Abstract
We present a spatio-temporally resolved analysis of electron power absorption in capacitively coupled argon plasmas at low pressures (1–10 Pa), based on the 1D momentum balance equation embedded into 1d3v particle-in-cell/Monte Carlo collisions simulations. In contrast to the predictions of theoretical models we find 'Ohmic heating' to be the dominant electron power absorption mechanism on time average at the lowest pressures, and not 'stochastic' or 'Pressure heating'. The cause for this is identified to be the attenuation of electron power absorption due to electron acceleration by the 'ambipolar' electric field on time average at low pressure, which is a consequence of the collisionless transit of energetic beam electrons generated during sheath expansion at one electrode to the opposite electrode. At such conditions, these energetic electrons arrive during the local sheath collapse and can be lost to the surface, thereby reducing the plasma density and creating a temporally more symmetric electron temperature within the radio frequency (RF) period compared to that in discharges operated at higher pressures. The more symmetric temperature profile causes a reduction of 'Pressure heating' on time average. The latter is reduced further, even to negative values, by the attenuation of the 'ambipolar' electric field at each electrode during the local sheath collapse, which is a consequence of the temporal modulation of the electron density profile within the RF period, observed at the lowest pressures studied.
Export citation and abstract BibTeX RIS

Content from this work may be used under the terms of the Creative Commons Attribution 4.0 licence. Any further distribution of this work must maintain attribution to the author(s) and the title of the work, journal citation and DOI.
1. Introduction
Low temperature capacitively coupled radio frequency (RF) plasma sources (CCPs) are indispensable tools for numerous applications such as etching, deposition, surface microstructuring or functionalization in microelectronics or medicine [1–3]. The optimisation and control of these plasma processes is immensely important and requires a thorough understanding of the physical mechanisms present in such plasmas. Owing to the complexity of these mechanisms, our understanding of low temperature plasmas and CCP discharges in particular, is still far from complete. One of the most important issues is the electron power absorption mechanism, i.e. the way in which energy is transferred from the electric field to, and then redistributed among, the electrons, since mostly energetic electrons generate the plasma via ionization and generate process relevant particle species such as reactive radicals and ions. Electron power absorption has also been called 'electron heating' historically, but in fact there are important differences between both mechanisms: while electron acceleration in one direction yields power absorption, it does not yield heating, since heating requires an increase of the electron temperature, i.e. an isotropization [4, 5]. Nevertheless, for simplicity and brevity, occasionally we will use the historic terminology of 'heating' or 'cooling' to describe the different electron power absorption mechanisms. Along the same line and in order to stick to the 'traditional' terminology, which the reader is most likely familiar with, we will use 'inertial/pressure/Ohmic heating' instead of the more precise wording 'power absorption term related to the inertial/pressure/Ohmic field'. Similarly, 'sheath width' (according to Brinkmann's criterion) is used throughout the text, although 'electron edge' would be a more precise, physically descriptive terminology [6].
There has been ongoing research during the last 50 years aimed at understanding the electron power absorption dynamics in radio frequency discharges. A nonzero energy deposition on time average from a periodically varying electric field can only exist, if the phase coherence between the electron motion and the electric field is broken. As collisions can achieve this, the first electron power absorption mechanism described by a model was called 'Ohmic heating' (also called 'collisional heating'), which incorporates collisions between electrons and atoms/molecules from the background gas. The model consists of a simplified version of the momentum balance equation (i.e. the first velocity moment of the Boltzmann equation), where gradients are neglected. For the collisional electron momentum loss a highly simplified version is used and a harmonic time dependence of the electric field is assumed [1–3]. As the predictions of this model could not fully account for the experimentally measured total power absorbed by electrons at low pressure, Godyak et al introduced an additional type of electron power absorption mechanism, called 'collisionless heating' [7, 8]. One model, aiming to provide a theoretical description of 'collisionless (or stochastic) heating', is called the hard wall model [9–12]. It assumes that the electron density is zero within the sheath and the electric field is zero outside the sheath. Therefore, electrons can only gain energy by interacting with the instantaneous sheath edge (hence the name). Furthermore, the model only treats a single sheath and assumes an input electron energy distribution function. The total electron power absorption is then calculated as the sum of the 'collisional/Ohmic' and the 'collisionless heating'. As the two models are conceptually separate, adding their results leads to an inconsistent description of electron power absorption (although, in some cases, experiments agree with the models' predictions [7, 8]). Furthermore, it has been shown for electropositive single frequency low pressure CCPs that the above assumptions are mostly invalid [4, 13].
Surendra and Dalvie were the first to use a self-consistent analysis of electron power absorption in an electropositive single-frequency low pressure CCP using the Boltzmann equation aided by particle-in-cell (PIC) simulations [14]. They found that there is a considerable contribution to 'collisionless heating' from the pressure gradient, which is originally neglected in both models described above, and a space- and time dependence of the mean electron energy is required in order to get a nonzero 'collisionless heating' on time average. Based on similar considerations, Turner and Gozadinos developed the theory of 'Pressure heating' in CCPs [15, 16]. Lafleur et al revisited electron power absorption making use of the self-consistent analysis of Surendra and Dalvie [17]. They found that most of the time only 'Ohmic heating' and 'Pressure heating' are significant for the total electron power absorption, although, under certain conditions, inertial power absorption can also have a nonzero contribution on time average. It should be noted, that 'stochastic heating' originating from the Hard Wall Model does not exist in this framework.
During the investigation of electron power absorption in low pressure CCPs, new phenomena were observed, and new models have been proposed, related e.g. to resonance phenomena, such as 'Nonlinear Electron Resonance Heating' (NERH) [18–23] and 'Bounce Resonance Heating' [24–26], as well as to the importance of plasma–surface interactions. Different electron power absorption modes (or discharge operation modes) have been introduced, e.g. the α-, γ-, drift-ambipolar and striation modes [27–40].
