Abstract
A 28 GHz system with a high-power gyrotron tube has been used for the QUEST spherical tokamak to form an over-dense plasma for electron Bernstein wave (EBW) heating and current drive with an 8.2 GHz-wave. Non-inductive high-density plasma ramp-up experiments with dual-frequency (dual-f ) electron cyclotron (EC) (8.2 GHz and 28 GHz) waves were conducted. A spontaneous density jump (SDJ) to an over-dense state was first observed as a bifurcation phenomenon in the dual-f wave experiment. The over-dense plasma on the 8.2 GHz wave was non-inductively ramped up to 25 kA, and was maintained for 0.4 s under stable plasma equilibrium after two such jumps in one shot. Heating to mildly energetic electrons and bulk electrons was observed even in the over-dense region. The electrostatic EBW heating effect on the mildly energetic electrons in the over-dense region is assessed following a dispersion analysis of the 8.2 GHz wave. The bulk electron heating effect observed is explained as heat exchange from mildly energetic electrons heated by the electrostatic EBW. Remarkably, a high hard-x-ray-radiation temperature of ∼500 keV was also observed in tangential viewing for current-carrying electrons in the over-dense core region. Synergetic heating from the overlap of different 28 GHz EC harmonic resonances as well as higher harmonic heating is discussed for maintaining the highly energetic electrons in the over-dense core region. In addition, the SDJ process and mechanism are considered based on the discussion of the electron heating effects with the 8.2 GHz wave.
Export citation and abstract BibTeX RIS
1. Introduction
The Q-shu University experiment with steady state spherical tokamak (QUEST) project was proposed at Kyushu University to achieve and investigate steady-state operation in the spherical tokamak (ST) configuration under controlled plasma–wall interactions [1]. In STs, the center solenoid (CS) fluxes for ohmic tokamak discharges are inherently limited because the center-stack area is very small. Advanced tokamak reactor designs with either zero or small CS fluxes have been proposed [2, 3]. Non-inductive plasma ramp-up experiments using electron cyclotron waves (ECWs) have been conducted to supplement either zero or limited CS fluxes in many STs [4–7] as well as tokamaks [8, 9]. Electron cyclotron heating and current drive (ECHCD) and electron Bernstein wave heating and current drive (EBWHCD) with an 8.2 GHz wave have been explored for non-inductive plasma ramp-up and steady-state plasma sustainment in the QUEST. In low-field side injection, electrostatic electron Bernstein waves (EBWs) have to be excited through mode conversions from the electromagnetic ECWs of ordinary (O) and slow extra-ordinary (X) modes in over-dense plasmas beyond the cut-off density. The O–X–B mode conversion scenario of the low-field side injection has been considered for EBWHCD experiments with the 8.2 GHz wave in the QUEST [10]. Recently, X–B mode conversion experiments of high-field injection have begun in the QUEST [11].
In collaboration between the National Institute for Fusion Science, University of Tsukuba and Kyushu University, a higher-frequency ECHCD system with a 28 GHz gyrotron tube has been developed to attain high-density plasmas and to ramp up high-current plasmas non-inductively [7, 12]. Although significant single-path absorption of the excited horizontal or O-mode wave was not expected in the second (2nd) harmonic electron cyclotron (EC) plasma ramp-up scenario, a high of 54 kA was attained and was stably sustained for 0.9 s with the simplest possible transmission and launcher systems [12]. A higher
of 66 kA was achieved with a 270 kW 28 GHz wave with a slow ramp-up of the vertical field. A clear ECHCD effect during recharging was also observed in the 28 GHz wave injection into the ohmically heated plasma maintained through feedback regulation of the CS coil current.
Dual-frequency (dual-f ) experiments with 8.2 GHz and 28 GHz waves have been conducted to explore high-current and high-density plasma sustainment even in the over-dense state with the 8.2 GHz wave. A 40 kW 8.2 GHz wave was injected into target plasma generated with a 270 kW 28 GHz wave at constant vertical field . The target current
kA decreased noticeably in connection with plasma shrinking due to the 8.2 GHz ECHCD effect, and then a small-sized plasma became stable at low current level ∼10 kA. Because the target plasma significantly expanded beyond the 8.2 GHz second harmonic electron cyclotron resonance (ECR) position
, the effect of the second harmonic ECHCD on increasing electron pressure also had to be taken into account for the observed increment of the Shafranov shift. If
decreases with changes in plasma shaping, the plasma shifts inward as the field
becomes too strong. The plasma also becomes small because of the shift in the inboard limiter configuration, and then
decreases more, resulting in a feedback loop for the decreasing
. The plasma shaping parameters, such as the minor radius a and the outermost major radius
of the plasma shrinking and further intersecting the inboard limiter, and the magnetic axis position
of the Shafranov shift, were evaluated from the magnetic surface structures reconstructed by the filament current method using 64 flux signals [13].
To maintain the target with the superposed 8.2 GHz wave, a relatively strong
was applied when superposition occurred [14]. With a strong
, the force balance during equilibrium was satisfied even with the increased electron pressure due to the ECHCD effect. Furthermore, some localized EC interactions near peripheral
can be avoided as
becomes smaller than
relatively early. A 40 kA plasma was ramped with the 270 kW 28 GHz wave, and then a 60 kW 8.2 GHz wave was injected into the 28 GHz target plasma. By increasing
, the minor radius a decreased with the constant
. A significant current-driving loop voltage was observed in the
ramp-up phase, but the 40 kA current level was later non-inductively maintained. The density began to increase spontaneously under the stable configuration. The line-averaged density
almost reached the O-mode cutoff density
of 8.35
m−3, but
did not exceed the cutoff.
