Abstract
Variable temperature magic angle spinning (MAS) NMR measurements are reported on 1H and 31P nuclei in KH2PO4 (KDP) in the vicinity of its paraelectric–ferroelectric phase transition temperature, Tc, of 123 K, to examine the transition mechanism, in particular if this is a model order–disorder type or whether it also involves a displacive component. It has been well established that the temperature variation of the isotropic chemical shift, δiso, in NMR measurements of the nuclei directly involved in the transition should remain constant or change smoothly through Tc for an order–disorder type transition but it should show an anomalous change for a displacive one. Here we demonstrate that the δiso for both 31P and 1H nuclei in KDP show clear anomalies as a function of temperature around KDP's Tc, providing direct evidence of a displacive component for the phase transition of KDP in contrast to the generally accepted notion that it is a model order–disorder type.
Export citation and abstract BibTeX RIS
The discovery of large, reversible electric polarization below a certain temperature, labeled the Curie point, Tc, in the KH2PO4 (KDP) family of hydrogen-bonded compounds [1] opened the field of ferroelectricity in the late 1930s. Over the last several decades, these ferroelectric materials have been studied extensively because of their extensive applications in electro-optic technology, such as laser frequency multiplication and power generation in the UV range, as well as for understanding the role of hydrogen bonding in a wide range of materials including ice and biological materials such as DNA [2, 3]. KDP has extensive O–H...O hydrogen-bonding network; the H's are located between the oxygen atoms of two neighboring phosphate (PO4) groups. KDP undergoes a paraelectric to ferroelectric phase transition at its Tc of 123 K [1]. The H's are intimately involved in the phase transition mechanism [1]. In the paraelectric region, i.e. higher temperature phase, the H's are found to be dynamically disordered between the two oxygens in the O–H...O bonds. While in the lower temperature, ferroelectric phase below 123 K, the H's are found to be localized closer to one or the other oxygen, leading to a lowering of the symmetry of the H2PO4 groups, and the development of electric polarization in the KH2PO4 lattice. The transition mechanism has been traditionally considered as the ordering of the H's in double-minimum type potential wells (figure 1, vide infra). That the hydrogen-bonded protons play a major role in the transition mechanism can be seen also from the fact that upon H → D substitution (i.e. deuteration), the Tc of KDP increases from 123 to 224 K [2, 3]. However, the atomistic view of the involvement of the K+–H2PO4 units is not fully understood. It is not clear, for example, whether the phase transition involves mainly the order–disorder motion of the O–H...O protons in their double-minimum potential well without the concomitant involvement of the K–PO4 framework, so-called order–disorder model [2, 3], or whether the transition involves also electronic structure changes in the whole molecular framework (displacive model) [2, 3].
Figure 1. Schematic representation of KH2PO4 (KDP) structures in (a) paraelectric phase and (b) ferroelectric phase viewed along the c-axis. Note that the two PO4 units are located at a distance of c/4 along the c-axis.
Download figure:
Standard image High-resolution imageIn order to distinguish between these two models, an earlier NMR study [4] attempted to detect any anomaly in the isotropic chemical shift δiso of 31P, using as an example deuterated KDP (KD2PO4), rather than KDP itself because (a) Tc of KD2PO4 (224 K) was experimentally easier to reach and (b) deuteration eliminated peak broadening due to the large 31P–1H dipolar interaction thus affording significantly higher spectral resolution. The fundamental premise of the study was that δiso is quite sensitive to electronic structure change due to any symmetry or structural change in the molecular framework around a given atom, but not to simple reorientational or translational changes in a molecular unit [5–10].
It seems worth noting that the premise that the isotropic chemical shift of the nuclei directly involved in the transition should remain constant (or vary smoothly) through the Tc is based on these two main considerations: (a) the general notion that an order–disorder ferroelectric phase transition usually involves an orientational (or positional) ordering of a polar moiety below the Tc, rather than a distortion in the chemical structure; and (b) the isotropic chemical shift is invariant to the orientational change or the displacement of this entity as a whole. These points have been fairly well established by the current authors [5, 7–9] and by others [4, 6]. For a ferroelectric transition of the 'displacive type', on the other hand, the polar moiety is thought to undergo a significant distortion in its chemical structure (electronic density distribution), and hence a significant anomaly in the isotropic chemical shift. As can be noted from the earlier work [4, 6] without MAS, it's rather difficult to delineate the isotropic part of the total chemical shift, since the signal position (total shift) and line-shape are dominated by the anisotropic components.
It is known also that in the case of H's in an O–H...O hydrogen bond, such as is the case for KDP, the δiso of the H depends sensitively on the variation in the O–O distance as well as the electronegativity effect of the surrounding PO4 units [11]. Crystal studies of KDP [2, 3] reveal no significant variation in the O–O distance as T is varied through Tc (even though the H becomes localized in the middle of the O–O bond). The measured change in the proton δiso is thus directly related to the electronic density redistribution on the proton as the phase transition ensues. Similar arguments apply to 31P isotropic chemical shift.
