Abstract
Stoichiometric near-room-temperature superconductors (NRTS) (for instance, H3S and LaH10) exhibit a high ground-state upper critical field, Bc2(0) ⩾ 100 T, so that the magnetic phase diagram in these materials cannot be measured in non-destructive experiments. However, (Semenok et al 2022 Adv. Mater.) proposed the idea of exploring the full magnetic phase diagram in NRTS samples, in which the superconducting order parameter is suppressed by magnetic element doping. If the elements areuniformly distributed in the material, then the theory of electron–phonon mediated superconductivity predicts the suppression of the order parameter in a 3D s-wave superconductor. (Semenok et al 2022 Adv. Mater.) experimentally proved this idea by substituting lanthanum with the magnetic rare-earth neodymium in (La1−xNdx)H10−y. As a result, the transition temperature in (La1−xNdx)H10−y (x = 0.09) was suppressed to Tc ∼ 120 K, and the upper critical field decreased to Bc2(T= 41 K) = 55 T. While the exact hydrogen content should be further established in the (La1−xNdx)H10−y (x = 0.09) (because similar Tc suppression was observed in hydrogen-deficient LaH10−y samples reported by Drozdov et al (2019 Nature 569 528)), a significant part of the full magnetic phase diagram for the (La1−xNdx)H10−y (x = 0.09) sample was measured. Here, we analyzed the reported (Semenok et al 2022 Adv. Mater.) magnetoresistance data for (La1−xNdx)H10−y (x = 0.09) compressed at P = 180 GPa and deduced: (a) Debye temperature, ; (b) the electron–phonon coupling constant,
; (c) the ground-state superconducting energy gap,
; (d) the gap-to-transition temperature ratio,
; and (e) the relative jump in specific heat at transition temperature,
. The deduced values indicate that (La1−xNdx)H10−y (x = 0.09; P = 180 GPa) is a moderately strongly coupled s-wave superconductor.
Export citation and abstract BibTeX RIS
1. Introduction
The discovery of a superconducting state with a transition temperature above 200 K in highly compressed H3S by Drozdov et al [1] with the consequent discovery of high-temperature superconductivity in superhydrides of thorium [2] and near-room-temperature superconductivity (NRTS) in superhydrides of lanthanum [3, 4], yttrium [5, 6] and lanthanum–yttrium [7] represent the most fascinating scientific explorations in the field of superconductivity since the discovery of high-temperature superconductivity in cuprates [8].
While in the majority of theoretical works (a comprehensive review has been published recently [9]) the electron–phonon mechanism is considered to be the primary pairing mechanism in NRTS superhydrides, there are several new and alternative approaches to the nature of charge carrier interaction, and pairing in NRTS is also under development [10–14]. One of the most solid experimental facts that supports the electron–phonon pairing mechanism in superhydrides is the prominent isotope effect with respect to the transition temperature, Tc [1, 3, 15]. However, the effect of hydrogen–deuterium exchange on other fundamental parameters of NRTS superhydrides, for instance, on the lower and upper critical fields, as well as on Bardeen–Cooper–Schrieffer (BCS) ratios (i.e. where
is the ground state of the superconducting energy gap,
is the Boltzmann constant), remains to be explored.
Another important question that needs to be answered is the superconducting gap symmetry in NRTS hydrides. To the author's best knowledge, there is general agreement [9] that the superconducting energy gap in superhydrides exhibits s-wave symmetry. However, the first experimental evidence that confirms s-wave gap symmetry was only recently reported by Semenok et al [16]. This research group proposed to verify the s-wave gap symmetry in NRTS by employing one of the conclusions of Abrikosov–Gor'kov [17], Anderson [18] and Openov [19, 20] theories of dirty superconductors. The conclusion is that uniformly distributed (on the atomic level) impurities exhibiting magnetic moment should suppress the superconducting order parameter in s-wave superconductors. However, this kind of impurity should not affect the superconducting order parameter in d-wave superconductors. Nevertheless, non-magnetic impurities should cause the suppression in d-wave superconductors [19, 20], but this kind of doping should not affect the s-wave superconducting state [17–20].
Thus, to reaffirm/disprove s-wave symmetry gap in LaH10, Semenok et al [16] performed gradual doping of lanthanum decahydride by the magnetic rare-earth element, neodymium. Hydrogen and rare-earth element (i.e. H:(La + Nd)) stoichiometry was targeted to be 10:1 in all samples. However, the exact hydrogen content in the synthesized samples of La1−x Ndx H10−y (x = 0.08, 0.09, 0.20, 0.25 and 0.50) [16] should be further established because similar Tc suppression was observed in hydrogen-deficient LaH10−y samples reported by Drozdov et al [3]). In addition, there is a need for further experimental confirmation that all Nd atoms replace the lanthanum at their sites in the crystal lattice. This is important, because Nd can form its own hydride phases, while the total hydrogen content might remain stoichiometric in the mixture of the two phases.
Semenok et al [16] reported on gradual suppression upon the increase in the Nd concentration. One of the interesting consequences associated with this
suppression is that the upper critical field,
, is also decreasing. This makes it possible to measure
for La1−x
Ndx
H10-y within a much wider reduced temperature range,
, in comparison with the range available for undoped stoichiometric NRTS materials H3S [21] and LaH10 [22], which exhibits a ground state upper critical field well above the value that is measurable in non-destructive experiments.
In this paper, we analyzed reports by Semenok et al [16] on temperature-dependent resistance, , and the upper critical field,
, and deduced the following fundamental parameters for the La1−x
Ndx
H10−y
(x = 0.09;
) superconductor:
- (a)Debye temperature,
;
- (b)Electron–phonon coupling constant,
;
- (c)Ground-state energy gap, Δ(0);
- (d)Relative jump in electronic specific heat at Tc,
(where
is the Sommerfeld constant);
- (e)Ground-state coherence length, ξ(0);
- (f)Gap-to-transition temperature ratio,
(where
is the Boltzmann constant).
- (g)Fermi temperature, TF.
2. Results and discussion
2.1. Temperature-dependent resistance of La1−xNdxH10−y (x = 0.09; P = 180 GPa)
Debye temperature can be deduced from the fit of experimentally measured temperature-dependent resistance, , to the Bloch–Grüneisen equation [23, 24]:

