ABSTRACT
In this work, we conclude the analysis of our CO line survey of luminous infrared galaxies (LIRGs: LIR ≳ 1011 L☉) in the local universe (Paper I) by focusing on the influence of their average interstellar medium (ISM) properties on the total molecular gas mass estimates via the so-called Xco = M(H2)/Lco, 1–0 factor. One-phase radiative transfer models of the global CO spectral line energy distributions (SLEDs) yield an Xco distribution with 〈Xco〉 ∼ (0.6 ± 0.2) M☉ (K km s−1 pc2)−1 over a significant range of average gas densities, temperatures, and dynamic states. The latter emerges as the most important parameter in determining Xco, with unbound states yielding low values and self-gravitating states yielding the highest ones. Nevertheless, in many (U)LIRGs where available higher-J CO lines (J = 3–2, 4–3, and/or J = 6–5) or HCN line data from the literature allow a separate assessment of the gas mass at high densities (⩾104 cm−3) rather than a simple one-phase analysis, we find that near-Galactic Xco ∼ (3–6) M☉ (K km s−1 pc2)−1 values become possible. We further show that in the highly turbulent molecular gas in ULIRGs, a high-density component will be common and can be massive enough for its high Xco to dominate the average value for the entire galaxy. Using solely low-J CO lines to constrain Xco in such environments (as has been the practice up until now) may have thus resulted in systematic underestimates of molecular gas mass in ULIRGs, as such lines are dominated by a warm, diffuse, and unbound gas phase with low Xco but very little mass. Only well-sampled high-J CO SLEDs (J = 3–2 and higher) and/or multi-J observations of heavy rotor molecules (e.g., HCN) can circumvent such a bias, and the latter type of observations may have actually provided early evidence of it in local ULIRGs. The only way that the global Xco of such systems could be significantly lower than Galactic is if the average dynamic state of the dense gas is strongly gravitationally unbound. This is an unlikely possibility that must nevertheless be examined, with lines of rare isotopologues of high gas density tracers (e.g., H13CN, high-J13CO lines) being very valuable in yielding (along with the lines of the main isotopes) such constraints. For less IR-luminous, disk-dominated systems, we find that the galaxy-averaged Xco deduced by one-phase models of global SLEDs can also underestimate the total molecular gas mass when much of it lies in an star-formation-quiescent phase extending beyond a central star-forming region. This is because such a phase (and its large Xco) remains inconspicuous in global CO SLEDs. Finally, detailed studies of a subsample of galaxies find ULIRGs with large amounts (∼109 M☉) of very warm (⩾100 K) and dense gas (≳105 cm−3), which could represent a serious challenge to photon-dominated regions as the main energy portals in the molecular ISM of such systems.
Export citation and abstract BibTeX RIS
1. INTRODUCTION
Soon after the discovery of the luminous infrared galaxies (LIRGs), whose bolometric luminosities are dominated by the infrared part of their spectral energy distributions (SEDs; LIR ⩾ 1011 L☉; e.g., Soifer et al. 1987), single-dish and interferometric CO J = 1–0, 2–1 line observations were used to determine their total molecular gas mass and its distribution (Sanders et al. 1988a, 1991; Tinney et al. 1990; Wang et al. 1991; Solomon et al. 1997; Downes & Solomon 1998; Bryant & Scoville 1996, 1999). These efforts were paralleled by several investigations of the so-called Xco = M(H2)/LCO(1–0) factor and its dependence on the average interstellar medium (ISM) conditions both theoretical (Dickman et al. 1986; Maloney & Black 1988; Wolfire et al. 1993; Sakamoto 1996; Bryant & Scoville 1996; Wall 2007) and observational (e.g., Israel 1988, 1997; Israel et al. 1993; Solomon et al. 1997; Downes & Solomon 1998; Yao et al. 2003). The average molecular gas conditions in LIRGs used in such studies have typically been constrained using CO(2–1)/(1–0) and CO/13CO J = 1–0, 2–1 line ratios (e.g., Braine & Combes 1992; Horellou et al. 1995; Aalto et al. 1995; Papadopoulos & Seaquist 1998). Higher-J transitions (J = 3–2 and higher) were used only sporadically and mostly for star-forming galactic nuclei (e.g., Devereux et al. 1994; White et al. 1994; Güsten et al. 1996; Nieten et al. 1999; Mauersberger et al. 1999; Dumke et al. 2001; Yao et al. 2003). This was a result of the larger difficulties, which such observations pose in terms of available submillimeter (submm) receivers, their sensitivity, and the dry weather conditions needed (especially for ν ≳ 460 GHz, CO J = 4–3). Receiver sensitivity limitations also hindered large multi-J line surveys of the much fainter lines from heavy rotor molecules such as HCN that probe higher density gas (>104 cm−3) except in nearby galactic nuclei (Jackson et al. 1995; Paglione et al. 1997) and a few luminous ULIRGs (e.g., Graciá-Carpio et al. 2008).