The plasma of kA was not properly sustained because of the plasma shrinking in the superposition of 8.2 GHz wave to the 28 GHz target plasma at constant
. The density achieved was not beyond
on the 8.2 GHz wave even when the plasma of
kA was sustained with the
ramp-up. After such challenges to attain an over-dense plasma with the 8.2 GHz wave, over-dense plasma was achieved through spontaneous density-jump (SDJ) phenomena in simultaneous dual-f injections. The plasma shaping was self-organized to be more stable in the simultaneous dual-f injections. The SDJ to the over-dense state was observed as a bifurcation phenomenon. A 25 kA over-dense plasma was maintained for 0.4 s with the dual-f waves. Energetic electron and bulk electron heating effects were observed in the over-dense region. The results of the dual-f experiments of over-dense plasma through SDJ are reported in this paper, with an emphasis on discussing electron heating effects in the over-dense region.
This paper is organized as follows. Section 2 introduces the experimental setup of the dual-f experiments in the QUEST. In section 3, non-inductive current ramp-up experimental results using dual-f ECWs are described. Energetic and bulk electron heating effects observed in the over-dense plasma under a stable equilibrium are discussed in section 4 through resonance condition and dispersion analyses. The SDJ process and mechanism are also discussed in this section. Conclusions are given in section 5.
2. Experimental apparatuses
The QUEST device is a medium-sized ST with nominal major and minor radii, R0 = 0.64 m and a = 0.42 m, respectively. A poloidal cross-section of the QUEST device (figure 1) shows four pairs of poloidal magnetic field coils to create field lines of various curvatures characterized by the n-index or magnetic mirror ratio M along the open field lines between starting points (the mid-plane) and terminating points (the flat diverter plates). The 8.2 GHz fundamental and the 28 GHz second harmonic 2
ECR locations are at the major radii of
= 0.55 m and
= 0.32 m, respectively. The 2
location is at
= 1.1 m, and within the outer plasma boundary if the large-sized plasma is formed, for instance, with the 28 GHz wave, whereas the inboard
location of
is outside the vacuum vessel boundary of
= 0.22 m.
Figure 1. Poloidal cross-section of the QUEST device. Fundamental and second harmonic electron cyclotron resonance positions are presented as and
for 8.2 GHz, and
for 28 GHz. Four pairs of poloidal magnetic field coils (PFCs) generate various field-line curvatures characterized by the n-index or magnetic mirror ratio M.
Download figure:
Standard image High-resolution imageIn the O–X–B mode conversion experiments, the incident mode and angle need to be well controlled to attain high mode-conversion efficiency. Phased-array antennas (PAAs) of the multiple waveguide-element launcher, which enable us to control the incident mode and angle, were proposed for the 8.2 GHz systems with 8 klystrons for EBWHCD experiments in the QUEST. We developed 8.2 GHz CW-PAA systems with 16 waveguide (WG) lines to focus and steer the beams and to control the incident mode; their performance was assessed on a low-power test bench [15, 16], and in the QUEST vacuum vessel at a high power level [7]. The O–X–B EBWHCD scenario was investigated in a multiple-ray-tracing simulation with ∼1000 rays using TASK/WR code [17] with implementation of the mode conversion process [18]. The PAA system was used for the dual-f experiments, but proper PAA performance was not expected on this occasion, because a number of operating antenna elements were lacking. Only six out of 16 waveguide elements were operating at the time. Because of the limited number of operating antenna elements, the antennae worked as a multiple waveguide-element launcher without proper phased-array setup for all experiments presented in this study.
A 28 GHz gyrotron with a cavity and a triode magnetron injection gun was developed at the University of Tsukuba [19]. A 1 MW maximum output power was obtained, and a high 40% oscillating efficiency was also demonstrated at 0.8 MW output without collector potential depression (CPD). For the QUEST experiments, the operational envelope of the 28 GHz gyrotron was evaluated and assessed. A 0.4 MW power capability without CPD was confirmed [10]. The output power from the 28 GHz gyrotron was supplied to the QUEST by the simplest possible transmission line, composed of circular-corrugated 63.5 mm diameter WGs following a miter bend. One WG was inserted at the mid-plane of the QUEST device as a launcher through a sapphire single-disk window with electrical isolation to the WG line. A new 28 GHz transmission line with a focusing launcher and corrugated-plate polarizers [20, 21] has been recently developed to study the local heating effect on EC plasma ramp-up and maintenance, but in the present dual-f experiments, the simplest possible 28 GHz system in [12] was used.
Note that multiple-wall effects with ray and mode scrambling should be dominant during heating because beam focusing and steering, as well as the incident mode, were not properly controlled for either of the dual-f injections. The ray-tracing approach was not suitable for consideration of the heating and mode conversion processes under the ray-scrambling situation with multiple wall reflections.
3. Experimental results
The SDJ across was found as a bifurcation phenomenon in the dual-f injections. Figure 2 shows the time evolution of plasma current
,
, H
intensity,
, minor radius a, and
(sum of average poloidal beta
and half plasma internal inductance
) with and without the SDJ.
was evaluated from the generalized Shafranov equation for the radial force balance in equilibrium with
[22].

Figure 2. Time evolution of: (a) plasma current , (b) line-averaged density
, (c) H
intensity, (d): magnetic axis position
and minor radius a, (e)
(sum of average poloidal beta
and half plasma internal inductance
) with and without spontaneous density jump (SDJ). Dual-frequency (dual-f ) (8.2 GHz and 28 GHz) waves are simultaneously injected to maintain the plasma. Adapted courtesy of IAEA. Figure adapted from [14]. Copyright 2014 IAEA.
Download figure:
Standard image High-resolution imageRelatively strong gas fueling was applied at t = 2.9 s, and the line-averaged density reached
for one brief moment at
3.0 s, but the density could not be maintained and later decreased gradually. The operating parameters including gas fueling were identical in both discharges. In the SDJ case, the H
intensity started to become constant, and
and a began to decrease and became constant.
initially decreased but then recovered. A relatively high
was obtained. The plasma was self-organized to be more stable with different plasma shaping, and then
was recovered in the high-
and high-density plasma.