Grapperhaus and Kushner developed a semianalytic RF sheath model to describe electron power absorption in CCPs [41]. Brinkmann derived a unified theoretical description of electron power absorption in CCPs [42, 43]. He showed that the total time averaged electron power absorption is the sum of four terms, each of which corresponds to a separate model of electron power absorption known previously, i.e. NERH (where the sheath collapse excites the plasma series resonance, which leads to an oscillating current, j(t), during sheath expansion and to dissipation via a high value of j(t)2), 'stochastic heating' (related to the Hard Wall Model), 'ambipolar/Pressure heating', and 'Ohmic heating'. Furthermore, it was also demonstrated that a time dependence of the electron temperature is needed to obtain a nonzero 'collisionless electron heating' on time average. This requirement, as we will see, has very important consequences on the nature of electron power absorption in CCPs operated at low pressures.
Schulze et al performed a space- and time-resolved analysis of electron power absorption dynamics in electropositive single frequency argon CCPs with a relatively large electrode gap of 5 cm based on the first two velocity moment equations of the Boltzmann equation (i.e. the continuity and momentum balance equations) [4]. They showed that there is a high electric field outside the sheath, which mainly originates from a term, which, in quasineutral regions, is the same as the 'classical ambipolar' electric field, denoted by , where T∥ is the (parallel) electron temperature, n the electron number density, and e is the electron charge [13]. It was found that the space and time averaged Ohmic power absorption is the dominant term at high pressures and it monotonically decreases with decreasing pressure. The other significant power absorption term, P∇p
, which is called 'Pressure heating', was found to be nearly constant throughout the whole pressure range, thus being the dominant power absorption mechanism at low pressure. They provided a theoretical explanation why a temporally constant electron temperature would yield zero power absorption for the term of the momentum balance equation associated with the pressure gradient, P∇p
= je ⋅ E∇p
. Here, je is the electron conduction current density and E∇p
is the electric field obtained from the pressure gradient term of the momentum balance equation [13]. E∇p
is the sum of two terms, one being proportional to the electron temperature gradient,
, the other to the electron density gradient,
(i.e. the 'ambipolar field'). Without a spatial dependence of the electron temperature, P∇T
= je ⋅ E∇T
would yield exactly zero. Without a temporal change of the electron temperature within each RF period, P∇n
= je ⋅ E∇n
would yield zero power absorption on time average. This can be understood as follows: as argon is an electropositive gas, the plasma density is a monotonically increasing function of the distance from the electrode up to the middle of the bulk region. In regions of quasineutrality outside the sheaths the electron density is equal to the ion density and, thus, there is no temporal modulation of the plasma density and its gradient, if the ion density is not time modulated. Thus, the plasma density and its gradient will be the same during sheath expansion as well as sheath collapse. As the electron current density changes its sign between these two sheath phases, unless there is a temporal asymmetry in E∇n
, P∇n
has to be zero on time average. The temporal asymmetry will usually be present for the following reason: as E∇n
∝ T∥, the electrons initially accelerated by E∇n
during sheath expansion, will acquire a higher energy, which amounts to an increase of the electron temperature. But this also leads to an increase of the magnitude of E∇n
, which will then accelerate the electrons even more. This self-amplifying mechanism lasts until the sheath is fully expanded. The same mechanism is not present during sheath collapse, as in this case cold electrons stream into the sheath region, which are even decelerated by E∇n
, thus leading to a low electron temperature region close to each electrode during sheath collapse. Thus, a temporal asymmetry within each RF period arises in the electron temperature, which leads to a nonzero value for P∇n
on time average.
Following this work, Vass et al investigated the electron power absorption dynamics in electronegative single-frequency oxygen discharges [5]. They found, that due to the increasing electronegativity of oxygen at low pressure, P∇p
is attenuated and the Ohmic power absorption is the dominant power absorption mechanism on space and time average. The reason for this is twofold: firstly, one consequence of the high electronegativity of oxygen is, that the density of the negative ions will increase, which, in order to ensure quasineutrality, leads to a decrease of the electron density within the bulk. This, in turn, will lead to a decrease of the electron conductivity (as , where ωpe is the electron plasma frequency). Therefore, there is a higher resistivity and consequently a higher Ohmic power absorption. Secondly, due to the depleted electron density inside the bulk, the spatial profile of the electron density will change; namely, an 'electropositive' edge region will form, where the electron number density has local maxima. From the electrode up to this point, the electron density monotonically increases, then it monotonically decreases from this point up to the middle of the bulk, i.e. the spatial gradient of the electron density will have opposite signs at the two sides of the electropositive edge region. This will reduce the above explained temporal asymmetry in the electron temperature, thus leading to an attenuated P∇p
. The reason for this is, that during sheath expansion the electrons are first accelerated by E∇n
, then, after reaching the electropositive edge region, are decelerated, as the gradient of the electron density changes its sign. Furthermore, the same electric field will accelerate incoming electrons during the phase of sheath collapse, thus leading to a temporally more symmetric electron temperature and, thus, to an attenuation of P∇p
.
Using the same analysis, Zheng et al investigated magnetized CCPs and found that, by increasing the magnetic field, the Ohmic power absorption caused by the Hall current, will completely dominate over the electron power absorption term associated with the pressure gradient, even at low pressure [44].