Such limitations will soon be overcome after the ongoing commissioning of the Atacama Large Millimeter Array (ALMA) is completed. Then, routine multi-J observations of CO and heavy rotor molecules will yield an unhindered view of the entire range of physical conditions in molecular clouds, from their quiescent and low-density phases (n(H2) ∼ (102–103) cm−3, Tkin ∼ (10–15) K) to the dense and warm gas intimately associated with star formation (n(H2) ∼ (104–107) cm−3, Tkin ∼ (30–150) K). The power of interferometric multi-J line imaging in revealing the mass distribution of dense warm star formation (SF) gas in LIRGs has already been demonstrated by pioneering Submillimeter Array observations (Sakamoto et al. 2008; Wilson et al. 2008; Iono et al. 2007, 2009), while in the grand spiral M 51 CO line ratio imaging at high resolution revealed active galactic nucleus (AGN)-excited gas in its nucleus (Iono et al. 2004). The influence of the high-excitation conditions found in SF regions gas on the Xco in galaxies may not necessarily be strong since dense and warm SF gas amounts to only ∼(0.5–3)% of typical giant molecular clouds (GMCs) mass. Even smaller fractions of the total molecular gas in spiral disks resides in their centers (∼(0.1–1)%), where strong tidal fields, high cosmic-ray (CR) energy densities, and/or AGNs can drive a high molecular line excitation. Nevertheless, this may no longer be true for the merger-driven starbursts in ULIRGs, where a dense SF gas phase can contain the bulk of their total molecular gas mass (e.g., Solomon et al. 1992; Gao & Solomon 2004). Moreover, cases of AGN-driven mechanical and radiative feedback affecting the bulk of the molecular gas of the host galaxy and the corresponding CO spectral line energy distributions (SLEDs) have now been identified (Papadopoulos et al. 2008; van der Werf et al. 2010). These systems, along with ULIRGs, yield a nearby glimpse of ISM conditions that could be prevailing in the distant universe.
In the present work, we examine the influence of the average molecular gas conditions found in LIRGs (Papadopoulos et al. 2012, hereafter Paper I) on the Xco factor. We do so by using the largest combined database of LIRGs/CO transitions for which such a study has been conducted, while also discussing the limitations and potential biases of past theoretical and observational studies. We then outline methods that could be employed in the upcoming era of ALMA, and the special role the Herschel Space Observatory (HSO) can play, toward improved total molecular gas mass estimates, especially for ULIRGs (LIR > 1012 L☉). Several such galaxies whose CO line ratios indicate extreme ISM conditions (see Paper I) are studied individually, and their impact on the Xco values is examined in detail. Throughout this paper, we adopt a flat Λ-dominated cosmology with H0 = 71 km s−1 Mpc−1 and Ωm = 0.27.
2. MOLECULAR GAS PHYSICAL CONDITIONS AND MASS ESTIMATES IN LIRGs
The formal dependence of the Xco factor on the average density, temperature, and kinematic state of large molecular cloud ensembles (where the statistical notion of Xco remains applicable) is explored in several papers (e.g., Dickman et al. 1986; Young & Scoville 1991; Bryant & Scoville 1996; Solomon et al. 1997; Papadopoulos & Seaquist 1999; Downes & Solomon 1998; Yao et al. 2003). CO and 13CO lines can yield constraints on these ISM properties and thus on the corresponding Xco, via radiative transfer models (e.g., Mao et al. 2000; Weiss et al. 2001). In this regard, low-J CO SLEDs (up to J = 3–2) with ncrit ∼ (400–104) cm−3 and EJ/KB ∼ (5.5–33) K are adequate for determining the average state of the molecular gas and thus the appropriate Xco, provided that most of its mass is distributed in ordinary GMCs. The low-J CO SLED segment and its modeling can then in principle yield the mass normalization for the entire CO or of any other molecular line SLED (e.g., of HCN) typically emanating from much smaller mass fractions. For several ULIRGs in our sample whose CO SLEDs will be extended up to J = 13–12 using the HSO, this normalization is especially important, as it allows us to determine the mass of the highly excited molecular gas emitting the very high J lines and to set important constraints on the energy source responsible for its excitation (van der Werf et al. 2010; Rangwala et al. 2011).
