that the degradation rate of the Alg-CM gels is contingent upon the SR in a fashion that gels with higher SR forms a polymer network that is less compact with ionic crosslinking and have a faster degradation rate [78]. In addition, CMC was found to have an very high degradation rate which can cause faster degradation of the hydrogel scaffolds with higher amount of CMC [32]. In addition, CMC was found to have a very high degradation rate which can cause more rapid degradation of the hydrogel scaffolds with a higher amount of CMC [32]. Overall, the SR results along with the cell viability assay in our study confirm that the bioprinted hydrogens facilitate nutrients' and metabolites' exchange between the medium and incorporated cells.
Mechanical properties of hydrogels is another important parameter that allows the scaffold to maintain their shape and greatly influences tissue regeneration and cell behaviors such as viability, spreading, proliferation, and differentiation [21, 53, 79]. Mechanical properties of the hydrogels depend on the crosslinking density, the type of crosslinking mechanism, the nature of the polymer [80]. Additionally, strand diameter and porosity of 3D printed scaffolds affect mechanical properties in a way that scaffolds with thicker strands have lower porosity and higher mechanical properties [6]. In this study, the mechanical properties of scaffolds with higher amount of CMC (Alg2%–CMC4%) was significantly lower as compared to two other groups, which can be due to the inherently poor mechanical properties of pure CMC [81], a lower degree of crosslinking, less tight polymeric network, and smaller strand size and higher porosity. In addition to the structural integrity and mechanical stability, 3D bioprinted scaffolds should be able to support printed cells to maintain their normal morphology, viability, and functionality (including cell attachment, proliferation, differentiation, and matrix production) [82, 83].
The results of our live/dead assay showed that all groups of 3D bioprinted scaffolds could support the viability and possibly proliferation of HAT-7 cells with minor cytotoxic effect from printing process. Other studies indicated that the nature of the polymer, polymer concentration, the type of crosslinker, crosslinking time, crosslinker concentration, cell type and density, and shear stress during the printing process depend on several factors, such as needle size and extrusion pressure and proper printability, that significantly impact cell survival/damage [6, 40, 84, 85]. In this study, the type and concentration of crosslinker, crosslinking time, cell type and density, needle size were kept constant. Although the bioprinted scaffolds with higher concentration of Alg (Alg3%-CMC3% and Alg4%-CMC2%) needed a higher extrusion pressure to be extruded, these two groups of scaffolds did not show a lower cell viability as compared to Alg2%–CMC4%. Previous studies have reported the use of multi-component bioinks containing Alg and CMC for bioprinting of different cell types including human neural stem cells [54], bone marrow mesenchymal stem cells [53, 57], and pluripotent stem cells [58], human osteogenic sarcoma cells [55]. Another study showed that Alg-CMC hydrogel scaffolds that were fabricated by Ca2+ crosslinking, without being 3D printed, and then seeded with cells could support cell growth and proliferation for neural tissue engineering applications [49]. However, to the best of our knowledge no study investigated the potential of the Alg-CMC-based hydrogels for fabrication of 3D bioprinted dental stem cells-laden constructs. In our study, SEM images revealed that Alg-CMC bioink could provide a favorable environment for HAT-7 cells to retain their round-shaped round morphology and promote their attachment to the scaffolds and be guided towards mineralization and differentiation, which are essential for the enamel-like tissue formation. This is in agreement with a previous study reporting the round morphology of the HAT-7 cells when co-cultured with dental pulp stem cells in biphasic scaffolds composed of chitosan-collagen I hydrogels where their viability and proliferation were supported [4]. ALP expression, as a marker of ameloblast differentiation and mineralization, that was detected via ALP staining of bioprinted HAT-7 cells further confirmed the mineralization potential of Alg-CMC bioink.
In our study, the expression of ameloblast differentiation markers along with ALP expression, as a marker of ameloblast differentiation and the detection of Ca and P as marks of matrix mineralization, further confirmed the suitability of these scaffolds for differentiation of the dental epithelial cells to ameloblasts producing enamel. Moreover, our results suggest that Alg4%–CMC2% scaffolds favor the progression of differentiation of HAT-7 cells to the mineralization stage, highlighting the importance of the mechanical properties of these scaffolds to promote dental epithelial cells to become ameloblasts. Our results also demonstrated that Alg4%–CMC2% exhibited the highest elastic modulus among all three groups, which means that they have the highest stiffness. In general, soft matrices direct differentiation towards neuronal tissue, while stiff matrices promote it towards bone tissue [85, 86], explaining the ability of Alg4%–CMC2% scaffolds to promote the enamel cell differentiation.
5. Conclusion
In this study, a system composed of dental epithelial stem cells incorporated into bioink composed of Alg and CMC was characterized for potential use in 3D bioprinting and dental tissue regeneration applications with a focus on potential enamel regeneration approaches. Collectively, our findings showed that Alg-CMC blend hydrogels have good printability and suitable swelling ability, adequate degradation rate and mechanical properties, and were able to provide a favorable microenvironment for the dental epithelial stem cells, HAT-7, to maintain their viability and to differentiate towards an ameloblast like genotype resulting in enamel-like mineralized matrix. The highly porous structure of the scaffolds could be an indicator of their ability to facilitate diffusion of nutrients and wastes. Our findings further support the use of Alg and CMC bioinks for tissue regeneration as previously shown in other tissues including bone tissue engineering [86, 87]. Future studies, similar to dentin regeneration reports published by our group and others [88–90], using in vitro culture of cell-laden scaffolds in differentiation media accompanied by in vivo studies using pre-clinical enamel regeneration models could further validate the suitability of the Alg-CMC bioinks for dental enamel tissue regeneration. Taken together, our findings suggest that Alg-CMC is a promising candidate to be used as bioink for enamel tissue engineering.
Acknowledgments
We would like to acknowledge the support from the Saskatchewan Health Research Foundation (SHRF), NSERC Discovery grant, the University of Saskatchewan Centennial Enhancement Chair, the University of Saskatchewan Global Community Service Fund and the College of Dentistry start-up funds (PI: PP); College of Medicine start-up funds (SP); Natural Science and Engineering Research Council (NSERC) of Canada (XC), and the University of Saskatchewan Dean's Scholarship and Biomedical Engineering Devolved Scholarship (to FM). Part of the research described in this paper was performed at the CLS, a national research facility of the University of Saskatchewan, which is supported by the Canada Foundation for Innovation (CFI), the Natural Sciences and Engineering Research Council (NSERC), the National Research Council (NRC), the Canadian Institutes of Health Research (CIHR), the Government of Saskatchewan, and the University of Saskatchewan. Authors would like to thank Adam Web, Adam McInnes, and Ibrahim Hoja for their help with synchrotron imaging.
Data availability statement
All data that support the findings of this study are included within the article (and any supplementary files).
Conflict of interest
The authors declare no competing financial interests.
Supplementary data (0.3 MB DOCX)