In this paper we demonstrate, that—contrary to common belief and theoretical models—there are conditions even in electropositive single-frequency argon CCPs, where Ohmic power absorption is the dominant power absorption term at low pressure. Although Vahedi et al [45] simulated the conditions of Godyak et al [8] to show that 'collisionless/stochastic heating' is the apparent dominant power absorption mechanism at low pressure, they did not perform or show a detailed power absorption analysis. Instead, they infer this behaviour at low pressure simply from a transition in the shape of the EEDF. We show, that for Ohmic power absorption to become dominant at low pressure, the driving frequency and electrode gap must be chosen in a way that ensures that energetic beam electrons generated at one electrode during sheath expansion reach the opposite electrode during the local sheath collapse with a high energy. The plasma is operated at the edge of existence at very low plasma densities. In this mode of discharge operation, the plasma sheaths expand very rapidly on timescales shorter than the local electron plasma frequency. This causes the self-excitation of resonance phenomena and the generation of multiple 'electron beams' per sheath expansion phase at each electrode [22], one of which hits the opposing electrode during the local sheath collapse and consequently leads to a drastic decrease in the electron number density [21]. By decreasing the pressure, energetic electrons generated at one electrode are more likely to be able to reach the opposite electrode without suffering any collisions with the gas atoms. This results in a temporally more symmetric electron temperature distribution, which, as a consequence, leads to the attenuation of 'ambipolar' power absorption and thus 'Pressure heating' on time average. As long as the axial electron density profile is stationary and characterized by a peak in the discharge centre, energetic beam electrons are decelerated by a local 'ambipolar' electric field upon arrival at the opposite sheath. This ensures some degree of temporal asymmetry of the electron temperature within the RF period and, thus, a positive 'Pressure heating' on space and time average. By lowering the pressure, however, a point can be reached, where 'Pressure heating' starts to be negative. This regime is characterised by a time-modulated electron density, so that the classical plasma bulk, where the electron density has a maximum at a fixed axial position, vanishes. Instead, the electron density maximum oscillates back and forth between the electrodes within each RF period. This is found to happen, if the electron mean free path is comparable or larger to the electrode gap and if the maximum sheath width gets similar to half the electrode gap. In this case the electron density maximum moves towards the electrode, where the sheath is collapsing, jointly with the 'electron beams', their deceleration by the 'ambipolar' field at the opposite electrode is attenuated significantly compared to discharge conditions present at higher pressures, where a stationary plasma bulk is present. This is caused by a decrease of the absolute value of the 'ambipolar' electric field around the position of maximum sheath width during sheath collapse at each electrode due to the axial shift of the electron density maximum which leads to a highly symmetric electron temperature in time, which results in an attenuated power absorption from the 'ambipolar' field. As P∇T , the other term in 'Pressure heating' is always negative, this overall results in negative 'Pressure heating'. 'Ohmic heating', on the other hand, is significant due to the low conductivity in the low pressure regime, because of the low plasma density. We perform a detailed spatio-temporal analysis of such discharge conditions based on 1d3v particle-in-cell/Monte Carlo collisions (PIC/MCC) simulations and a model based on the first velocity moment of the Boltzmann equation to understand the electron power absorption dynamics in detail.
The paper is structured as follows: in section 2, the theoretical background of the spatio-temporal analysis of electron power absorption is briefly introduced alongside with the PIC/MCC simulational method from which the input parameters for this analysis are obtained. In section 3, results are presented and discussed. Finally, in section 4 conclusions are drawn.
2. Theoretical background and computational method
The details of the analysis applied here have been described in [4] and its recently revised form in [5]. Therefore, we only provide the most important conceptual aspects.
The basis of this spatio-temporally resolved analysis of the electron power absorption is formed by the first velocity moment of the 1D Boltzmann equation (i.e. the momentum balance equation), which can be rearranged for the total electric field, that consists of three terms, i.e. Etot = Ein + E∇p + EOhm [5]:
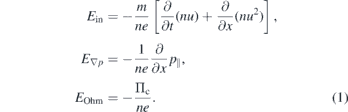
Here n and u are the electron density and mean velocity, respectively, m is the electron mass and e the electron charge. p∥ denotes the diagonal element of the electron pressure tensor. Here, 'parallel' refers to the direction of the electric field, which is perpendicular to the electrode surfaces. Πc incorporates the change of electron momentum due to collisions.
Each term in equation (1) originates from a distinct physical mechanism. The inertia term, Ein, is the electric field needed to balance the change in electron momentum. EOhm is a result of collisions. E∇p , which is the electric field originating from the pressure gradient (for easier readability, from now on, referred to as 'Pressure heating'), is commonly split into two separate parts (E∇p = E∇n + E∇T ), which have the following forms:

Here T∥ denotes the parallel electron temperature (in units of eV), which is defined as the ratio of the parallel electron pressure and the electron density, T∥ = p∥/n. This relation resembles the ideal gas law. Note, however, that T∥ is different from the 'classical electron temperature', Te; with T⊥ as the perpendicular temperature, the relation is . E∇n
is, in quasineutral regions, identical to the 'classical ambipolar' electric field (see e.g. [13]). E∇T
originates from the gradient of the electron temperature.
Based on this, the power density absorbed by the electrons can be calculated for each term and, consequently, for the total electric field by simply multiplying the terms with the electron conduction current density, je.
Although the above framework consists of no a priori assumptions and in this sense describes every physical mechanism of electron power absorption, n, u, p∥, T∥, Πc, and je must be known as input parameters. These quantities are taken from self-consistent 1d3v PIC/MCC simulations and the electric field and power absorption terms are calculated using these data. The simulation code used for the analysis was thoroughly described in [4, 46]. Therefore, here only the most important aspects are summarized. For more details the reader is referred to e.g. [4].
In the simulations, plane and parallel electrodes with a gap of L = 25 mm are assumed. The operating gas is argon. One of the electrodes is driven by a voltage waveform ϕ = ϕ0 cos(2πft), where f = 27.12 MHz and ϕ0 = 500 V, while the other electrode is at ground potential. During the simulations, no secondary electrons are considered and the reflection probability of electrons reaching the electrodes is 20% [47]. The gas temperature is Tg = 350 K. The neutral gas pressure is varied from 1 Pa to 10 Pa. The species traced are positive argon ions (Ar+) and electrons. For the electrons elastic collisions, excitation and ionization are considered [48]. For the ion-atom collisions, two processes are considered: isotropic scattering and backward scattering (corresponding to charge exchange) [48]. The computations are carried out using a spatial grid with Nx = 1200 points and Nt = 20 000 time steps within the RF period. We use ∼105 particles for each species (i.e. Ar+ and e−) and average over ∼104 RF-cycles after full convergence to obtain high precision data. These discharge conditions are chosen to ensure the presence of large sheaths and a situation, where energetic 'electron beams' generated during sheath expansion at one electrode reach the opposite electrode during the local sheath collapse at the low pressure end of the pressure interval investigated here. Simulations are also performed at higher pressures to illustrate the transition from the non-local and non-linear discharge mode of interest at low pressures, where 'Ohmic heating' is dominant, to the more conventional and previously studied low pressure mode, where 'Pressure heating' is dominant.
3. Results
In the following, results are presented for a pressure range between 1 and 10 Pa, for a driving voltage amplitude of ϕ0 = 500 V, and a frequency f = 27.12 MHz.