Moreover, the over-dense plasma was non-inductively ramped up to ∼25 kA and was stably sustained for 0.4 s as shown in figure 3 after SDJs occurred twice with the dual-f wave injections. Figure 3 shows the time evolution of differential pressure at a fueling hydrogen reservoir,
, H
intensity, a,
,
,
, and total amount of hard x-ray (HX) counts in the energy range of 50–250 keV measured by a CdTe detector [24]. The 270 kW 28 GHz wave was injected into pre-ionized plasmas with the 50 kW 8.2 GHz wave. The plasma current
was rapidly ramped with the 28 GHz wave at t = 1.6 s at constant
. The 8.2 GHz wave was simultaneously injected with the 28 GHz wave even after the
ramp-up. Gas-fueling timings were marked with
increments (at
and
). The gas injector was located at the inboard center stack. The H
intensity increased at the fueling times, whereas the density
did not always increase at these moments. The density
increased spontaneously twice (at
and
) in the shot, and finally exceeded
. The minor radius a became larger with the
ramp-up until the 1st SDJ. It seemed that the outermost radius
1.2 m was clamped at around
= 1.1 m after the 1st SDJ, and then gradually decreased after the second SDJ together with
. Proper plasma shaping or equilibrium was successfully obtained in the simultaneous dual-f injected experiment from the beginning of
ramp-up. The
value was relatively large (>1.5), and roughly changed inversely proportionally to the
evolution. The HXs were measured at forward (FWD) and backward (BWD) tangential viewings for current-carrying energetic electrons at the mid-plane. The measured HX intensity of the FWD viewing was several times larger than the BWD intensity. The HX intensity rapidly increased in the low-density phase until
, and dropped again at
after bouncing back at
.
Figure 3. Time evolution of: (a) differential pressure at a fueling hydrogen reservoir,
and H
intensity, and (b) a,
,
,
, and the total amount of hard x-ray (HX) counts in the energy range of 50–250 keV measured by a CdTe detector. The HX count measured in forward (FWD) viewing for current-carrying electrons at a tangential radius of
= 0.71 m is shown after averaging in 2 shots. The 270 kW 28 GHz wave is injected into the pre-ionized plasma with the 50 kW 8.2 GHz wave at t = 1.6 s. The plasma current of
is rapidly ramped to ∼25 kA with the 28 GHz wave. The dual-f waves are simultaneously injected to maintain the plasma. Gas-fueling timings are marked with
increments (
and
). Reproduced courtesy of IAEA. Figure adapted from [23]. Copyright 2016 IAEA.
Download figure:
Standard image High-resolution imageFigure 4 shows the HX energy spectra at the tangential radii of = 0.71 m and 0.34 m in the over-dense plasma after the second SDJ. The tangential viewing of
= 0.34 m also included the HX emission in the inner region near
= 0.32 m, whereas that of
= 0.71 m only crossed the core region around
m and the outer region. The core region around
m was over-dense after the second SDJ as shown in figure 7 later. To obtain the HX energy spectra with an improved signal-to-noise ratio, the HX signals were accumulated for 0.4 s after the second SDJ in two shots of the FWD viewings at
0.34 m and 0.71 m respectively, out of the shots repeated for the Thomson scattering (TS) measurements mentioned below. The HX radiation temperature
was (507
35) keV at
= 0.71 m but was (231
10) keV at
= 0.34 m.
was notably about 50 keV in the low-density plasma ramped non-inductively with solely the 28 GHz wave. The HX temperature in the dual-f experiment was remarkably high, in particular at the core region. The possible heating and maintenance mechanisms are discussed later in section 4.3.
Figure 4. Hard-x-ray (HX) energy spectra at = 0.71 m and 0.34 m after the second SDJ in the 25 kA plasma with dual-f waves. The tangential viewing of
= 0.34 m includes HX emission near
= 0.32 m, whereas that of
= 0.71 m only crosses the core region around
∼ 0.8 m and the outer region. The HXs are measured at FWD viewings for current-carrying energetic electrons at the mid-plane. Reproduced courtesy of IAEA. Figure adapted from [23]. Copyright 2016 IAEA.
Download figure:
Standard image High-resolution imageFigure 5 shows the time evolution of OII line intensity, visible light intensity at a mid-plane chord measured by a fast camera, and an absolute extreme ultraviolet (AXUV) bolometer signal in the shot. The visible light intensity increased at fueling timings similar to the H intensity evolution. The OII line intensity did not respond to the fueling, and did not follow the other evolution. The AXUV signal started to increase rapidly at
and was extremely enhanced after t =
. Figure 6 shows the time evolution of the AXUV fan-arrayed intensity profile at a tangential viewing of
= 0.50 m from an elevated position of Z = 0.77 m for the mid-plane. The impact parameter p was defined as the profile coordinate of the orthogonal distance to the chords from
=
[12]. A few chords with
and all the chords with p < 0 cross the mid-plane (Z = 0). The notable increment in the AXUV signal with significant sensitivity in the soft x-ray (SX) energy range indicates a pressure increase of the bulk and/or mildly energetic electrons around
after t =
. The extremely enhanced signal evolution showed effective heating of the bulk and/or mildly energetic electrons.
Figure 5. Time evolution of OII line intensity, visible light intensity measured by a fast camera at the mid-plane, and absolute extreme ultraviolet (AXUV) bolometer signal in the 25 kA over-dense plasma by the dual-f waves.
Download figure:
Standard image High-resolution imageFigure 6. Time evolution of the AXUV fan-arrayed profile at a tangential viewing with its radius = 0.50 m from an elevated position of Z = 0.77 m for the mid-plane in the 25 kA over-dense plasma. The impact parameter p is defined as the profile coordinate of orthogonal distance to the chords from
.