In the aforementioned NMR study of KDP family [4] no anomaly in the isotropic chemical shift was detected, admittedly within the large error bars evident in the data, owing to the fact that the authors had used wide-line NMR techniques. This result has thus been considered as a definitive evidence for showing the KDP-type materials being model order–disorder systems [3–5].
On the other hand, more recent NMR studies, using the currently available techniques that can provide much higher spectral resolution, as obtained via magic angle spinning (MAS), have reported a significant anomaly in the 31P δiso around the Tc of KD2PO4 [12]. These results questioned the conclusions drawn in the earlier work that the KDP family was a model order–disorder lattice of hydrogen-bonded systems [4]. It was thus of interest to carry out such measurements on KDP itself; in part also because the significant difference in the quantum tunneling characteristics of H and D are hard to translate to their chemical shift behavior. But until rather recently, commercial instrumentation to carry out MAS well below the KDP phase transition (123 K) was not available. Our initial studies related to this question [13] were thus carried out on their antiferroelectric analogue, NH4H2AsO4, since its transition temperature (216 K) was achievable for MAS NMR studies. At present the triple resonance MAS probe with the 600 MHz NMR spectrometer at the National High Magnetic Field Laboratory in Tallahassee covers the range down to 100 K, which provided a strong impetus for reexamining KDP.
Single crystals of KDP were grown by slow evaporation of commercial grade powder (Aldrich, 99% pure) dissolved in doubly distilled water at room temperature [8]. The crystals grew quite transparent bricks with prismatic ends, elongated as usual along the c-axis. The 3D structure of KDP has been well described in the literature [1–3]. Figure 1 shows the crystal structure of KDP viewed along the tetragonal c-axis in the paraelectric (a) and ferroelectric phase (b) [1–3, 14], where the PO4 groups and K+ ions locate alternatingly along the c-axis. The PO4 groups are linked by hydrogen bonds in a 3D way. At the paraelectric phase, the O–H...O protons are delocalized between the two minima positions of a double-minimum potential well along the O–H...O bond (figure 1(a)). In the ferroelectric phase, protons are localized at one or the other position of this double minimum potential well, as shown in figure 1(b) [15–17].
A small (~1 × 1 × 1 mm3) piece of visually defect-free crystal was packed in a 3.2 mm MAS rotor and used for MAS NMR measurements in order to obtain higher resolution by obviating the anisotropic bulk magnetic susceptibility [9, 10, 12]. The 1H and 31P MAS NMR line-shape measurements were made using a 3.2 mm Bruker triple resonance MAS probe on a 600 MHz spectrometer. The resonance frequency for 31P and 1H was 242.95 and 600.13 MHz, respectively. In the probe all three gas channels of bearing, drive (these two are used for spinning samples) and VT gas were vacuum insulated and were cooled using the cryocooling unit PH2700, enabling the measurements over the range of 300 K down to about 100 K.
A standard π/2-τ-π-τ spin-echo pulse sequence, where 2τ is the echo time, was used to record 1H signals in order to suppress the 1H background signals from the probe components. Adamantane (1.63 ppm, relative to TMS) was used as the chemical shift reference [18]. The 1H MAS NMR spectra were recorded using a spinning speed of 10 kHz and τ was set to a multiple of the spinning period. 31P spectra were recorded under high-power 1H decoupling in a direct polarization mode and spinning speed of 7.5 kHz. A 62.5 % H3PO4 solution at 0 ppm was used as the 31P chemical shift reference.
Sample temperature was regulated through the three gas channels using a Bruker BVT-3000 unit with the accuracy of ±0.1 K. The temperature range of measurement was from 100 K to 153 K. At every temperature, the three gas channels were set to the same temperature values. Spectra were collected at 1 K intervals around Tc, and 2–5 K intervals at other temperatures, with a waiting time of at least 5 min for temperature equilibration at each point. The value of Tc was defined as the temperature of the steepest rise of the chemical shift versus temperature curve.
Figure 2(a) shows some typical 1H MAS NMR spectra of KDP around its phase transition temperature Tc = 123 K. The spectra show a definitive shift in the peak position, characterized by the isotropic chemical shift, δiso, around Tc, as can be seen from figure 2(b). Figure 3 shows the temperature dependence of the 1H signal line width. It is seen that with decrease in temperature, the line width gradually increases until Tc and then becomes essentially constant as the temperature is lowered further, implying that below Tc the protons localize in a structurally different environment than the average of the two high-temperature positions, thereby indicating that the phase transition involves a displacive component. Additional, more quantitative, data on the motional dynamics were obtained via spin-lattice relaxation time, T1, measurements.