Figure 1 shows the space- and time-averaged electron power density associated with each electric field term introduced in equation (1), as well as the total electron power density as a function of pressure. The averaging is performed as , where i is an index that refers to the respective electric field term. The sum of all individual power absorption terms yields the total power absorbed by electrons (as obtained directly from the simulation) at every position and time within the RF period. The vertical black dashed line indicates the pressure at which a mode transition happens, i.e. below/above this pressure 'Pressure heating' is negative/positive on space and time average. Similarly, the red dotted line indicates the pressure below which 'Ohmic heating' becomes dominant over 'Pressure heating'. According to figure 1 the inertial power absorption, Pin, is negligible on space and time average under all discharge conditions studied here. The 'Pressure heating' term, P∇p
, is the most significant one above 2 Pa, in accordance with previous results obtained in [4]. One of the main observations of [4] was, that by decreasing the pressure, the most significant power absorption term (and thereby essential to sustaining the plasma at low pressure), is P∇p
. As seen in figure 1, this is not the case under the discharge conditions considered here: below 2 Pa, P∇p
decreases monotonically, acquiring a negative value below 1.1 Pa. Instead, the dominant power absorption term at low pressure (i.e. below 2 Pa) in this case is the Ohmic power absorption, POhm, which acquires a nearly constant, nonzero value between 1 and 2 Pa. The fact, that Ohmic power absorption, i.e. the power absorption due to collisions, is the most dominant term at low pressure in an inert electropositive gas, such as argon, is a counterintuitive result, since one might expect this type of power absorption to be reduced by decreasing the pressure, as the probability of collisions decreases.
Figure 1. Space- and time-averaged electron power density corresponding to each electric field term as a function of pressure. The vertical black dashed line at p ≈ 1.2 Pa indicates the pressure below/above which 'Pressure heating' is negative/positive on space- and time-average, while the red dotted line at p ≈ 1.8 Pa indicates the pressure below which 'Ohmic heating' becomes dominant on space- and time-average. L = 25 mm, ϕ0 = 500 V, f = 27.12 MHz.
Download figure:
Standard image High-resolution imageTo understand the underlying physical mechanisms leading to these results, plasma properties at two particular pressure values (1 and 2 Pa) will be discussed and analysed. These pressures are chosen, since 1 Pa is the lowest pressure studied here and 2 Pa approximately corresponds to the pressure, at which a mode transition from dominant Ohmic power absorption to dominant 'Pressure heating' happens.
Figure 2 shows the spatial profiles of the time-averaged electron power density terms as well as of the total absorbed power for 2 Pa (a) and 1 Pa (b). The vertical dashed blue lines indicate the maximum sheath widths, smax. The sheath edges, s(t), have been calculated using Brinkmann's sheath criterion [6].
Figure 2. Spatial profiles of the time-averaged total electron power absorption, Ptot(x), and the contribution of each individual term at 2 Pa (a) and 1 Pa (b). The vertical dashed lines indicate the maximum sheath widths, smax. L = 25 mm, ϕ0 = 500 V, f = 27.12 MHz. The powered and grounded electrodes are situated at x = 0 mm and x = 25 mm, respectively.
Download figure:
Standard image High-resolution imageAt 2 Pa there are two significant maxima of the electron 'Pressure heating', P∇p , at each electrode on time average. One maximum is located inside the local sheath and the other one is located on the bulk side of the position corresponding to the maximum sheath width. In the plasma bulk, where the electron density is nearly constant, P∇n is low, P∇T is significant, but negative on time average, due to the formation of a double layer of charges around the position of the maximum sheath width (see later). Therefore, P∇p is negative on time average inside the bulk. The Ohmic power absorption, POhm, is positive throughout the whole spatial domain, exhibiting maxima within the sheath region. Finally, 'Pressure heating', P∇p , exhibits a negative value near the electrodes. This is caused by a 'reversed' temporal asymmetry of the electron temperature within each RF period in the vicinity of the electrode, which will be discussed in more detail later.
At 1 Pa (figure 2(b)) a different scenario compared to the situation at 2 Pa is observed: the maximum sheath widths are much larger, of the order of half the electrode gap, than in case of 2 Pa and, thus, the plasma bulk region is much smaller. The 'Pressure heating', P∇p , is even more negative at the electrode surface on time average because of the 'reversed' temporal asymmetry of T∥, which is more pronounced than in case of 2 Pa. The positive 'Pressure heating' further inside the plasma is attenuated strongly compared to the situation at 2 Pa. The Ohmic power absorption on the other hand, exhibits a maximum in the middle of the discharge, and is the most dominant term on time average throughout most of the spatial domain.
To understand the characteristics of 'Ohmic heating' in these two cases, one has to look at the spatio-temporal distribution of the electron conduction current densities, which is shown in figure 3 for 2 Pa (a) and 1 Pa (b).
Figure 3. Spatio-temporal distribution of the electron conduction current density, je, during one RF period at 2 Pa (a) and 1 Pa (b). The colorbar shows units of A m−2. The black lines indicate the sheath edges. L = 25 mm, ϕ0 = 500 V, f = 27.12 MHz.
Download figure:
Standard image High-resolution imageThe scenario at 2 Pa (figure 2(a)) can be understood as follows: as argon is an electropositive gas, the electron density increases monotonically as a function of the distance from the electrode. Consequently, the conductivity, σ, being proportional to the electron density, also increases, thus being higher within the bulk. This leads to a high 'Ohmic heating' within the sheath region, where the electron density is low, and an attenuation of this power absorption term within the bulk, where the electron density and, consequently, the conductivity is high.
At 1 Pa, the different characteristics of the profile of the time averaged 'Ohmic heating', i.e. its strong maximum in the middle of the discharge, can be explained based on two observations: (i) as seen in figure 3(b), the electron conduction current density, je, at 1 Pa has, due to the large sheath widths, its maximum value within the region of the narrow plasma bulk. Thus, there is a maximum of 'Ohmic heating' in the centre. (ii) As the plasma density is low at 1 Pa, the conductivity is also low, even at the position of maximum plasma density. Thus, 'Ohmic heating' is generally strong at 1 Pa.
This is further emphasized in figure 4, which shows the space- and time-averaged particle densities (red/blue points) as well as the ratio of the average electron and ion densities (black points) as a function of pressure. The average electron density changes from its value at 2 Pa (4 ⋅ 1015 m−3) to 1014 m−3 at 1 Pa, thus allowing for a lower conductivity and, therefore, a higher 'Ohmic heating' on time average.