Download figure:
Standard image High-resolution imageFigure 7 shows the radial profiles of the electron temperature , electron density
, and electron pressure
measured by the TS diagnostics at t = 1.85 s, 2.25 s, and 2.55 s before and after the second SDJ in the 25 kA over-dense plasma. Relatively high
eV was observed at a major radius R = 0.64 m near
only at a low-density TS timing of t = 1.75 s. As shown in the figure,
becomes over-dense and
drops at this moment because of the cutoff at t = 1.85 s. At t = 2.25 s and 2.55 s after the second SDJ,
has become over-dense over almost the entire region, and
increases even in the over-dense region at R = 0.93 m. A considerable increase in
was observed in the over-dense region after the second SDJ in concert with a significant rise in the AXUV signals. In the peripheral region of R = 1.08 m, which is close to
of 1.1 m, significant
values were observed at t = 1.85 s and 2.25 s. The pressure profiles were hollow-like, and the outer peaks corresponding to the inner peaks near
may be located around
m, at an intermediate position between the TS measuring positions of R = 0.93 m and 1.08 m. Equilibrium plasma shaping of the 25 kA over-dense plasma is discussed later in section 4.1. As far as
is concerned in regard to the pressure profiles,
was around
m before the second SDJ, and then appeared to shift inward a little after the second SDJ together with
; see figure 3. The peripheral
at R = 1.08 m became smaller after the second SDJ because of a shift in
and
. Radiation cooling in the core region around
, where
was over-dense and
50 eV, may contribute to the formation of the hollow-like pressure profiles. Electron heating effects in the over-dense region are discussed in section 4.
Figure 7. Radial profiles of the electron temperature , electron density
, and electron pressure
, measured by Thomson scattering diagnostics, in the 25 kA over-dense plasma by the dual-f waves. Reproduced courtesy of IAEA. Figure adapted from [23]. Copyright 2016 IAEA.
Download figure:
Standard image High-resolution image4. Discussion
The force balance at equilibrium and stable plasma shaping should be properly considered when increasing the electron pressure with dual-f waves. The self-organized plasma equilibrium or shaping was attained in the over-dense plasma through simultaneous injections of the 8.2 GHz and 28 GHz waves. Energetic and bulk electron heating effects were observed in the over-dense region. In this section, both these effects in the over-dense region are discussed in light of the plasma equilibrium analyses obtained with EFIT code [25].
4.1. Plasma equilibrium analysis for over-dense plasma through SDJs
The equilibrium of the 25 kA over-dense plasma obtained through SDJs in the dual-f injections was analyzed using EFIT code. Figure 8 shows the equilibrium magnetic surface structures of the 25 kA over-dense plasma before and after the second SDJ (at t = 1.85 s and 2.25 s). Large Shafranov shifts were seen at both timings; and
decreased after the second SDJ, in agreement with the filament current analysis and the TS measurement result. Dense flux surface structures appeared between
and
. The self-organized plasma shaping with small elongation
was quite different from the shaping of the plasma ramped up with solely the 28 GHz wave [12]. The poloidal beta
in the plasma using the dual-f waves (∼1.5) was larger than for the 28 GHz plasma (∼0.8). The self-organized plasma shaping has been discussed in regard to high
plasmas [26].
Figure 8. Equilibrium magnetic surface structures of the 25 kA over-dense plasma before and after the second SDJ (at t = 1.85 s and 2.25 s) analyzed with EFIT code. Reproduced courtesy of IAEA. Figure adapted from [23]. Copyright 2016 IAEA.
Download figure:
Standard image High-resolution imageThe plasma or electron pressure required for the equilibrium was larger than 400 Pa near the axis and, being a function of nested flux surfaces, its profile was bell-shaped. In contrast, the bulk pressure measured by the TS diagnostics was ∼10 Pa and its profile was hollow-like. In the EFIT analysis, the pressure and current profiles of low-order polynomials were used with the external magnetics for constraints; the distribution profiles therefore cannot be discussed in detail.
4.2. 8.2.GHz heating effect on bulk and/or mildly energetic electrons in the over-dense region
Electron heating effects with the 8.2 GHz wave on energetic and bulk electrons after the second SDJ are discussed next. Figure 9 shows the radial profiles of electron cyclotron frequency , plasma frequency
, and upper hybrid resonance (UHR) frequency
on the 25 kA over-dense plasma at t = 2.55 s. An 8.2 GHz wave reached the O-mode cutoff layer
m where f =
, and the O-mode wave converted into the X-mode. The wave propagated to the upper hybrid resonance layer of
m after the O–X mode conversion, and then the X-mode converted into EBW at
. The electrostatic EBW can propagate to the inner (high-density) side beyond
. The resonance condition at R = 0.93 m, where the significant
increase was observed, was considered in regard to the 8.2 GHz heating effect for bulk and/or mildly energetic electrons. Although the position of R = 0.93 m is significantly far from
, the bulk and mildly energetic electrons are expected to be in resonance with the electrostatic wave of relatively large parallel refractive index
to the magnetic field, as discussed below. The position of R = 0.93 m was roughly half the minor radius, a/2.
Figure 9. Radial profiles of electron cyclotron frequency , plasma frequency
, and upper hybrid resonance frequency
on the 25 kA over-dense plasma at t = 2.55 s.
Download figure:
Standard image High-resolution imageThe nth harmonic relativistic Doppler-shifted ECR condition with parallel refractive index is introduced. The nth harmonic ECR condition is expressed as
with relativistic factor
, where
and
are the normalized electron momenta in the perpendicular and parallel directions to the magnetic field, respectively. Here,
and f are the parallel electron velocity and operating frequency, respectively. Furthermore, m is the electron rest mass, and c is the speed of light. The ECR condition can be rewritten as

As is well known, this equation can be reduced to an elliptical equation of [,
] when
[12, 27], whereas it is reduced to parabolic and hyperbolic equations when
and
, respectively [28].
