Abstract
The effect of Al2O3 and BaTiO3 packing on the plasma-enhanced NOx synthesis from air was investigated in a packed-bed dielectric barrier discharge (DBD) reactor. The discharge characteristics are significantly influenced by packing different materials into the discharge gap. Compared with the DBD without packing, the presence of Al2O3 or BaTiO3 beads in the discharge effectively enhanced the charge accumulation, average electric field, and mean electron energy, all of which contribute to the enhanced production of NOx. The lowest energy consumption of 15.9 MJ mol−1 for NOx production was achieved when placing BaTiO3 beads in the DBD, which can be attributed to the increased mean electron energy using the BaTiO3 packing, facilitating the formation of more N2 excited species in the reaction. These results suggest that choosing proper packing materials can effectively reduce the energy cost for plasma NOx synthesis using DBD.
Export citation and abstract BibTeX RIS

Original content from this work may be used under the terms of the Creative Commons Attribution 4.0 licence. Any further distribution of this work must maintain attribution to the author(s) and the title of the work, journal citation and DOI.
1. Introduction
Artificial nitrogen fixation is essential for agriculture and modern fertiliser production. The commercialisation of the Haber–Bosch (H–B) process—large-scale ammonia synthesis from the reduction of atmospheric dinitrogen—in the early 1900s enabled the drastic growth of the world's population over the past century [1]. To date, the synthetic fertilisers from N2 fixation are undoubtedly a boon to food production and responsible for sustaining approximately 40% of people on Earth [2]. However, nitrogen fertiliser production through the commercial H–B process is energy-intensive and accounts for more than 1% of the global energy consumption [3]. In addition, the current H–B process is highly dependent on the hydrogen produced from fossil fuels (primarily natural gas) and emits 1.2% of the anthropogenic CO2 on an annual basis [4]. Furthermore, the rigorous reaction conditions of the H–B process require continuous and large scale operation, making it difficult for decentralised nitrogen fixation powered by renewable energy sources (e.g. wind and solar), particularly intermittent and geographically isolated renewable energy. Therefore, developing sustainable, green, and efficient processes for decentralised nitrogen fixation using intermittent renewable energy has become a grand challenge that merits further investigation.
Non-thermal plasma (NTP) has been considered as a promising and emerging technology for sustainable N2 fixation under mild conditions [5]. NTP allows the thermodynamically stable and kinetically inert dinitrogen to be activated at low temperatures by the electron-induced highly reactive chemical mixture; this activation is extremely challenging at atmospheric pressure [6, 7]. As a result, external heating or a high energy input can be simply replaced by applying an electromagnetic field, this makes NTP a compact, flexible option that can be easily switched on and off. This unique feature enables NTP to accommodate intermittent sustainable energy in the context of on-site N2 fixation, especially for wind farms and solar parks [8, 9]. Moreover, the modern ammonia-producing plant is localised yet the application of fertilisers is highly decentralised [10]. The potential application of plasma-based nitrogen fertiliser production in proximity to agricultural areas can provide a solution for the imbalance between energy generation and consumption.
As the ammonia synthesis typically requires an expensive hydrogen feedstock, plasma-activated NOx production from the air has attracted growing interest for fertiliser production where the produced nitrogen oxides can be used as precursors to form nitric acid (HNO3). The plasma-based NOx synthesis through dinitrogen oxidation has a low theoretical limit of energy consumption at about 0.2 MJ mol−1, which is over 2.5 times more energy-efficient compared to the H–B process even when using methane-derived H2 [11]. Moreover, fulfiling the demand for oxidised nitrogen through NOx synthesis from air possesses many advantages, being abundant, inexpensive, and widely available in nature. Even so, precautionary measures should be taken in the future applications to prevent leaks of synthesised nitrogen oxides into the atmosphere as they may cause environmental problems.
To date, various types of NTP have been investigated for NOx synthesis [12–21]. Patil et al investigated the plasma-catalytic NOx synthesis in a packed-bed dielectric barrier discharge (DBD) reactor, achieving a 0.5% NOx yield using γ-Al2O3 packing with an energy consumption of 18 MJ mol−1 for NOx production [12]. Pei et al compared different plasma systems (e.g. DBD, glow discharge, spark discharge, and propeller arc) for air oxidation, and suggested that the average electric field and gas temperature of the plasma are critical in N2 fixation [13]. Among these plasmas, DBD is attractive as catalytic and non-catalytic materials can be easily packed in the plasma area with great potential to enhance chemical reactions through the physicochemical interactions between the plasma and packing materials [22]. Despite recent progress, limited efforts have been paid to better understand the interactions between DBD and packing materials in NOx synthesis. More specifically, the influence of packing materials on coupled electrical and chemical processes in the plasma-activated NOx synthesis remains underexplored. Well-designed experiments can help fill this knowledge gap and benefit the practical application of the N2 fixation.