Figure 4. Space- and time-averaged particle densities as well as the ratio between the average electron and ion density as a function of pressure. L = 25 mm, ϕ0 = 500 V, f = 27.12 MHz.
Download figure:
Standard image High-resolution imageThe drastic drop in the electron number density between 1 and 2 Pa is due to energetic 'electron beams' generated at one electrode hitting the other sheath during its collapse and thereby leading to an ineffective confinement of energetic electrons [20]. This can be seen in figure 5, which shows the ionization source function, Sion, for 2 Pa (a) and 1 Pa (b). Let us identify 'electron beams' (following [20]) as straight features that follow the maximum of the ionization rate, Sion. We note, that contrary to the traditional definition of a beam of particles, these electrons are not strictly monoenergetic. At both pressures, 1 Pa and 2 Pa, the mean free path of the energetic beam electrons is long enough to allow beam electrons generated at one electrode to reach the other electrode. According to this definition, at 2 Pa, only one 'electron beam' can be seen, which causes significant ionization within the bulk, and hits the opposing sheath at ≈12 ns. Within this feature a fine structure can be seen, that is a consequence of the higher local plasma frequency compared to the 1 Pa case. Under these conditions, energetic electrons are formed at a faster rate during sheath expansion, which can lead to several energetic electrons forming one 'broad' ionization maximum. At 1 Pa, a different scenario is observed: here, two distinct lines with ionization maxima can be seen (figure 5(b)). One of these 'beams' reaches the opposing sheath at ≈10 ns, the other at ≈18 ns. The latter hits the opposing electrode during sheath collapse, thus leading to the loss of numerous energetic electrons during one RF-cycle, resulting in a depleted electron density due to the less effective beam confinement [20]. The two distinct maxima are a consequence of the lower pressure and hence the lower local plasma frequency of the electrons, which leads to a situation where the time between the generation of two energetic 'electron beams' is high enough to be easily distinguished in the figure.
Figure 5. Spatio-temporal distribution of the ionization source function, Sion, during one RF period at 2 Pa (a) and 1 Pa (b). The white lines indicate the sheath edges. L = 25 mm, ϕ0 = 500 V, f = 27.12 MHz. The powered and grounded electrodes are situated at x = 0 mm and x = 25 mm, respectively.
Download figure:
Standard image High-resolution imageAlthough electron heating is a complex phenomenon (as already demonstrated above), we can gain additional insight into why a 'transition' at approximately 1.8 Pa occurs from a 'Pressure heating' regime to an 'Ohmic heating' regime, by considering a particle-based description. In this description, 'Pressure heating' is a 'hybrid' heating mechanism where 'nonlocal' collisions are needed to yield a nonzero power absorption on time average [51, 52]. Here, electron collisions with heavy particles are vital for breaking the phase coherence between the RF electric field and the electron velocity. In other words, energetic electrons generated at one sheath need to undergo collisions before reaching the opposite electrode, otherwise the electron temperature distribution will be temporally symmetric and thus, 'Pressure heating' will be attenuated. However, these collisions can occur at a different location in the discharge to that of the electron energy gain by the expanding sheath, and for this reason, have a 'nonlocal' character (and are distinctly different from 'local' collisions which lead to the usual 'Ohmic heating').
Let us consider the energetic electrons generated by the expanding sheath edge near one of the electrodes. The collision time of these energetic electrons, tcoll, which is the average time between consecutive collision events, can be approximated by , where ng is the neutral gas density, v is the speed of the electrons, and σm is the effective momentum transfer cross-section at the electron energy. Another important time scale is the electron transit time, ttransit, which is the time needed for the energetic electrons to traverse the 'bulk' plasma region and arrive at the opposite sheath edge. This can be found from
, where Lbulk is the distance between the instantaneous sheath edges the electron starts from and reaches, which is approximated in this case as Lbulk ≈ L − smax, with L the gap length, and smax the maximum sheath width. If the transit time is less than the collision time, the electrons will on average reach the opposite sheath edge without having a collision, and thus, it is reasonable to expect that the needed field-velocity phase randomization (or, equivalently, the temporally asymmetric electron temperature distribution) essential for a nonzero 'Pressure heating', will be less likely (as theoretically and numerically predicted previously [51, 52]). This would then lead to a decrease of the 'Pressure heating' term.
Figure 6(a) shows the ratio between the collision time, tcoll, and transit time, ttransit, of energetic electrons. The energy of the electrons is taken to be the ionization threshold, 15.7 eV (i.e. their velocity is ≈2.3 ⋅ 106 m s−1). The horizontal black dashed line indicates the point where these times are equal. The vertical blue dotted line indicates the pressure below which 'Ohmic heating' becomes the dominant power absorption term (cf figure 1). Under the current conditions, this transition happens very close to where the two times are equal, which is quite remarkable given the simplicity of the criterion used.
Figure 6. Ratio between the collision time of energetic electrons, tcoll, and their transit time, ttransit as a function of pressure for an electrode gap of L = 25 mm (a) and the ratio of the space- and time averaged 'Ohmic' and 'Pressure heating', POhm/P∇p , as a function of pLbulk. The horizontal black dashed lines in panel (a) and (b) indicate the points where the corresponding ratios are equal to one. In panel (b) the blue line results from a pressure variation (1–10 Pa) at a constant electrode gap of 25 mm, while the red line results from a gap variation (25–50 mm) at a constant pressure of 1 Pa. The vertical coloured lines (blue: constant gap size, red: constant pressure) indicate the point below which 'Ohmic heating' becomes dominant on space- and time-average. Datapoints, which correspond to a negative 'Pressure heating', have been left out from panel (b). ϕ0 = 500 V, f = 27.12 MHz.
Download figure:
Standard image High-resolution imageFigure 6(b) shows the ratio of the space- and time averaged 'Ohmic' and 'Pressure heating', i.e. POhm/P∇p as a function of pLbulk. Again, the vertical dashed lines indicate the transition from dominant 'Ohmic heating' to dominant 'Pressure heating'. The blue line indicates the transition at a fixed gap length of L = 25 mm, with the transition point of ≈34.5 Pa mm. Similarly, the red line indicates the transition at a fixed pressure of p = 1 Pa, with a transition point of ≈25 Pa mm.