2.1. Prior Work and Some Methodological Limitations
There are currently only three major observational studies of Xco using CO lines for substantial LIRG samples (Solomon et al. 1997; Downes & Solomon 1998; Yao et al. 2003). For the highly turbulent molecular gas in (U)LIRGs, these typically find Xco = (1/10–1/3)Xco, Gal, with the low values attributed mostly to non-self-gravitating gas distributions. For SF spiral disks, an Xco, Gal ∼ (4–5) Xl6 remains applicable as most of their molecular gas is found in cool, low-density, self-gravitating GMCs such as those in the Milky Way, pockmarked by SF "spots" of warm and dense gas also with nearly Galactic Xco (e.g., Young & Scoville 1991). In isolated spirals, low Xco ∼ (1/20–1/5)Xco, Gal values can be found only in their nuclei (2r ≲ 100–200 pc; Regan 2000), but involve only small fractions of the total molecular gas reservoirs. Thus, for the metal-rich environments of LIRGs, current observational work points to a bimodal Xco distribution, with near-Galactic values for all isolated spiral disks, and 〈Xco〉 ∼ (1/4–1/5) × Xco, Gal in the merger-driven starbursts of ULIRGs. This view has been widely adopted even for high-z star-forming LIRGs where only sparse sampling of their CO SLEDs exists (e.g., Greve et al. 2005; Tacconi et al. 2006; Dannerbauer et al. 2009). It is worth noting that the claimed bimodality of the Schmidt–Kennicutt star formation relations (linking star formation rate (SFR) and molecular gas supply) between disks and mergers (Daddi et al. 2010b; Genzel et al. 2010) is nearly equivalent to such an Xco bimodality (Narayanan et al. 2011 and references therein).
Nevertheless, much of the aforementioned observational work contains some serious methodological limitations borne out of the limited molecular line data available per object and specific assumptions made about the radiative transfer models. These are as follows:
- 1.Setting Tkin = Tdust in the CO line radiative transfer models used to constrain Xco even though photoelectric, turbulent, or CR heating can easily induce Tkin ≫ Tdust.
- 2.Using a constant dV/dR (usually 1 km s−1 pc−1) in the large velocity gradient (LVG) radiative transfer models that interpret CO line ratios even if: (1) virial gas motions alone correspond to ∼8–10 times higher values for the dense gas (n ≳ 105 cm−3) that may be dominant in ULIRGs, and (2) stellar mass concomitant with molecular gas and/or tidally induced velocity fields can easily yield much larger dV/dR values.
- 3.Using empirical relations and object-specific assumptions to obtain Xco expressions that can account for the potentially significant stellar mass concomitant with the molecular gas, an issue that arises especially in ULIRGs.
- 4.The molecular lines used in such studies (low-J CO lines) are insensitive to the properties of the gas with densities n(H2) > 103 cm−3.
The last two set the most serious methodological limitations on the very influential study of Xco in ULIRGs by Downes & Solomon (1998, hereafter DS98), whose results are widely used for presumably similar systems at high redshifts. In that study, models of the CO J = 1–0, 2–1 interferometric images of five ULIRGs were used to deduce Xco ∼ (1/5–1/6)Xco, Gal, attributing these low values to a continuous molecular gas distribution encompassing large fractions of stellar mass and thus velocity fields determined by the total (gas)+(stellar) mass (see also Downes et al. 1993; Solomon et al. 1997). The use of empirical relations (e.g., LIR/L'co = 200 L☉ (K km s−1 pc2)−1) and setting an rm, * = Mnew, */Mbulge, * ∼ 0.5 mass ratio of newly formed stars (Mnew, *) to those in an old stellar bulge (Mbulge, *) in the pre-merger spirals make the computed Xco specific to the few ULIRGs studied and certainly not automatically applicable to other ULIRGs in the local or the distant universe. Moreover, "freezing" dV/dR to 1 km s−1 pc−1 renders such models incapable of constraining the average dynamic state of the gas and thus of exploring its effect on Xco in a straightforward way (see Section 2.2).