The parallel refractive index is expressed as
, where
and
, and
and
are the refractive indexes and magnetic field components in the toroidal and poloidal directions, respectively; and B is the magnetic field strength. The toroidal component of
is normally dominant in the
evolution; however, the poloidal component of
can become significant in electrostatic waves. The perpendicular refractive index
to the magnetic field should be less than unity for electromagnetic waves, whereas it can become large beyond unity for electrostatic waves. For instance,
may be an order of
for EBW, where
is the Larmor radius of the electron.
is linked to
through total magnitude of the refractive index N; specifically,

where is a radial component of the refractive index in the toroidal geometry.
would become large beyond unity as well as N and
.
Figure 10 shows, for the fundamental ECR conditions, various plots for several
s at R = 0.93 m where
. The minimum
satisfying the resonance condition is expressed as
, and then
= 0.81 at R = 0.93 m. In the figure, small ellipses, each with a relatively large offset of the origin
of 1.8, appear for
. The major (
and minor (
radii,
and
, of the ellipse are defined as


Figure 10. Various relativistic Doppler-shifted resonance curves of the 8.2 GHz fundamental electron cyclotron wave in normalized momentum space [,
] for several
values at R = 0.93 m, where
= 0.59. The normalized parallel and perpendicular momenta to the magnetic field,
and
, are defined as
and
with parallel and perpendicular electron velocities
and
, respectively. Here, m is the electron rest mass, and c is the speed of light.
is the parallel refractive index to the magnetic field, and f is the operating frequency of 8.2 GHz.
Download figure:
Standard image High-resolution imageWith increasing
, the radii and origin offsets of the ellipse become large. The parabolic curves for
=
with x-axis intercepts
of
0.55 are also shown in the figure. In addition, hyperbolic curves with x-axis intercepts of small and large magnitudes
are plotted for
=
. As the Doppler-shifted EC resonance effect is taken into account through the product
, electrons with
are in resonance with waves for
under ray-scrambling situations, whereas there is an asymmetry of
at a specific
.
If an sign is specified with the focusing and steering beam, the resonance
range becomes asymmetric on its
region, in particular, to the electrostatic wave resonance as suggested from the hyperbolic resonance structure with small and large magnitudes of x-axis intercepts. The asymmetry of the hyperbolic resonance is much stronger than that of the elliptical resonance. Therefore, the plasma current can be driven more effectively by the EBWHCD with a hyperbolic resonance than by the ECHCD with an elliptical resonance, if the incident wave is properly absorbed at specific
s. The
evolution of the electrostatic wave notably depends on the propagating poloidal position and its direction, because the poloidal component
becomes significant in the evolution. If the launching aperture and the propagating poloidal position expand over the upper and lower sides for the mid-plane, the rays are not easily absorbed at specific
s after mode conversion to the EBW [18, 28].
During the non-inductive plasma-current start-up, a Pfirsch–Schlüter current may be created as a seed current on the open field lines if the vertical motion from toroidal drift is cancelled by the vertical component of the parallel velocity
determined by the magnetic field pitch angle [29]. Therefore, there is an asymmetric property of
imposed on the electron confinement in the current start-up phase at the open field lines. Moreover, a different radial shift or deviation of the passing electrons with
from the magnetic surface contributes to the plasma-current ramp-up in the inner limiter configuration after the formation of closed flux surfaces. The ramped plasma current is enhanced by the ECW even in the ray-scrambling situation with
, as
has an asymmetric distribution in the
directions in the start-up phase. Banana orbits are possible even in open field lines with a magnetic mirror with
. A relatively high
favors the confinement of energetic electrons generated by the ECW. The confined trapped electrons also contribute to current generation through precession in the toroidal direction. The electron heating effect and mechanism by the ECW and EBW should be properly assessed for such non-inductively EC ramped-up plasmas.
In regard to bulk electron heating, figure 11(a) shows a semicircle with constant energy of 50 eV as well as some resonance hyperbolic curves in the [,
] plane. The intersection point between the resonance curve and the constant-energy semicircle indicates the resonance condition of the electron for a specific energy. Because the semicircle radius of
was so small for the 50 eV electron, there was no intersection point with the resonance ellipse when
. The hyperbolas with larger
may have intersection points with the constant-energy semicircle. These 50 eV electrons are in resonance with the electrostatic waves for
. Although
may be larger than unity, the required
is an order of magnitude greater than that expected from previous ray-tracing analyses [18, 28] with various incident
s. It is unlikely the electrons were directly heated by the 8.2 GHz electrostatic wave in the over-dense region, even if the tail components of the Maxwell distribution were in resonance with the wave for somewhat smaller
.
Figure 11. Semicircles with constant energy of (a) 50 eV and (b) 5 keV and 10 keV, as well as some resonant hyperbolic curves in the [,
] plane with several
s.
Download figure:
Standard image High-resolution imageThe AXUV diagnostic, which is sensitive in the SX energy range (>0.3 keV), showed extremely enhanced signals in the over-dense plasma. The EBW heating effect on mildly energetic electrons was considered here. The constant-energy semicircles for 5 and 10 keV electrons are plotted in figure 11(b), together with some resonance hyperbolas for several s. Such mildly energetic electrons may be in resonance with the electrostatic waves for moderate
of 2–3, as shown in the figure. The possibility that mildly energetic electrons were directly heated by the 8.2 GHz electrostatic wave with reasonable
is indicated, taking the resonance condition into account. Moreover, whether such electrostatic waves may be excited should be considered. The ray-tracing approach was not available for consideration of the wave excitation under the ray-scrambling situation with multiple wall reflections.