Based on the expressions for tcoll and ttransit provided above, the ideal gas law, and the proposed criterion for the heating mode transition, tcoll/ttransit = 1, we can obtain an approximate criterion for when 'Ohmic heating' will be dominant:

where p is the neutral gas pressure. For Tg = 350 K, and σm ≈ 1.5 ⋅ 10−19 m2, this criterion predicts a heating mode transition at a point of ≈32.2 Pa mm. This lies between the two transition points in figure 6(b). The proximity of these transition points based on the simulation results and that predicted by the simple model suggests that the latter captures correctly the underlying physics despite its simplicity. Therefore, our findings do not represent a special case, but are of general relevance, and are expected to occur whenever the parameter pLbulk is sufficiently low.
The ineffective confinement of energetic electrons and, thus, the low density lead to a significant increase of the maximum sheath width at each electrode. Figure 7 shows the ratio between the maximum sheath width, smax, and the gap length, L. The vertical dashed line indicates the transition point, where 'Pressure heating' goes negative as one moves below this point.
Figure 7. Ratio between the maximum sheath width, smax, and the gap length, L as a function of pressure. The vertical dashed line indicates the pressure below/above 'Pressure heating' is negative/positive on space- and time-average. L = 25 mm, ϕ0 = 500 V, f = 27.12 MHz.
Download figure:
Standard image High-resolution imageAt 1 Pa the maximum sheath width is almost as large as half the electrode gap. This leads to the extinction of the classical plasma bulk, characterized by a stationary position of the maximum of the electron density in the centre. As shown in figure 8(b) and (d), at 2 Pa the axial position of the electron density maximum is still constant, but at 1 Pa it is not. Instead it oscillates back and forth between the electrodes within the RF period, jointly with the movement of the energetic beam electrons. Actually, the transition between a stationary position of the electron density maximum (classical plasma bulk) and this oscillation happens at a pressure of about 1.2 Pa, at which smax/L crosses a threshold value of 0.35, under the discharge conditions studied here. According to figure 1 this coincides with the pressure at which 'Pressure heating' is zero.
Figure 8. Spatio-temporal distribution of the electron temperature during one RF-cycle at 2 Pa (a) and 1 Pa (c), as well as the normalized electron density gradient, ∇ne/ne, at 2 Pa (b) and 1 Pa (d). The solid black lines indicate the sheath edges, the dashed black line indicates the position of the maximum of ne. L = 25 mm, ϕ0 = 500 V, f = 27.12 MHz. The powered and grounded electrodes are situated at x = 0 mm and x = 25 mm, respectively.
Download figure:
Standard image High-resolution imageThe oscillation of the position of the maximum of the electron density within each RF period is the key to understand why 'Pressure heating' vanishes and even gets negative on space and time average at low pressures according to figure 1. At 2 Pa the power absorption due to 'Pressure heating' is positive on space- and time-average (see figure 1). As noted earlier, P∇p
consists of two separate terms, P∇n
and P∇T
, where the latter results in 'cooling'. Consequently, E∇n
needs to be temporally asymmetric in the electron temperature (T∥) and/or the normalized electron density gradient () to yield a positive 'Pressure heating' on space and time average. As the 'ambipolar' electric field, E∇n
, is proportional to the gradient of the electron density, its sign does not change during the whole RF-cycle at a given electrode. As the electron current changes sign between sheath expansion and collapse the 'ambipolar' power absorption has to be zero on time average unless there is a temporal asymmetry in E∇n
[4].
Figure 8 shows the spatio-temporal distribution of the electron temperature at 2 Pa (a) and 1 Pa (c), as well as the normalized electron density gradient at 2 Pa (b) and 1 Pa (d). At 2 Pa, the electron temperature exhibits a pronounced temporal asymmetry around the position of maximum sheath width, i.e. there is a strong difference between the local values of T∥ during sheath expansion and collapse at each electrode. This is the consequence of a self-amplifying mechanism [4]: the electrons accelerated by the 'ambipolar' field during sheath expansion will have a higher energy, which leads to a higher electron temperature. As E∇n ∝ T∥, this will increase the magnitude of the 'ambipolar' electric field during sheath expansion, which can accelerate electrons even more. This mechanism stops when the sheath is fully expanded. During sheath collapse, the same mechanism does not work, as the 'ambipolar' field, which has the same sign at a given electrode throughout the whole RF-cycle, will decelerate electrons coming from the other electrode. In the presence of a large electron mean free path, the reason why there is a temporal asymmetry in the electron temperature at 2 Pa, and therefore, a nonzero 'ambipolar' power absorption, is the fact, that the 'ambipolar' field has a maximum at the position of the maximum sheath width, which can be seen in figure 8(b), in the normalized electron density gradient. This leads to a deceleration of incoming beam electrons around the position of maximum sheath width during the local sheath collapse and, thus, to a minimum of the electron temperature at this position during the local sheath collapse. This temporal asymmetry in the electron temperature will lead to a nonzero 'ambipolar' power absorption on time average.
At this stage, one can also understand why in figure 2(a) 'Pressure heating' has a negative contribution in the vicinity of the electrodes on time average: although the energetic electrons, which reach the opposing electrode, will be decelerated by the 'ambipolar' field, they do reach the electrode. As in this region the electron density is low, these electrons can effectively increase the local electron temperature. This leads to a 'reversed' temporal asymmetry of the electron temperature within each RF period in the vicinity of the electrode, i.e. at the electrode surface the electron temperature is higher during sheath collapse compared to the sheath expansion phase. Thus, as the temporal asymmetry is reversed, so is the sign of the 'ambipolar' power absorption, which will, therefore, acquire a negative value.