In this work, the plasma NOx synthesis from air was performed using a coaxial packed-bed DBD reactor. The electrical characteristics of the discharge including the average electric field and mean electron energy were evaluated using a DBD equivalent model. The effect of Al2O3 and BaTiO3 packing on the selectivity of NOx and energy consumption of this process was examined. The physicochemical interactions between the plasma and packing materials were discussed for the process optimisation of N2 fixation in a packed-bed DBD.
2. Experimental
2.1. Experimental setup
Figure 1 shows the schematic of the experimental setup. The NOx synthesis was conducted in a coaxial DBD reactor. A quartz tube with an external diameter of 13 mm and an inner diameter of 10 mm served as the dielectric layer. A 50 mm long stainless-steel mesh was wrapped around the quartz tube and used as a ground electrode, and a stainless-steel rod with a diameter of 4 mm served as a concentric inner high voltage electrode. The discharge gap was 3 mm, and the discharge volume was 3.3 ml. Different packing materials (Al2O3 and BaTiO3) of 1 mm in diameter were fully packed into the discharge area. The dielectric constants of Al2O3 and BaTiO3 are ∼9 and >1000, respectively. The DBD reactor was connected to a high voltage AC power supply with a frequency of 9.3 kHz and a maximum peak voltage of 30 kV. The applied voltage was measured by a high voltage probe (Testec, TT-HVP 15 HF), while the current was recorded by a current monitor (Magnelab CT-E0.5). The voltage on an external capacitor Cext (0.47 μF) was measured to determine the total charge accumulated in the plasma. These electrical signals were sampled by a four-channel digital oscilloscope (Tektronix, MDO3024). The discharge power was calculated using the area of the Q–U Lissajous figure. The gas temperature in the DBD reactor was measured by a fibre optic thermometer (Omega, FOB102) with the fibre inserted 10 mm into the plasma region through the outlet of the reactor.
Figure 1. Schematic diagram of the experimental setup.
Download figure:
Standard image High-resolution image2.2. Product analysis
The gas products were analysed using an online Fourier-transform infrared (FTIR) spectrometer (FTIR-4200, Jasco) with a resolution of 0.5 cm−1. Air (Zero grade, BOC) was used as the reactant and introduced into the DBD reactor by a mass flow controller (Omega, FMA-2404). The total air flow rate was fixed at 100 ml min−1 for all measurements. The effluent gases passed a standard gas cell with a 10 cm path length placed in the FTIR. The products from the air DBD were determined as NO, NO2, and N2O in all working conditions and the sum of their concentrations was defined as NOx concentration. Calibration gases with known concentrations of NO, NO2, and N2O diluted in N2 were used to quantify the concentration of these products. Each experiment was repeated 3 times, and the margin of error in this work was within 3%.
The selectivity of the NOx product i was calculated using the following equation:

The energy consumption (EC) of the NOx synthesis was determined as:
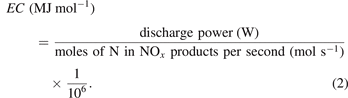
2.3. Evaluation of electrical parameters
Figure 2(a) illustrates the classic equivalent circuit of a DBD reactor, in which Cdiel and Cgap correspond to the capacitance of both the dielectric layer and the capacitance of the discharge gap, respectively. In the presence of packing materials, Cgap includes the contribution of the gas–solid integration in between the electrodes. When the breakdown conditions are reached, and conductive filaments take place, the switch 'K' in the parallel resistive channel will be on.
Figure 2. (a) The equivalent electrical circuit of a DBD reactor and (b) a typical Q–U Lissajous figure of the DBD.
Download figure:
Standard image High-resolution imageThe shape of a Q–U Lissajous figure typically approximates a parallelogram where the internal electrical discharge characteristics can be derived from its dimensions, including the breakdown voltage, reactor charge, and reactor capacitances [23, 24]. Figure 2(b) shows a typical Q–U Lissajous plot where the peak-to-peak charge (Qp–p) accumulated in the DBD can be determined. The lines AB and CD in a Lissajous figure represent the discharge-off phase, during which only displacement current occurs and therefore no charge transfers between the electrodes. The slope dQ/dU of lines AB and CD is equal to Ccell in this period, which is composed of the gap capacitance Cgap and the dielectric capacitance Cdiel, as demonstrated in the following equation:

The lines BC and DA correspond to the discharge phase, where the plasma is ignited, and the air gap is shortcut. The slope dQ/dU of BC and DA represents the effective capacitance Ceff which equals the dielectric capacitance Cdiel in a fully bridged gap [25]. The gap capacitance Cgap is then calculated as:

The total charge in the plasma Q can be acquired from the voltage Uc on an external capacitor Cext of 0.47 μF. The voltage on the dielectric layers Ud can be calculated using charge Q and Cdiel according to equation (6) [26].


Then the gas voltage (also called gap voltage) Ug of the discharge region will be the difference between applied voltage Ua and dielectric voltage Ud:

The breakdown voltage Ub can be determined using equation (8) where Umin stands for the minimum external voltage derived from the Lissajous figure (Figure 2(b)) [24, 27].

The average electric field (E) can be calculated by the ratio of breakdown voltage to the discharge gap distance dgap:

In the presence of packing materials, the discharge gap is significantly changed. To date, there is no standard approach for the approximation of the discharge gap in a packed-bed DBD reactor. The current established simplified geometrical models based on spherical beads enable the estimation of the average electric field in a packed DBD reactor [22, 28] but do not consider the pores, small voids, and surface curvature of the pellets, and thus readily underestimate the volume of the discharge gap. Here, a method based on water absorption [29] is used to estimate the discharge volume of the packed-bed DBD reactor. In this case, the average electric field is determined as follows:

where the dpacking-gap denotes the equivalent discharge gap in the packed-bed plasma zone and is calculated by equation (11):

where the coefficient β stands for the volume fraction of the gas in the fully packed plasma region and can be calculated with the relation:

where Vpacking-gap represents the gas volume in the discharge area of the packed reactor and Vdischarge denotes the total discharge volume (3.3 ml in this study). For the measurement of Vpacking-gap, the same amount of packing material as used in the experiments was placed inside a measuring cylinder. Deionised water was gradually added into the cylinder using a calibrated volume pipette until it perfectly submerged the pellets and the water level reached 3.3 ml. The volume of deionised water was recorded from readings of the pipette. In the present study, the coefficient β for Al2O3 and BaTiO3 was determined as 0.78 and 0.43, respectively.
3. Results and discussion
3.1. Effect of packed materials on discharge characteristics
The electrical signals of the DBD plasma operating in dry air with and without packing materials are illustrated in Figure 3. In the absence of pellets, a number of strong current pulses can be observed, with the amplitudes of current peaks reaching more than 400 mA. This suggests the presence of a typical filamentary discharge mode where plasma streamers propagate across the full gas gap in the discharge area. The duration of these filaments is in tens of nanoseconds [30]. Packing pellets into the plasma region significantly influences the discharge behaviour of the DBD reactor. More specifically, a transition from full gap discharges to more localised discharges usually takes place when introducing the packing materials in the discharge region [31]. Two typical discharge modes can be observed in a packed-bed DBD: (a) local filamentary discharges that form in the small voids in between the pellets; and (b) surface discharges that spread over the surface of pellets covering the full gas gap due to the packed-bed effect [32, 33].
Figure 3. (a) The electrical signals of the air DBD: (a) plasma only; (b) packed with Al2O3; (c) packed with BaTiO3 (discharge power 15 W).
Download figure:
Standard image High-resolution imageNotably, changing packing materials forms disparate discharge patterns, as shown in Figure 3. Compared with plasma only, the discharge with Al2O3 packing showed similar voltage signals but lower amplitudes of current peaks, indicating the formation of weaker microdischarges when using the Al2O3 packing. Due to the typical packed-bed effect, the discharge behaviour transforms from the filamentary discharge of plasma only to a coupled surface discharge alongside localised microdischarges when using the Al2O3 packing. In contrast to plasma only and Al2O3 packing, the current profiles of the DBD packed with BaTiO3 demonstrated more numerous pulses with lower amplitudes of less than 40 mA, corresponding to the formation of localised filamentary discharges between bead–bead and bead–quartz wall. Evidenced by intensified charge-coupled device imaging of the BaTiO3-packed discharge, local microdischarges are observed in between the pellets, while surface discharges are hardly visible [34]. This phenomenon is associated with the lower β coefficient of BaTiO3 (β = 0.43) when compared with Al2O3 (β = 0.78) and plasma only (β = 1); this low β indicates a limited plasma volume in the reactor. The DBD with BaTiO3 packing showed a lower average gas voltage and thus should be expected to deposit fewer charges per filament, this can be evidenced by the lower amplitudes of the current peaks in Figure 3(c). Simultaneously, packing with BaTiO3 produced more filaments and total charge deposition compared to Al2O3 and plasma only (see Figure 6). The BaTiO3 with a high dielectric constant significantly decreased the applied voltage within the gas gaps and resulted in a lower gas voltage, this may also benefit the charge trapping [34]. Similar observations of disparate current profiles when using different packing materials were also reported in previous studies [12, 35, 36].
Figure 3 shows the time-resolved gas voltage (red colour) of the air discharge. A flat curve of gas voltage takes place simultaneously with the ignition of plasma, this value was above 2 kV for both plasma only and Al2O3 packing and was ∼1.5 kV for BaTiO3 packing. This phenomenon indicates that the gas voltage remains relatively constant over time after the breakdown of the gas, implying that the formation of individual filaments (with a lifetime of nanoseconds) is not affected by the applied voltage after reaching gas breakdown. This constant gas voltage in multi-filament DBD can be ascribed to the development of deposited charge on the dielectric surface [37]. To better validate this effect, the breakdown voltage (Ub) of the DBD was derived from the equivalent circuit and Lissajous figures (Figure 4). The breakdown voltage remained constant when changing the discharge power and was ∼2.6 kV, ∼2.4 kV, and ∼1.5 kV for plasma only, Al2O3 packing, and BaTiO3, respectively, consistent with the observation from the gas voltage signals. Previous studies [31, 35] also reported that packing BaTiO3 into a DBD reactor lowered the breakdown voltage compared to the DBD without packing or with Al2O3 packing.