Furthermore, while the DS98 formalism can be generalized to arbitrary rm, * values, it remains impractical for the dusty gas-rich systems at high redshifts where stellar populations are heavily dust-enshrouded. In addition, an Xco factor deduced solely from CO J = 1–0, 2–1 line emission models may be inapplicable for a much denser gas phase (n(H2) ≳ 104 cm−3). This will yield a small error when such a phase represents only a few percent of the total mass per typical GMC (as is the case in spiral disks), but it will yield a potentially much larger error if the dense gas dominates the molecular gas mass budget. These difficulties are compounded by setting dV/dR = 1 km s−1 pc−1, which, while justified by the sole CO(2–1)/(1–0) line ratio available in the DS98 study, may be partly responsible for the contradictory results obtained for the average gas density and CO line excitation. Indeed, while HCN and high-J CO observations indicate much of the gas in some ULIRGs to be very dense (n(H2) > 104 cm−3), radiative transfer models of CO J = 1–0 and J = 2–1 interferometric images of some of the same ULIRGs (e.g., Arp 220) become incompatible with these images already for n ≳ 103 cm−3 (DS98). Velocity gradients of dV/dR ≫ 1 km s−1 pc−1 (expected for the highly turbulent gas disks of ULIRGs) and high temperatures for the dense gas can greatly reduce line optical depths and the emergent CO line emission, possibly alleviating these discrepancies.
In summary, all current observational studies of Xco in LIRGs leave its range and dependence on the average density, temperature, and kinematic state of the molecular gas still largely unexplored. The small LIRG/CO line data sets used in such studies, and the aforementioned limitations of the analysis methods, limit their applicability to other such systems in the local or the distant universe.
2.2. The Average State of the Molecular Gas and Xco
Our data set of total CO line luminosities for a substantial sample of LIRGs provides a good opportunity for exploring the Xco dependence on the average ISM state in vigorously star-forming systems. The average dV/dR for the gas is now a free parameter to be constrained along with the density and temperature by LVG radiative transfer models. This provides an extinction-free and straightforward method for determining the effects of stellar mass concomitant with molecular gas and/or tidal disruption of molecular clouds on Xco.
To place our discussion regarding molecular gas mass estimates in LIRGs in an autonomous context, we reproduce the Xco factor (see Appendix A) as

where α = 0.55–2.4 depending on the assumed cloud density profile (see Bryant & Scoville 1996, their Equations (A11), (A17)), and n(H2) and Tb, 1–0 are the average gas density and CO J = 1–0 brightness temperature of the molecular cloud ensemble. The parameter Kvir is given by

(with Λco = [CO/H2]/(dV/dR) being one of the outputs of a typical LVG model). This determines the average dynamic state of the molecular gas, with Kvir ∼ 1–3 for the (mostly) self-gravitating GMCs in the Galactic disk (and Kvir ≪ 1 corresponding to dynamically unattainable gas motions). For a typical value of α = 1.5, Equation (1) becomes

which we use in the present work. For ordinary GMCs with n(H2) ∼ 500 cm−3, Tb, 1–0 ∼ 10 K, and Kvir ∼ 1–2, the latter yields Xco ∼ (3–6) Xl (where Xl = M☉(K km s−1 pc2)−1).
The optically thin limit for CO J = 1–0 line emission is often adopted to provide a lower limit for the total molecular gas mass (e.g., Bryant & Scoville 1996; Solomon et al. 1997). The availability of several CO lines allows a more robust such limit without assuming LTE:
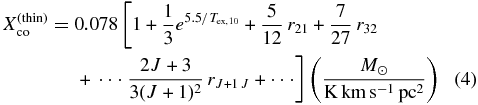
(see Appendix A), which makes obvious that unless the CO line ratios become significantly larger than unity (which they can in optically thin emission), most of the contributions come from the low-J levels. The LTE expression is

which gives a higher (and less reliable) lower limit since the LTE partition function can be significantly larger than the non-LTE one. The optically thin approximation also can be used for the realistic case of significant CO line optical depths as long as large velocity gradients keep the photon escape probability local throughout the bulk of the molecular gas mass. Then, Equation (4) is modified to
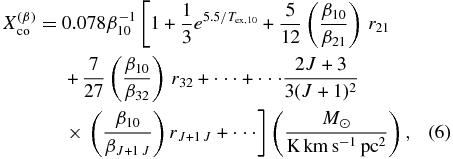
where βJ + 1 J = [1 − exp (− τJ + 1 J)]/τJ + 1 J is the photon escape probability (see Appendix A). The last equation provides a more robust lower limit than Equation (4), as it accounts for finite line optical depths (computed from radiative transfer models). If enough line ratios are available, the estimate from Equation (6) will approach that of Equation (3) as long as one average gas phase dominates the emergent CO line emission.