At 1 Pa the electron temperature shows a different behaviour (figure 8(b)): due to the fact, that the elongated sheaths result in a 'vanishing' classical bulk region, the spatio-temporal structure of the 'ambipolar' field changes compared to the 2 Pa case. This can be seen in the normalized electron density gradient, figure 8(d): as the sheath widths are so long, that the 'classical' bulk vanishes, the maximum of the electron density will be time-modulated, i.e. it will acquire its maximum at different spatial positions at different times during the RF-period. This is indicated by a dashed line in this plot. Due to the time modulation of the electron density, and, thus, the electron density gradient, the corresponding 'ambipolar' field will have a spatio-temporal structure, which does not lead to an efficient deceleration of electrons during sheath collapse, as the maximum of the 'ambipolar' field, which was at the spatial position of the maximum sheath widths (or at the edge of the bulk) at higher pressures, vanishes. This is caused by the fact that the electron density profile moves jointly with the 'electron beams' and, thus, their deceleration due to a steep density profile at the opposing electrode during the local sheath collapse is attenuated. As the electron density is highly depleted, the incoming energetic electrons can effectively increase the electron temperature at the opposite electrode during sheath collapse. During sheath expansion, the same self-amplifying mechanism works as described above, but the presence of energetic beam electrons generated at the opposite electrode during the local sheath collapse results in a temporally more symmetric electron temperature distribution, which leads to the attenuation of the 'ambipolar' power absorption on time average. Indeed, as shown in figure 1, at 1 Pa P∇p is negative on time average, which is due to the fact, that P∇n is attenuated and P∇T acquires a negative value on space and time average due to the 'cooling' effect described above.
In order to understand these observations and the reasons leading to the differences between 1 and 2 Pa in more detail, a complete spatio-temporal analysis of the electron power absorption is provided below, as described in section 2. Figures 9 and 10 show the spatio-temporal distributions of the electric field and the electron power absorption associated with the different terms according to equations (1) and (2) for 2 and 1 Pa, respectively.
Figure 9. Spatio-temporal distribution of the electric field terms (left) and the electron power density terms (right) during one RF period at 2 Pa. The black lines indicate the sheath edges. L = 25 mm, ϕ0 = 500 V, f = 27.12 MHz. The powered and grounded electrodes are situated at x = 0 mm and x = 25 mm, respectively.
Download figure:
Standard image High-resolution imageFigure 10. Spatio-temporal distribution of the electric field terms (left) and the electron power density terms (right) during one RF period at 1 Pa. The black lines indicate the sheath edges. L = 25 mm, ϕ0 = 500 V, f = 27.12 MHz. The powered and grounded electrodes are situated at x = 0 mm and x = 25 mm, respectively.
Download figure:
Standard image High-resolution imageFor a pressure of 2 Pa, figures 9(a) and (b) show the inertial electric field (Ein) and the corresponding electron power density (Pin), respectively. Both of them exhibit a complex spatio-temporal structure, which is a consequence of a highly oscillatory, non-local mode of the plasma, investigated previously by Wilczek et al [20, 21]. The 'stripes' present in Pin are of two types: plasma oscillations, which are vertical 'stripes', i.e. they do not show any spatial propagation (or, equivalently, their group velocity is zero), and electrostatic waves, which are 'stripes' that have a slope, i.e. they can propagate in space. Both of them are generated by energetic electrons during sheath expansion at a given electrode [49]. The number of these 'wave maxima' depends on the local plasma frequency of the electrons, ωpe. Electrons can react to perturbations on a timescale of the inverse local electron plasma frequency, . When an energetic 'electron beam' is generated, as it propagates through the bulk, it leaves a positive space charge behind, which will generate an electric field that attracts cold bulk electrons towards the expanding sheath edge, which will, in turn, result in the generation of another energetic 'electron beam', when they hit the expanding sheath edge. This mechanism can continue until the sheath is fully expanded, which happens on a timescale of f−1. Thus, the ratio of the two frequencies
yields the number of times an energetic 'electron beam' can be generated within an RF-cycle at both electrodes [50].
At 2 Pa, the characteristics of the other terms are similar to the results obtained in [4]: the 'ambipolar' electric field, E∇n
(figure 9(c)), exhibits two extrema, one at the position of the maximum sheath width, and another in the vicinity of the instantaneous sheath edge. Furthermore, at a given electrode, the 'ambipolar' electric field has the same sign during the whole RF-cycle, which is a consequence of the characteristics of the spatio-temporal distribution of the electron number density, ne. As argon is an electropositive gas, the electron number density will monotonically increase as a function of the distance from the electrodes, up to the discharge center irrespective of time. As , the electric field will always have the same sign at a given electrode, e.g. it acquires a negative value in the vicinity of the powered electrode. Based on this, one can understand why in figure 2(a) there are two maxima in P∇p
: they correspond to the two distinct maxima of E∇n
, which is a consequence of the spatio-temporal distribution of the electron density. For the corresponding electron power absorption term, P∇n
(figure 9(d)), this means that there will be a region of positive power absorption, where the electron current density and the 'ambipolar' field have the same sign, e.g. during sheath expansion at the powered electrode, and also a negative power absorption in the other half of the RF-cycle, as the electron current density changes sign between sheath expansion and collapse, but the electric field does not. Figure 9(e) shows the electric field term corresponding to the temperature gradient, E∇T
. It exhibits extrema at the positions of the maximum sheath widths, with opposite signs compared to the 'ambipolar' field (panel (c)). The reason for this is the generation of a double layer of charges with negative space charges at the bulk side. Electrons are accelerated by the 'ambipolar' electric field, thus leaving positive charges behind, which will lead to the formation of a charged double layer. This generates an electric field that decelerate electrons generated during sheath expansion, but accelerates electrons coming from the other electrode during sheath collapse. Therefore, in the figure of P∇T
(figure 9(f)), a region of negative power absorption is observed during sheath expansion at the position of the maximum sheath width, and, correspondingly, a positive power absorption during sheath collapse. The Ohmic electric field term, EOhm (figure 9(g)), which is a result of collisions, has a small magnitude compared to the other electric field terms, but it extends into the bulk region as well, where the electron density is higher. As Πc changes its sign as well as je between sheath expansion and collapse, POhm (figure 9(h)) yields a region of positive power absorption throughout the whole RF-cycle in the sheath region and also within the bulk.