Concerning wave excitations, dispersion with the bulk and energetic electron components was considered. The dispersion D is expressed in the electrostatic approximation [30] in the form,
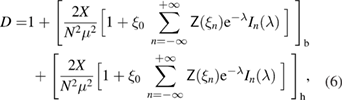
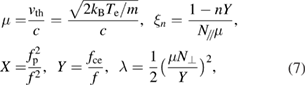
where is the Boltzmann constant, and
is the electron temperature. The plasma dispersion function
is used in the non-relativistic limit, and In is the nth-order modified Bessel function. Here, the subscripts (b) and (h) signify that the associated quantity refers to the bulk and energetic electron components, respectively. The bulk electron temperature
is set to 50 eV, and the proper
giving D = 0 is determined for a specific
and energetic electron temperature
. Furthermore, as the characteristic wave-damping length is 2
, the imaginary part of D is evaluated to take into account heating effects on the energetic electrons considered.
Figure 12 shows the evaluated dependences of and
on the energetic electron temperature
for several
s at R = 0.93 m and 0.80 m. The
range of (1,3] was considered for electrostatic waves with hyperbolic resonance conditions. The energetic electron density
was taken to be 3% of the bulk electron density
. Because
= 0.1 at
keV, a larger range than this value is marginal in the non-relativistic limit. Given a proper
for a specific
, when the dispersion D becomes zero, a wave with a specific
and
may be excited in the plasma. As the
is mainly determined by the bulk electron component
,
should be almost constant despite various
values. The imaginary part
is expressed with the imaginary part of
,
, depending on
. The magnitude of the imaginary part increases as
increases, because the exponent magnitude of
decreases with increasing
. It then starts to decrease as
with larger
when
increases further. The exponent magnitude
is normally large because of the smallness of
; however, when
,
. The condition
(at
) corresponds to the nth Doppler-shifted resonance condition in the non-relativistic limit. In general, the
or
range with significant wave absorption diminishes when taking larger
, as expected from the hyperbolic resonance condition, because the factor
and the argument
of
are expressed with the product
.
Figure 12. Dependences of the perpendicular refractive index to the magnetic field and
on energetic electron temperature
with various
s at (a) R = 0.93 m and (b) 0.80 m. Proper
satisfying dispersion relation D = 0 is found for specific
and
. An imaginary part of D,
, is evaluated to consider the heating effect on the considered energetic electrons as the characteristic wave-damping length is described as 2
.
Download figure:
Standard image High-resolution imageFirst, the results of the dispersion analysis at R = 0.93 m are discussed in regard to the bulk electron heating effect observed in the TS measurements. A weak dependence of –23 on
was reasonably obtained for several
s. The
range with significant
is reduced for larger
.
may grow to a large value for larger
, because
does not dominate at lower
. Although the hyperbola with
2 crosses the 10 keV constant-energy circle, the wave with
= 2 may start to be absorbed even in the energy range less than 0.5 keV, as suggested from the
analysis. The waves may be absorbed by the tail electrons of the relatively lower than expected
from resonance condition analysis. Mildly energetic electrons of around 1 keV may be heated by the electrostatic EBW with
at R = 0.93 m. Thus, the bright AXUV signal after the second SDJ can be explained by the EBW heating effect on the mildly energetic electrons of ∼1 keV in the over-dense region.
The bulk electron heating suggested from the TS measurements was considered from the point of view of the relaxation processes from mildly energetic (1 keV) to bulk (50 eV) electrons. The heat-exchange time is about 16 ms, and its relaxation power density
may be significant (∼12 kWm−3) in heating the bulk electrons. The increments of
and
in the over-dense region may be due to heat exchange from the mildly energetic electrons heated by the electrostatic EBW. There was concern over the linkage of electron heating between the inner (high-field) and outer (low-field) sides of the same flux surface. However, concerning the heating effect, as
shifts inward after the second SDJ, the outer position of R = 0.93 m is probably not on the same flux surface as the inner position with high
near
. The observed bulk electron heating may not be explained by the 28 GHz ECHCD effect and its related effects.
A weak bulk electron heating effect was observed at R = 0.80 m. Hence, the results of dispersion analysis at R = 0.80 m are assessed in comparison with those at R = 0.93 m. The parameters X and Y with and
at R = 0.80 m were used in this analysis. The perpendicular refractive indexes
s
–52 were evaluated with weak dependence on
for several
s. Because
or
becomes large at R = 0.80 m,
is significantly diminished in terms of
. The evaluated
of R = 0.80 m is an order of magnitude less than that of R = 0.93 m (figure 12). Although the wave absorption has to be evaluated from the wave-damping characteristic length and its path length, in actuality, the small
may be key in explaining the difference between the heating effects at R = 0.93 m and 0.80 m.
Backscattering instability with parametric decay may prevent the wave from mode-converting into the EBW at the UHR [31]. However, the power-density threshold on the instability becomes high when electron temperature at the UHR is high, because the instability is suppressed by Landau damping for lower hybrid waves generated at the parametric decay. As we have not attempted to measure the parametric decay instabilities (PDIs), whether PDIs have occurred or not is uncertain. In the present dual-f experiment,
is relatively high, because the UHR position
m is near the outer position
m on the magnetic flux surface of
at the inboard side. It is likely that the significantly low 8.2 GHz power density under the ray-scrambling situation due to multiple wall reflections did not exceed the power-density threshold with relatively high
.
4.3. 28 GHz heating effect on highly energetic electrons in the over-dense core region
The 8.2 GHz heating effects on bulk and mildly energetic electrons were assessed at R = 0.80 m and 0.93 m. The heating on highly energetic electrons of more than 60 keV was not considered because no significant absorption effects were evaluated from the dispersion analysis. However, the high keV was actually observed in the FWD tangential viewing of
= 0.71 m with the over-dense region, but without the off-axis region near
of 0.32 m. In contrast,
was ∼230 keV in the FWD viewing of
= 0.34 m with the off-axis region. It is likely that there are abundant highly energetic electrons in the over-dense core region, as suggested from the EFIT equilibrium analysis.