In Figure 1, we show the Xco and X(thin)co(LTE) distributions, computed from Equations (3) and (5) and the results of one-phase radiative transfer models for the CO SLEDs and 13CO lines for all the LIRGs in our sample (see Paper I). Most Xco values are ≲1.5 Xl, with 〈Xco〉 ∼ 0.60 Xl obtained for the main distribution (excluding the few outliers beyond 3 Xl). The X(thin)co(LTE) distribution gives similar results, indicating that most of the states compatible with the CO SLEDs have optically thin or moderately optically thick CO J = 1–0 line (τ10 ∼ 1–3). This has been noted in the past in the context of a two-phase ISM model and was attributed to the high temperatures of a turbulent "envelope" phase in molecular clouds (Aalto et al. 1995).
Figure 1. Xco factors estimated from Equations (3) and (5) using the results from one-phase LVG radiative transfer models. The black bar histogram corresponds to the best and least degenerate solutions obtained from these models.
Download figure:
Standard image High-resolution imageThe Xco range obtained using our one-phase models of global CO SLEDs is similar to that reported by DS98 and Yao et al. (2003). Moreover, in Figure 2 the distribution of demonstrates that, in contrast with what is often stated, the effects of density and temperature do not always cancel out but are instead responsible for much of the Xco variations over different ISM environments (compare Figure 2 with the Xco distribution in Figure 1). Nevertheless, the average dynamic state of the gas (i.e., Kvir) remains the most important factor affecting the average Xco. This is shown in Figure 3, where the Xco distributions for virial/near-virial and unbound average gas dynamic states are shown. In the merger-driven starbursts of ULIRGs, highly non-virial gas velocities can be caused by significant amounts of stellar mass concomitant with a continuous molecular gas distribution, as noted by DS98 (see also Solomon et al. 1997), albeit without using radiative transfer models to constrain Kvir. Strong tidal fields acting on GMC cloud envelopes and/or a diffuse intercloud molecular gas distribution whose velocity field traces the combined potential of the surviving dense GMC cores as well as stars will both raise the average Kvir, and further reduce the corresponding Xco (Equation (3)).
Figure 2. Distribution of the factor for the LVG solutions obtained for the sample (black bars are the same as in Figure 1).
Download figure:
Standard image High-resolution imageFigure 3. Xco factors computed from Equation (3) and the one-phase LVG solutions obtained for the CO line ratios measured in LIRGs (see Paper I) for two different dynamic regimes of average gas motions: self-gravitating (1 ≲ Kvir ≲ 4) and unbound (Kvir > 4).
Download figure:
Standard image High-resolution imageFigure 4 shows the distribution of X(β)co computed from Equation (6), which, unlike that deduced from Equation (3), uses the CO line ratios explicitly. This makes it more suitable when fully sampled CO SLEDs from J = 1–0 up to high-J (e.g., J = 6–5, 7–6) are available and when the turbulent models of the hierarchical density structures of molecular clouds (e.g., Ossenkopf 2002) become refined enough to provide global CO and 13CO SLEDs as well as the corresponding βJ + 1, J values per density "sub-phase." For well-sampled 13CO SLEDs, the X(β)co expression (using the appropriate [13CO/H2] abundance) is even more useful as βJ + 1, J(13CO) ∼ 1. In our current study, using the output βJ + 1 J values from our one-phase models in Equation (6) yields an X(β)co distribution similar to that of Xco from Equation (3).
Figure 4. Xco factor, estimated from Equation (6) using the βJ + 1 J values computed from one-phase radiative transfer models.