where and
are free-fitting parameters. From the deduced
and measured
, the electron–phonon coupling constant,
, can be calculated as a unique root of the advanced McMillan equation [25, 26]:

where


where is the Coulomb pseudopotential parameter, which can be assumed to be
for all NRST materials.
The fit of data set to equation (1) is shown in figure 1. This
data set reported in figure 2(a) in [16] and the raw data are freely available online by Semenok et al [27]. The deduced parameters are:
,
and
. By utilizing the general requirement [26] that
should be defined at the lowest possible
ratio, and considering that the same criterion should be used to define the
data set from the
curves (reported in figures 2(b) and (c) in [16]), the ratio of
was used. In the result,
for
in figure 1 was defined as
.
Figure 1.
R(T) data for highly compressed La1−x
Ndx
H10−y
(x = 0.09; P = 180 GPa) and the data fit to equation (1) (raw data are freely available online from Semenok et al [16, 27]). Green balls indicate the bounds for which R(T) data were used for the fit to equation (1). Deduced ,
,
, fit quality is 0.9977. Pink shaded area shows 95% confidence band.
Download figure:
Standard image High-resolution imageThe root of equations (2)–(4) for given ,
and
is
. The deduced values are in good agreement with
values calculated by first-principles calculations by Semenok et al [27] in their table S5.
2.2. Temperature-dependent upper critical field of La1−xNdxH10−y (x = 0.09; P = 180 GPa)
By utilizing the same criterion of , the
dataset was derived from the
curves shown in figures 2(b) and (c) of [16]. In figure 2, the
data set is fitted to the equation for temperature-dependent upper critical field for s-wave superconductors [28, 29]:
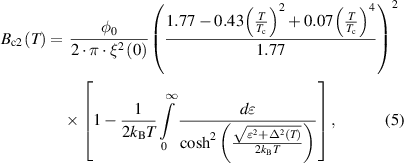
Figure 2. Superconducting upper critical field data, Bc2(T), and data fit to equation (5) for highly compressed La1−x
Ndx
H10−y
(x = 0.09; P = 180 GPa). Raw R(T,B) data set is freely available online from Semenok et al [16, 27]. Deduced parameters are: ,
,
,
. Fit quality is 0.9976. Pink shaded areas show 95% confidence bands.
Download figure:
Standard image High-resolution imagewhere the amplitude of temperature-dependent superconducting gap, Δ(T), is given by [30, 31]:

where η = two-thirds for s-wave superconductors.
The fit converges with a high quality (with goodness-of-fit R = 0.9976) (figure 2). The deduced parameters are: ,
and
,
. Considering that the weak coupling limits of the BCS theory [30–32] are
and
, and the upper limits for low-Tc electron–phonon mediated superconductors are
(for Pb0.50Bi0.50 alloy [33]) and
(for Pb0.70Bi0.30 alloy [33]), one can conclude that La1−x
Ndx
H10−y
(x = 0.09;
) is moderately strongly coupled superconductor.
2.3. La1−xNdxH10−y (x = 0.09; P = 180 GPa) in the Uemura plot
Final characterization of the superconducting properties of La1−x
Ndx
H10−y
(x = 0.09; ) was to position this hydride in the empirical Uemura plot [34, 35], where heavy fermions, fullerenes, cuprates, pnictides and hydrogen-rich superconductors [28, 36, 37] form a narrow band that exhibits the ratio of the superconducting transition temperature, Tc, to the Fermi temperature, TF, within a range:

while all low-Tc conventional superconductors have much smaller ratio:

The Fermi temperature can be calculated by following equation [37]:

where all parameters were deduced above. In the result, calculated Fermi temperature is and, thus,
for this superhydride. As a result, La1−x
Ndx
H10 (x = 0.09;
) falls into the unconventional superconductor band in the Uemura plot (figure 3), and is located in close proximity to YBa2Cu3O7−δ and Bi2Sr2Ca2Cu3O11 cuprates and other NRTS counterparts.
Figure 3. Uemura plot (Tc versus TF), where the La1−x
Ndx
H10−y
(x = 0.09; ) compound is shown together with other superconducting families: metals, heavy-fermions, pnictides, cuprates and near-room-temperature superconductors. Reference to the original data can be found in [36].
Download figure:
Standard image High-resolution imageTo address the possible issue of the electron–phonon mediated materials being located in the unconventional superconductor band, we should point out that superhydrides are not the only known electron–phonon mediated superconductors that are located in the unconventional superconductor band in the Uemura plot (for instance, we can mention bulk s-wave A3C60 (A = K, Rb) superconductors).
On the other hand, the Uemura plot is not intended to reveal the pairing mechanism, but rather indicates the geometrical ratio of the characteristic size of the Cooper pair (which is proportional to ) with average spatial distance between the centers of the Cooper pairs. This implies that materials with low superfluid density tend to locate closer to the Bose–Einstein condensate (BEC) line,
, while materials with high volume concentration of Cooper pairs tend to be located closer to pure metals, such as aluminium, for which
. This limit is also known as the BCS limit. Detailed studies of the transition of the same material from BEC to BCS pairing state (under high pressure) can be found elsewhere [38].
3. Conclusion
We summarize our findings and mention that while the detection of superconductivity in elemental highly compressed hydrogen is an ongoing task [39–41], the near-room-temperature superconductivity has been observed in several superhydires [1–7]. One of these superhydrides, LaH10 [3, 4], represents a versatile platform to study the physics of NRTS because Tc and other superconducting parameters in this compound can be modified by:
- (a)Hydrogen stoichiometry [3];
- (b)
- (c)
Here, we analyzed experimental magnetoresistance data, R(T,B), for highly compressed La1−x
Ndx
H10−y
(x = 0.09; ) superconductor in which the superconducting order parameter was suppressed by magnetic rare-earth element (neodymium) impurity. Raw experimental R(T,B) data sets for La1−x
Ndx
H10−y
(x = 0.09;
) were recently reported by Semenok et al [16, 27]. Deduced parameters, such as the gap-to-transition temperature ratio,
, and the relative jump in specific heat at the transition temperature,
, indicate that La1−x
Ndx
H10−y
(x = 0.09;
) is moderately strongly coupled superconductor. This hydride exhibits a ratio of the superconducting transition temperature to the Fermi temperature of
, and it falls into the unconventional superconductor band in the Uemura plot.
Acknowledgments
The author thanks Dmitrii V Semenok (Skolkovo Institute of Science and Technology) and co-workers of [16, 27] for making raw experimental data is freely available prior the peer-review publication of their paper.
The research funding from the Ministry of Science and Higher Education of the Russian Federation (theme 'Pressure' No. АААА-А18-118020190104-3 and Ural Federal University Program of Development within the Priority-2030 Program) is gratefully acknowledged.
Data availability statement
The data that support the findings of this study are available upon reasonable request from the author.
Declaration of interests
The author declares that he has no known competing financial interests or personal relationships that could have appeared to influence the work reported in this paper.