The resonance conditions on the highly energetic electrons keV were considered for the 28 GHz wave. For the notably high energetic electrons, higher harmonic heating effects must be properly considered. The third (3rd) 3
and fourth (4th) 4
resonance positions are at
= 0.48 m and
= 0.64 m, respectively. Figure 13 shows the dependences of the second, third, and fourth resonance momentum pitches on R, [
/
] (R), of the 500 keV electrons for several
s. The normalized resonance momentum pitches were derived from intersection points of the resonance ellipses and a 500 keV constant-energy semicircle. The positive pitches were evaluated for up-shifted relativistic Doppler-shift resonances with positive
in this configuration. The waves that could access the core region near
m were considered. A couple of the parallel indexes (
= 0.2, 0.5 and 0.8) were taken at R = 0.80 m, and the
evolution maintaining
was considered along the propagation. A wave for which
approaches unity along the propagation cannot access the inner high-field side further in the low-field side injection. For instance, the wave with
= 0.8 at R = 0.80 m cannot propagate beyond R = 0.64 m. The wave trajectory becomes tangential (
= 1) at R = 0.64 m and then goes out to the low-field side. In the high-field side access after reflection at the inboard limiter, the wave propagates easily to the low-field side. The
of unity at
= 0.22 m becomes 0.275 in propagating to R = 0.80 m while maintaining
=
., all waves after the reflection at the inboard limiter can reach R = 0.80 m and
.
Figure 13. Dependence of the second (2nd), third (3rd), and fourth (4th) resonance momentum pitches on the major radius R, [ /
] (R), of 500 keV electrons for various
s on the 28 GHz wave.
Download figure:
Standard image High-resolution imageThe plotted resonance momentum pitch curves must cut across a zero pitch at a specific but same R for several s, depending on the harmonic number. For instance, the fourth resonance pitch curves for several
s cut cross the zero pitch around
. A broad extent of the Doppler-shifted regions around the relativistic shifted (zero-pitch) position is shown for larger
cases mainly in the up-shifted resonance side, because the waves cannot propagate to the high-field or the down-shifted side beyond R with
= 1 in propagation from the low-field side. The up-shifted resonance contributes effectively to the current drive if there is no down-shifted resonance in propagation from the low-field side, because the down-shifted absorption normally cancels out the current driven by the up-shifted absorption. In general, even if the incident beam is properly controlled to obtain significant single-path absorption, the down-shifted absorption after the up-shifted resonance reduces the current drive efficiency if the up-shifted absorption is not perfect.
Resonance overlapping between different cyclotron harmonics appears in the core region. The resonance momentum pitches depend on the harmonic number. Although the absorption at the lower harmonic resonance is strong, synergetic heating is expected because of overlapping resonances. Such heating along with higher harmonic resonances is one of the candidates for the heating and maintenance mechanism of the highly energetic electrons in the core region. The growth process of the highly energetic electrons may be considered with the simultaneous heating effect of the dual-f wave in the low-density phase. The energetic electrons ∼80 keV may be generated with the 8.2 GHz wave in the core region near at the low-density state, as expected from the previous 8.2 GHz EC plasma ramp-up experiments. The incident 28 GHz wave must be significantly absorbed by the energetic electrons at the higher (third and fourth) harmonic ECRs. Therefore, the generated energetic electrons may be accelerated more in the low-density phase with the dual-f waves. This heating effect with the dual-f waves may be verified if the polarized incident beams are properly focused with specific
s for the dual-f waves, a topic that will be addressed in future work.
4.4. Spontaneous density jump phenomena
The TS density already exceeded
at R = 0.64 m even at t = 1.85 s before the second SDJ, and the core density rose rapidly during the SDJ. Concerning the SDJ process, the plasma produced effectively in the core region near
was notably a higher-density plasma, probably formed through wave–plasma and/or some interactions of the bulk electrons. The electron heating effect on the effective density rise was considered through the dispersion analysis at R = 0.64 m. The bulk electron temperature
was fixed at 20 eV in this analysis. The parameters Y was evaluated with
at R = 0.64 m, and two
parameters were used for the electron density of 1
1018 m−3 and 2
1018 m−3 before and after the second SDJ (at t = 1.85 s and 2.55 s), respectively. Figure 14 shows the dependences of
and
on the energetic electron temperature
in the high- and low-density cases before and after the second SDJ at R = 0.64 m for several
s. The bulk terms of
mainly determined
with
s and Y at R = 0.64 m. Relatively large
s (∼167 and 209 in the low- and high-density cases) were obtained with weak dependences on
for several
s. As
is smaller at R = 0.64 m near
, the
range with significant
diminishes.
is significantly large, and therefore,
at R = 0.64 m becomes smaller than that at R = 0.80 m in terms of
. However,
for the high-density plasma was the same as that in the low-density plasma even with larger
. A larger
satisfying D = 0 in the high-density case was evaluated to compensate twice the
of the low-density case. When
increases linearly with increasing
, for both low- and high-density plasmas, the factors in the independent
on
were also identical in the energetic electron component, thereby explaining the same absorption effects for both cases. A modeling of the increment in
together with
was supported from the extremely enhanced AXUV signals after the second SDJ. The heat-exchange time
decreased by half if
increased twofold with increasing
. The heat-exchange power then quadrupled with the doubling of
and
in the high-density case, suggesting effective plasma production and maintenance with non-decreasing
even in the high-density plasma. The core
after the second SDJ increased by more than twice as much as before the second SDJ. Although
becomes smaller at R = 0.64 m, the heat exchange from the
component ∼0.5 keV heated by the electrostatic EBW with
should be effective in increasing the density through increased ionization and sustaining the high-density plasma against radiation cooling.
Figure 14. Dependences of and
on energetic electron temperature
in high- and low-density plasmas before and after the second SDJ at R = 0.64 m for various
s.