Download figure:
Standard image High-resolution imageInterestingly, the Xco distributions, while concentrated within ∼(0.3–1.5) Xl, do extend out to Galactic values ∼(2.5–6) Xl. These are found for disk-dominated LIRGs (e.g., NGC 157 and Mrk 1048), (U)LIRGs with unexpectedly "cold" CO ratios (e.g., IRAS 05189−2524) where cool (∼15 K) gas with low/modest densities (∼(102–103) cm−3) dominates their average ISM state. Surprisingly, Galactic Xco values can be found also in some ULIRGs whose "hot" CO ratios (e.g., IRAS 08572+3915) indicate a dominant warm (∼(100–150) K) and dense (∼(3 × 104–106) cm−3) phase or where other evidence (e.g., multi-J HCN lines) suggests a massive, dense, gas phase (e.g., Mrk 231). Thus, large Xco values are possible for both high- and low-excitation gas phases and therefore cannot be used as indicators of Galaxy-type ISM conditions in distant star-forming disks (e.g., Daddi et al. 2010a). This also suggests that in many local ULIRGs and similar systems at high redshifts the widely adopted Xco ∼ (0.8–1) Xl values underestimate their molecular gas mass.
2.3. Eddington-Limited Star Formation and a Minimum Molecular Gas Mass
Recent studies of SF feedback suggest a maximum g, * = L(*)IR/M*(H2) ∼ 500 (L☉/M☉) for the dense and warm gas M*(H2) near SF sites in galaxies as a result of strong radiation pressure from the nascent O, B star clusters onto the concomitant dust of the accreted gas fueling these sites (Scoville 2004; Thompson et al. 2005; Thompson 2009). Thus, provided that average dust properties (e.g., its effective radiative absorption coefficient per unit mass) remain similar in metal-rich star-forming systems such as LIRGs, a near-constant
g, * is expected among them. A value of
g, * ∼ 500 (L☉/M☉) is actually measured in individual SF sites of spiral disks such as M 51 and entire starbursts such as Arp 220 (Scoville 2004), while ∼(440 ± 100) (L☉/M☉) is obtained for CS-bright star-forming cores in the Galaxy (Shirley et al. 2003). Further evidence for a near-constant
g, * is the tight linear LIR–HCN(1–0) correlation found for individual GMCs up to entire ULIRGs (Wu et al. 2005) with HCN J = 1–0 used as a dense gas mass tracer. However, the intermittency expected for galaxy-sized molecular gas reservoirs (i.e., at any given epoch of a galaxy's evolution some dense gas regions will be forming stars while others will not) will lower the global
g, * to ∼1/3–1/2 of the Eddington value (Andrews & Thompson 2011).
Similar g, * values can be obtained without explicit use of the Eddington limit (and the detailed dust properties it entails) but from the typical L*/Mnew, * in young starbursts where Mnew, * is the mass of the new stars and L* is their bolometric luminosity (∼L(*)IR for the deeply dust-enshrouded SF sites). For
SF, c = Mnew, */[Mnew, * + M*(H2)] as the SF efficiency of the dense gas regions where the new stars form, it is

For SF, c ∼ 0.3–0.5 typical for dense SF regions and L(*)IR/Mnew, * = (300–400) (M☉/L☉) (Downes & Solomon 1998 and references therein), Equation (7) yields
g, * ∼ (130–400) (M☉/L☉). Here, we choose
g, * = 250 (L☉/M☉), close to the average values from Equation (7) and the blackbody limit deduced for compact CO line emission concomitant with an optically thick (τ100 μm > 1) dust emission (Solomon et al. 1997). Eddington-limited star formation in LIRGs implies a minimum molecular gas mass MSF = L(*)IR/
g, * fueling their observed SFRs. In Figure 5, we show the MSF distribution, which, for the ULIRGs in our sample, reaches up to ∼5 × 109 M☉, i.e., surpassing the total molecular gas reservoirs of typical spirals.
Figure 5. Minimum molecular gas masses necessary for fueling and Eddington-limited star formation in the LIRGs of our sample (see Section 2.3).