Figure 4. Effect of packing materials on the breakdown voltages at different discharge powers.
Download figure:
Standard image High-resolution imageFigure 5 shows typical Lissajous figures of the air DBD with and without packing at a constant discharge power of 15 W. The Lissajous figure of the discharge without packing and with Al2O3 packing exhibits a similar parallelogram shape, while the Lissajous figure of the DBD packed with BaTiO3 shows an oval shape, which is likely the consequence of enhanced charge deposition when packing BaTiO3. The changes in the Lissajous shape also demonstrate the transition of the discharge mode. Our previous works proved that packing pellets into a DBD changed the discharge mode due to the reduced discharge volume induced by the packing [22, 38]. At a constant discharge power, the discharge packed with BaTiO3 showed a much higher peak applied voltage compared to the DBD without packing (Figure 5), which can be associated with the significantly reduced amplitude of current pulses due to the formation of confined discharge when using the BaTiO3 packing. As derived from the Lissajous plots, the equivalent capacitance of the discharge gap Cgap increased from 4.6 pF without packing to 9.5 pF and 26.0 pF when packed with Al2O3 and BaTiO3, respectively. This significantly higher gap capacitance in the presence of BaTiO3 packing can be attributed to the great capacity of BaTiO3 to trap charges due to the low β coefficient.
Figure 5. The Lissajous plots of the air DBD plasma with and without different packing materials (discharge power 15 W).
Download figure:
Standard image High-resolution imageAs shown in Figure 6, the peak-to-peak charges accumulated in the DBD increases with the discharge power. Clearly, introducing packing materials into the discharge area greatly affects the charge characteristics of the DBD. The generated charges were significantly enhanced when fully packed with pellets, especially with BaTiO3 beads. In general, the peak-to-peak charges were almost doubled with BaTiO3 packing in comparison to no packing. Moreover, the charge depositions of BaTiO3 packing were also found superior to Al2O3 packing. As shown in Figures 3 and 4, packing with BaTiO3 results in a lower breakdown voltage and generates more filaments than Al2O3 and plasma only due to the lower β coefficient and higher dielectric constant, which contributes to a greater total charge deposition.
Figure 6. Effect of packing materials on the peak-to-peak charges at different discharge powers.
Download figure:
Standard image High-resolution image3.2. Effect of packing materials on the electric field and electron energy
Table 1 illustrates the influence of packing materials on the time-averaged electric field in the DBD at different discharge powers. As discussed above, increasing the applied voltage (and thus the discharge power) at a fixed frequency has no influence on the breakdown voltage, and therefore results in a constant average electric field. Compared with plasma only, introducing packing materials in the plasma zone effectively enhances the electric field. For instance, in this work, the highest average electric field of around 11.5 kV cm−1 was observed in the BaTiO3-packed reactor, while the average electric field of the DBD without packing was only 8.7 kV cm−1. Gallon et al found that materials with a high dielectric constant can greatly enhance the local electric field near the contact points between packing beads [39]. A modelling study also reported that an enhanced average electric field can be achieved when using packing materials with a higher dielectric constant [35]. The influence of packing materials on the calculated reduced electric field is consistent with the time-averaged electric field, as shown in Figure 7. The enhanced reduced electric field at a higher discharge power could be attributed to the decreased neutral particle concentration N due to the increased plasma temperature. The gas temperatures of the DBD with and without packing at different discharge powers are listed in Table 2.
Table 1. Effect of packing materials on the average electric field at different discharge powers.
Discharge power (W) | Average electric field (kV cm−1) | ||
---|---|---|---|
Plasma only | Al2O3 | BaTiO3 | |
9.0 | 8.7 | 10.3 | 11.5 |
11.0 | 8.7 | 10.3 | 11.4 |
13.0 | 8.7 | 10.2 | 11.4 |
15.0 | 8.7 | 10.3 | 11.5 |
16.0 | 8.7 | 10.2 | 11.5 |
Figure 7. The reduced electric field as a function of discharge power with different packing materials.
Download figure:
Standard image High-resolution imageTable 2. Effect of packing materials on the gas temperatures at different discharge powers.
Discharge power (W) | Gas temperature (°C) | ||
---|---|---|---|
Plasma only | Al2O3 | BaTiO3 | |
9.0 | 137.6 ± 0.8 | 143.4 ± 1.0 | 158.8 ± 0.9 |
11.0 | 151.1 ± 1.7 | 154.3 ± 1.4 | 172.8 ± 1.9 |
13.0 | 161.9 ± 1.2 | 168.0 ± 2.1 | 183.5 ± 1.6 |
15.0 | 168.8 ± 1.5 | 176.1 ± 1.2 | 196.0 ± 2.0 |