Figure 2. (a) Typical 1H MAS spectra of KDP showing a shift at Tc (123 K). (b) Temperature dependence of 1H isotropic chemical shift δiso.
Download figure:
Standard image High-resolution imageFigure 3. Temperature dependence of line width of 1H MAS NMR spectra.
Download figure:
Standard image High-resolution imageWhile measuring the proton T1 for KDP we noted that the T1 is quite long, about one hundred seconds, at temperatures close to Tc (123 K). It was thus feasible to make T1 measurements at only a few selected few points on both sides of the phase transition temperature. Some typical data are shown in figure 4. The analysis procedure was helped by the observation that the T1 trend was a linear increase with decrease in temperature, a sign of slow motion according to the Bloembergen–Purcell–Pound (BPP) model [19].
Figure 4. Temperature dependence of spin-lattice relaxation time (T1) of 1H in KDP.
Download figure:
Standard image High-resolution imageIn the slow motion limit, the BPP model provides the following well-tested equation [19]:

where γ is the proton gyromagnetic ratio, M2 the second moment, ω0 the Larmor frequency. Furthermore, we assume that τc obeys the Arrhenius equation,

where Ea the activation energy, R is the gas constant, and τo the infinite-temperature correlation time. In the 'slow motion' limit, i.e. , equation (1) becomes:
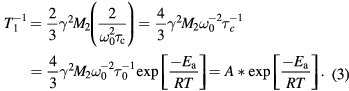
Thus, from the fitting of the temperature dependent 1H NMR T1 rates () by equation (3), the activation energy (Ea) was obtained as 1.0 ± 0.1 kJ mol−1 for T > Tc and 3.5 ± 0.7 kJ mol−1 for T < Tc. The activation energy thus increases by a factor of about four from paraelectric to ferroelectric phase, indicating the proton motion slows down at the ferroelectric phase boundary. These activation energy values are in agreement with similar values reported by Kim et al [20] using the broad-line NMR, and by Beutier et al [21] using resonant x-ray diffraction.
Figure 5(a) shows typical 31P MAS NMR spectra of KDP around Tc = 123 K. In contrast to the conclusions from the broad-line NMR data [4], we here show that the 31P δiso exhibits a significant shift on going through Tc, as illustrated in figure 5(b). As the temperature approaches Tc from above, the 31P δiso initially moves to down-field (higher chemical shift values) and then rapidly to up-field (lower chemical shift values) when going to lower temperatures through Tc, indicating increased electron density on the nearby P–O bonds lying in the hydrogen bond direction [22]. Clearly the δiso of the PO4 moiety as in figure 5(b) undergoes a significant abrupt change around Tc, again implying the presence of a displacive character for the ions while taking part in the ferroelectric phase transition.
Figure 5. (a) 31P MAS spectra of KDP at 243 MHz recorded around Tc. (b) Temperature dependence of 31P chemical shift.
Download figure:
Standard image High-resolution imageUsing the same procedure as for the protons, we measured T1 for 31P. Unfortunately, the T1 was found to be over 10 min even at ambient temperature. Since T1 was expected to be much longer at temperatures close to Tc, it was thus considered to be not a worthwhile experiment to carry out detailed measurement of its temperature dependence, keeping in mind also that the major goal of this study was to use the temperature dependence of δiso as a probe of the displacive character of KDP's phase transition.
In conclusion, high-field (14.1 T) MAS NMR measurements of temperature dependence of the δiso of the 1H and 31P nuclei in KDP reveal that δiso for both nuclei undergoes anomalous changes around the transition temperature implying significant atomic structure change at the Tc. Supporting evidence for anomalous atomic displacements (evidence of a displacive character at the transition temperature) is provided by a systematic probing of the linewidths and spin-lattice relaxation time, T1. These results strongly suggest that the KDP phase transition should thus be characterized as a mixed order–disorder and displacive type [9, 23], in contrast to the generally accepted notion that KDP is a model order–disorder lattice [2–4].
Finally, we note that the present work has broader significance in the phase transition field as follows. At the NMR timescale (millisecond to sub-picosecond), the atomic fluctuations underlying the ferroelectric transitions in the hydrogen-bonded ferroelectrics, usually considered as model order–disorder systems, and the inorganic BaTiO3-type perovskites, usually thought of as model displacive materials, exhibit both order–disorder and displacive characteristics, rather than purely one or the other [8].
Acknowledgments
We are grateful for financial support from the User Collaboration Grants Program (UCGP) at the National High Magnetic Field Laboratory which is supported by National Science Foundation (NSF) Cooperative Agreement No. DMR-1157490, the State of Florida, and the U.S. Department of Energy. J J Kweon and E S Choi also acknowledge NSF-DMR 1309146 for financial support. We thank also Dr N Abhyankar for providing KDP single crystals, Dr W Brey and J Kitchen for the design and fabrication of a tuning box for 31P measurements and Dr Ivan Hung for help with the low temperature set up.