Download figure:
Standard image High-resolution imageDuring the EC non-inductive plasma ramp-up, energetic electrons were created to generate the plasma current effectively. However, it has been rather difficult to heat bulk electrons in non-inductively ramped-up plasmas after creation of these energetic electrons [21]. The incident ECW power seems to couple into the highly energetic electrons easily even in the ray-scrambling situation with multiple wall reflections. Evaluation of the synergetic heating with higher harmonic resonances at ∼500 keV tells us that heating on the highly energetic electrons is performed effectively with a feedback process in association with weaker heat exchanges or collisions between the energetic and bulk electrons. As becomes larger in the electrostatic wave, the
term could suppress the EBW absorption via the highly energetic electrons. Absorption was still effective enough in the higher-density plasma to heat the mildly energetic and bulk electrons through heat exchanges under stable equilibrium with highly energetic electrons. The effective EBW heating may start to increase the density and maintain an over-dense plasma even if the density is beyond the cutoff. Effective plasma production through the electrostatic EBW heating appeared as the SDJ of a bifurcation phenomenon.
5. Conclusions
Non-inductive electron cyclotron plasma ramp-up experiments were conducted with dual-f (8.2 GHz and 28 GHz) waves. The SDJ was found in the dual-f experiment as a bifurcation phenomenon. A 28 GHz wave was injected into the pre-ionized plasma with an 8.2 GHz wave to ramp up the plasma current. Dual-f waves were simultaneously injected after the non-inductive plasma ramp-up. The over-dense 25 kA plasma on the 8.2 GHz wave was non-inductively ramped up and sustained for 0.4 s after two SDJs in a shot. The bulk electron pressure measured in TS diagnostics increased at a major radius R = 0.93 m in the over-dense region after the second SDJ. AXUV-bolometer intensities with significant sensitivity in the soft x-ray energy range were extremely enhanced after the second SDJ, indicating effective EBWH of the bulk and/or mildly energetic electrons. The heating effects of the bulk and mildly energetic electrons were discussed with dispersion analyses in the electrostatic approximation. The wave excitation with reasonable perpendicular and parallel refractive indexes ( and
) to the magnetic field was considered through the dispersion analyses, and the wave-damping characteristic length or imaginary part of the dispersion was also evaluated in regard to wave absorption. Mildly energetic electrons around 1 keV could be heated by the electrostatic EBW with
at R = 0.93 m. Weak heating of the mildly energetic electrons was estimated at R = 0.80 m in comparison to that at R = 0.93 m, corresponding to the smaller TS bulk pressure increase at R = 0.80 m. The observed bulk electron heating effects were explained by heat exchange to the bulk electrons from the mildly energetic electrons heated with the electrostatic EBW.
The plasma equilibrium of the over-dense 25 kA plasma was analyzed with EFIT code. The plasma or electron pressure required for the equilibrium was larger than 400 Pa near the axis of its bell-shaped profile. A high HX radiation temperature of ∼500 keV was evaluated from the HX spectrum at forward tangential viewing, mainly including the over-dense core region. It is most likely there were abundant highly energetic electrons in the over-dense core region as suggested from the EFIT equilibrium analysis. Relativistic Doppler-shifted resonance conditions were considered for the 500 keV electrons in the core region. Synergetic heating through overlapping harmonic resonances as well as higher harmonic heating was indicated in maintaining highly energetic electrons in the core region. Because of the growing-up process of the highly energetic electrons, the heating effect at the 28 GHz higher harmonic resonances must be considered for the energetic electrons with the 8.2 GHz wave in the core region to be accelerated effectively in the low-density phase.
The SDJ process and mechanism were considered based on the electron heating analysis. The density profile was peaked beyond the cutoff in the core region even at t = 1.85 s before the second SDJ, and the core density rose rapidly during the SDJ. In regard to this SDJ process, the over-dense plasma was effectively produced in the core region, probably through wave–plasma interaction and some interactions of the bulk electrons. The EBWH effects in the lower- and higher- density plasmas before and after the second SDJ were assessed following the dispersion analysis at R = 0.64 m in the core region. Although the EBW absorption effect evaluated became smaller at R = 0.64 m, it did not decrease in the higher-density plasma in comparison with in the lower-density plasma if the energetic electron density linearly increased with increasing bulk density in the SDJ. Furthermore, the heat exchange to the bulk electrons rather increased in the higher-density plasma, because of the increase in bulk density and the decrease in heat-exchange time with the increase in energetic electron density. The core bulk electron pressure after the second SDJ increased by more than twice as much as before the second SDJ, indicating effective electron heating in the higher-density plasma. The effective EBWH begins to increase the density and sustains the over-dense plasma once the density exceeds the cutoff. The effective heating and production of plasma with electrostatic EBWH even in the higher-density plasma appears as a mechanism of the SDJ phenomenon.
In the dual-f experiments, the highly energetic electrons required for stable plasma equilibrium may be effectively created with the 28 GHz wave. The bulk electrons may be heated by the relaxation process from mildly energetic electron components heated with the 8.2 GHz EBW, as expected from the dispersion analysis, in the stable self-organized plasma. In further work to clarify the EBWH effect with increasing electron pressure in the over-dense region as well as on the SDJ mechanism, the incident waves must be controlled well to produce clear heating scenarios with single-path absorptions. We plan to measure parametric decay instabilities to answer whether or not the backscattering instability is occurring, and whether more efficient EBWH without the instability can be achieved with better-controlled 8.2 GHz wave injection.
Acknowledgments
This work was performed under the auspices and support of the NIFS Collaboration Research Programs (NIFS09KUTR046/NIFS10KUTR048/NIFS11KUTR059/NIFS11KUTR069). This research was partially supported by the Ministry of Education, Science, Sports and Culture, Grant-in-Aid for Sc. Res. (B) [15H04231].