16.0 | 177.2 ± 1.9 | 182.7 ± 1.7 | 202.6 ± 1.6 |
To get insights into the influence of packing materials on the changes of the electric field and electron energy of the DBD, the rate coefficients of electron impact reactions and electron energy distribution function were calculated using the Boltzmann solver BOLSIG+ (version 12/2019) [40]. The cross-sections of air components were retrieved from the LXCat project database [41]. The electric field has a significant impact on the plasma properties including the mean electron energy and electron density, and thus the prevalence and distribution of reactive species generated in the plasma [42]. Figure 8 shows the calculated mean electron energy as a function of the reduced electric field. Clearly, the mean electron energy increases with the increase of the reduced electric field in the air DBD. The coloured rectangle illustrates the range of the reduced electric field in this study, between 49.1 Td for plasma only and 74.2 Td for BaTiO3, while the DBD packed with Al2O3 has an E/N of around 60 Td.
Figure 8. Calculated mean electron energy as a function of the reduced electric field.
Download figure:
Standard image High-resolution imageFigure 9 shows the influence of packing materials on the calculated mean electron energy at different discharge powers. Referring to the trend in Figure 8, increasing the discharge power enhanced the reduced electric field and mean electron energy. The mean electron energy of the DBD packed with Al2O3 or BaTiO3 was higher compared to the discharge using plasma only. The DBD with BaTiO3 packing showed the highest mean electron energy of 1.81 eV at a discharge power of 16 W. More importantly, our calculation implies the presence of packing materials significantly changes the physical properties of the discharge, generating more energetic electrons benefitting the activation of dinitrogen, which is consistent with the improved performance of plasma N2 fixation (see section 3.3).
Figure 9. Calculated mean electron energy as a function of discharge power.
Download figure:
Standard image High-resolution image3.3. Effect of packing materials on the NOx synthesis
In this study, NO and NO2 were identified as the major products in the plasma synthesis of NOx , while N2O was also detected. Figure 10 shows the influence of packing materials on the NOx production at different discharge powers. Clearly, increasing discharge power enhanced the NOx concentration. It is noteworthy that increasing discharge power from 9 to 16 W almost doubled the NOx concentration from 5811 to 11 830 ppm using BaTiO3 packing. Increasing discharge power can generate a greater number of filaments, creating more channels for plasma chemical reactions, thus enhancing the production of NOx [43]. Patil et al also reported NOx concentration increased significantly when increasing the discharge power in a DBD reactor [12].
Figure 10. Effect of packing materials on NOx concentration at different discharge powers.
Download figure:
Standard image High-resolution imageCompared with the reaction using plasma only or Al2O3, packing BaTiO3 in the DBD significantly enhanced the formation of NOx , which can be attributed to the enhanced reduced electric field and mean electron energy induced by the packing, generating more energetic electrons and chemically reactive species (e.g. nitrogen and oxygen excited states) for the activation of N2 molecules. The positive effect of BaTiO3 packing on the plasma chemical reactions was also reported in previous studies. For instance, Michielsen et al evaluated the effect of different packing materials (e.g. glass wool, quartz wool, SiO2, ZrO2, Al2O3 and BaTiO3) on CO2 dissociation in a packed-bed DBD reactor and found that the BaTiO3 packing exhibited the highest CO2 conversion and energy efficiency [44]. Mei et al also reported that packing BaTiO3 into a DBD reactor substantially enhanced the conversion of CO2 and energy efficiency compared to the plasma reaction without packing [45].
Figure 11 illustrates the selectivity of NO, NO2, and N2O as a function of discharge power with and without packing materials. Increasing the discharge power enhanced the NO selectivity but decreased the selectivity of NO2 and N2O regardless of whether the packing is used or not. NO and NO2 were the dominant products for the reaction using plasma only or Al2O3 packing, while packing BaTiO3 into the DBD substantially increased the NO selectivity, reaching a maximum of 67.9% at a discharge power of 16 W. This finding indicates that the stronger electric field induced by BaTiO3 packing is favourable for the formation of NO, which can be explained by the NO formation pathways below.
Figure 11. Effect of discharge power on the selectivity of different NOx products: (a) plasma only; (b) packed with Al2O3; (c) packed with BaTiO3.
Download figure:
Standard image High-resolution imageIn the NOx synthesis from N2 and O2, the primary step is the formation of NO, as shown in (R1).