Figure 10 shows the other case, 1 Pa. Figure 10(b), which shows the inertial electron power absorption, Pin, exhibits two positive maxima, (the red stripes) corresponding to the two 'electron beams' generated during sheath expansion, seen in figure 5(b). This is due to the lower electron density (see figure 4) and, consequently, the lower local electron plasma frequency, which means, that the time difference between two successive 'electron beams' will be larger than in case of 2 Pa. One of these energetic 'electron beams' hits the opposing sheath during its collapse, thus leading to the loss of numerous energetic electrons and, thus, to a lower electron density. This, in turn, leads to longer sheath widths, which will result in a scenario where the bulk region essentially vanishes, the sheath regions 'merge' together, and the axial position of the electron density maximum oscillates back and forth between the electrodes.
The characteristics of the 'ambipolar' field, E∇n (figure 10(c)) change compared to the 2 Pa case. The larger sheaths and, thus, the vanishing of the 'classical' bulk region as well as the time modulation of the electron density profile result in a temporally more symmetric 'ambipolar' power absorption (figure 10(d)). This is caused by the fact that energetic beam electrons generated at one electrode during sheath expansion are not decelerated efficiently, when they reach the opposite sheath during its collapse due to the absence of a steep local electron density profile upon arrival at the opposite sheath. The electric field proportional to the electron temperature gradient, E∇T , and the corresponding power absorption term (figures 10(e) and (f)) show complex features that are attributed to the spatio-temporal dynamics of the electron temperature. As in the case above, the Ohmic power absorption term, POhm (figure 10(h)), albeit smaller in magnitude compared to other terms, has a positive value over a wide spatio-temporal domain.
Overall, the above spatio-temporal analysis of electron power absorption in CCPs operated in argon at low pressure explains the counter-intuitive result that 'Ohmic heating' is dominant at low pressure, while 'Pressure heating' vanishes or even gets negative. This is caused by the large sheaths and the extinction of the classical plasma bulk. Instead, the electron density profiles moves back and forth between the electrodes within each RF period jointly with the energetic 'electron beams' generated during sheath expansion at each electrode. This shift of the electron density profile attenuates the 'ambipolar' field at each electrode during the local sheath collapse and reduces the deceleration of beam electrons generated at the opposite electrode. Thus, the electron temperature gets more symmetric in time within the RF period and 'Pressure heating' is greatly attenuated.
4. Conclusions
The electron power absorption dynamics in low pressure capacitive RF plasmas operated in argon at a frequency of 27.12 MHz, a driving voltage amplitude of 500 V, and a gap distance of 25 mm were studied using a self-consistent, spatio-temporally resolved analysis based on the first velocity moment (i.e. the momentum balance equation) of the Boltzmann-equation, for which the input quantities were taken from 1d3v electrostatic PIC/MCC simulations.
At 2 Pa (and above) the dominant power absorption mechanism on space- and time-average is 'Pressure heating', P∇p . This is due to the fact, that the 'ambipolar' electric field has a temporal asymmetry within the RF period originating from the time dependence of the electron temperature. This temporal asymmetry is a consequence of a self-amplifying mechanism during the phase of sheath expansion, where the presence of energetic electrons initially accelerated by the 'ambipolar' electric field increases the electron temperature and, thus, the magnitude of the 'ambipolar' electric field as well. This region of high electron temperature during sheath expansion is not present during sheath collapse, as incoming electrons are decelerated by the local 'ambipolar' electric field, which is high due to a steep local electron density profile.
Below 2 Pa, there is a 'transition' (at around 1.8 Pa), where 'Ohmic heating' starts to become the dominant power absorption term on space- and time-average. This is due to the fact that below this pressure the transit time of energetic electrons exceeds their collision time. Energetic beam electrons generated at one electrode propagate to the opposite electrode without colliding with gas atoms. Therefore, the phase between the electrons' velocity and the electric field will not be broken, or, equivalently, the electron temperature distribution at each electrode will be temporally symmetric, thereby leading to the attenuation of 'Pressure heating'. This mechanism is amplified as the pressure is decreased, whereby a pressure can be reached below which 'Pressure heating' is negative. This regime is characterised by a time-modulated electron density profile. This will happen, if the maximum sheath width gets comparable to half the electrode gap (it is ≈0.35L under the conditions investigated here). The large sheaths are a result of the low plasma density at low pressure due to the ineffective confinement of energetic electrons for the chosen frequency and gap length values.
At 1 Pa, the plasma is in a strongly non-local and resonant mode, where two 'electron beams' are generated during sheath expansion at each electrode, one of which hits the opposing sheath during its collapse thereby leading to a less effective beam confinement and a depleted electron density. The lower plasma density results in larger sheaths so that the plasma bulk practically vanishes and the electron density profile moves back and forth between the electrodes within each RF period jointly with the 'electron beams'. This oscillation of the electron density profile leads to an attenuation of the 'ambipolar' field at each electrode during the local sheath collapse, since the local density gradient is reduced. Thus, incoming energetic 'electron beams' generated during sheath expansion at the opposite electrode are no longer decelerated by the local 'ambipolar' electric field, when they approach the collapsing sheath edge. This effect leads to a temporally more symmetric behaviour of the electron temperature compared to scenarios, where the sheath width is smaller and the electron density maximum does not move. Thus, 'Pressure heating' is greatly reduced and 'Ohmic heating' is found to be dominant at low pressure due to the low plasma density and conductivity. As the power absorption associated with the pressure gradient, P∇p , consists of two terms, the 'ambipolar', P∇n , and the term proportional to the electron temperature gradient, P∇T , where the latter leads to 'cooling' due to a formation of a double layer of charges, the overall contribution of P∇p to the space- and time-averaged electron power absorption is negative at the lowest pressures studied in this work.
This spatio-temporal analysis of electron power absorption in low pressure electropositive capacitive RF discharges explains the counter-intuitive result that 'Ohmic heating' is dominant at the lowest pressure, at which the plasma can be sustained, and not 'Pressure or Stochastic heating'. These results are expected to be of significant fundamental relevance, since they contradict results of models of such plasmas. They provide the basis for a modified fundamental understanding of such discharges and for knowledge based optimization of low temperature RF plasma processes.
Acknowledgments
This work was funded by the German Research Foundation in the frame of the project, 'Electron heating in capacitive RF plasmas based on moments of the Boltzmann equation: from fundamental understanding to knowledge based process control' (No. 428942393). Support by the DFG via SFB TR 87, project C1, and by the Hungarian Office for Research, Development, and Innovation (NKFIH 119357) is gratefully acknowledged.