However, this direct bimolecular reaction is symmetry-forbidden. It is well recognised that the NO molecules are generated through a combination of the elementary reactions (R2) and (R3) following the Zeldovich mechanism. The first reaction (R2) is typically regarded as the rate-determining step [46].


In NTPs, collisions between the plasma-induced energetic electrons and neutral particles can generate numerous highly reactive species that can participate in the synthesis of NOx . As a result, NOx synthesis reactions that only occur at combustion temperatures can be achieved at low temperatures by overcoming the high energy barrier of NO formation. To be more specific, the rate-limiting reaction (R2) can be significantly accelerated by the plasma-generated nitrogen excited species, as shown in (R4).

Besides, the enhancement of the electric field and mean electron energy could effectively facilitate the vibrational and electronical excitation of nitrogen molecules, as proved by the enhanced rate coefficients calculated by BOLSIG+ (Figure 12), which explains the improved NO selectivity with the increase of discharge power in the presence of the BaTiO3 packing.
Figure 12. The rate coefficients of different excitation channels of N2 molecules as a function of the mean electron energy (the coloured area illustrates the range of the mean electron energy in this study).
Download figure:
Standard image High-resolution imagePrevious studies of plasma NOx synthesis [19, 47, 48] showed that reaction (R5) is the dominant pathway for NO2 formation in NTPs, while the generated NO2 molecules may be converted back to NO via reaction (R6). (M: third body)


The formation of N2O can be mainly attributed to the reactions (R7)–(R9) [18].



As the initial energy of ground-state molecules can be elevated by plasma-generated chemically reactive species, the energy barriers of the aforementioned reactions can therefore be effectively reduced.
Figure 13 shows the effect of packing materials on the energy consumption of NOx synthesis at a constant discharge power of 16 W. Note the overall energy consumption was almost constant when increasing the discharge power. The NOx synthesis using plasma only exhibited the highest energy consumption of 51.3 MJ mol−1, significantly higher than when using Al2O3 packing (33.4 MJ mol−1) and BaTiO3 packing (15.9 MJ mol−1). This result indicates that the energy cost of the plasma NOx synthesis using DBDs can be significantly reduced by using packing materials. More importantly, introducing proper packing materials into the plasma region can benefit the NOx generation from both physical and chemical standpoints.
Figure 13. The energy consumption of NOx synthesis with and without different packing materials (discharge power 16 W).
Download figure:
Standard image High-resolution imageTable 3 compares the reaction performances of the NOx synthesis using DBD plasmas. The concentration of NOx produced in this study is higher than that reported in previous works using the same type of NTP. Without packing materials, the energy consumption of NOx synthesis is comparable or higher than that using similar DBD systems, while the energy consumption (15.9 MJ mol−1) for NOx synthesis using BaTiO3 is lower compared with the previous studies using packed-bed DBDs.
Table 3. A comparison of NOx synthesis using different DBD plasmas.
Plasma reactor | Reactants | NOx concentration (ppm) | SEI (J L−1) | Energy consumption | Reference |
(MJ mol−1) | |||||
DBD | N2 (94%) O2 (6%) | 290 | 3000 | 232 | [17] |
DBD packed with MnOx /Al2O3 | N2 (94%) O2 (6%) | 170 | 1700 | 224 | [18] |
DBD packed with Al2O3 | N2 (50%) O2 (50%) | 5000 | 4300 | 18 | [12] |
Surface DBD | Air | 6000 | — | 56 | [13] |
DBD | Air | 3850 | 9600 | 51 | This work |
DBD packed with Al2O3 | Air | 5790 | 9600 | 33 | This work |
DBD packed with BaTiO3 | Air | 11830 | 9600 | 16 | This work |
4. Conclusion
In this study, a coaxial packed-bed DBD reactor was developed for the synthesis of NOx from air. Packing Al2O3 or BaTiO3 beads in the DBD reactor significantly affected the discharge behaviours in comparison with no packing. The DBD packed with BaTiO3 showed a higher average electric field and mean electron energy compared to the Al2O3 packing, which is likely attributed to the greater capacity of BaTiO3 to trap charges. Increasing the discharge power or introducing packing materials into the plasma region greatly enhanced the formation of nitrogen oxides. The production of NOx was strongly correlated to the reduced electric field and mean electron energy of the DBD. The presence of packing materials (especially BaTiO3 beads) can generate more energetic electrons that facilitate the formation of chemically reactive species including excited N2 molecules, thus effectively accelerating the synthesis of nitrogen oxides. Furthermore, the BaTiO3-packed DBD system achieved a low energy consumption of 15.9 MJ mol−1, indicating the energy consumption for NOx production using DBDs can be significantly reduced by introducing proper packing materials into the plasma region.
Acknowledgments
The support of this work by the UK EPSRC Impact Acceleration Account (IAA) is gratefully acknowledged. Y Ma also acknowledges the PhD fellowship co-funded by the University of Liverpool and the Chinese Scholarship Council (CSC). Y Wang acknowledges the European Union (EU) and Horizon 2020 funding awarded under the Marie Sklodowska-Curie Action to the EUROPAH Consortium (Grant No. 722346).
Data availability statement
The data that support the findings of this study are available upon reasonable request from the authors.