Download figure:
Standard image High-resolution image2.4. Computing Xco in a Two-Component Approximation
In some cases where one-phase LVG models of global CO line ratios of LIRGs do not converge to a well-defined range of physical conditions, a simple two-phase model can be used to examine the underlying ISM conditions and the corresponding Xco factors. The total CO J = 1–0 luminosity can then be expressed as

where (h) and (l) indicate the high- and the low-excitation phases, X(h)co is the CO–H2 conversion factor for the former, and g, * = 250(L☉/M☉). This assumes that the high-excitation phase fuels Eddington-limited star formation. For any other transition,

The molecular gas near H ii regions and interfaces between molecular clouds and O,B stellar associations is an obvious choice for obtaining template high-excitation CO SLEDs. The beam-matched CO line survey of the Orion A and B GMCs found r21 = 1.2–1.3 and R21 = 10 (the CO/13CO J = 2–1 ratio) in such "hot" spots (Sakamoto et al. 1994). These ratios, along with Tkin ⩾ 100 K (expected from the high CO line brightness temperatures in such SF spots), set as constraints to a one-phase LVG model yield the following: Tkin = (125–150) K, n(H2) = 3 × 105 cm−3, Kvir = 7, and corresponding line ratios: r(h)21(3) = 1.35, r(h)32 = 1.33, r(h)43 = 1.30, r(h)54 = 1.27, r(h)65 = 1.25, and r(h)76 = 1.22. For this LVG solution set, we compute (Equation (3)) X(h)co ∼ 2.2 Xl and from Equations (8) and (9) the CO line ratios of the low-excitation (l)-phase. These can then be used as constraints on one-phase LVG models to obtain the average ISM conditions and X(l)co. For such a two-phase decomposition, the effective X(2 − ph)co factor would be

with ρ(l − h)co = L(l) 'co, 1–0/L(h) 'co, 1–0. Using the Eddington-limited normalization for yields

When lines such as higher-J CO transitions (J = 4–3, 6–5) and/or heavy rotor molecular lines (e.g., HCN) are available, they are used to set constraints on the (h)-phase, ρ(l − h)co, and X(2 − ph)co without assuming the (h)-phase CO SLED and X(h)co deduced from the Orion GMC star-forming "spots."
3. THE Xco FACTOR IN LIRGs
The average ISM conditions deduced using the CO lines available per LIRG (see Paper I) encompass the [n(H2), Tkin, Kvir] parameter space, where most of the mass is expected to reside in ordinary GMCs. Thus, the narrow Xco distribution around 〈Xco〉 ∼ 0.6 Xl (Figures 1 and 4) does not seem to be the result of an excitation bias induced by the particular molecular lines used. This is not to say that other high-J CO or, e.g., HCN, line luminosities are expected to be compatible with the average ISM states deduced from our current CO line data set. Rather, it indicates that such lines with their higher critical densities will probe gas mass fractions per typical GMC too small to be of any consequence when it comes to the global Xco.
It is tempting to consider the aforementioned picture as complete. It is certainly compatible with models of supersonic turbulence in ordinary GMCs, where most molecular gas mass is found at densities < 104 cm−3, its conditions thus "accessible" to the CO, 13CO lines used to constrain them (and Xco) in our study. In the merger-driven starbursts of ULIRGs, however, GMCs may be far from ordinary. Stripping their outer envelopes by strong tidal fields and/or the large pressures from a hot ionized gas phase resulting from H i cloud collisions, both expected in merger environments (e.g., Solomon et al. 1997), can dramatically alter the M(H2) − n(H2) distribution toward most mass being at n(H2) ⩾ 104 cm−3. This actually occurs in the Galactic center (e.g., Güsten & Philipp 2004), and in ULIRGs it can involve their entire molecular gas reservoirs. In such cases, low-J CO, 13CO lines are no longer sensitive to the average ISM conditions and may thus yield inaccurate "corrections" to the global Xco factor with respect to its Galactically calibrated value.
3.1. The Xco in ULIRGs: Theoretical Expectations for Highly Turbulent Gas
The high pressures in the highly turbulent gas disks in ULIRGs (DS98) will (1) result in much larger average gas densities at all scales and (2) "relocate" large mass fractions of their GMCs to densities n(H) > 104 cm−3. The latter can be shown easily from the probability distribution function (PDF) of the density in supersonically turbulent clouds. This is well approximated by a lognormal distribution with a dispersion

where Ms = σv/cs is the average 1 dim Mach number (Padoan & Nordlund 2002). The mass fraction contained in cloud structures with overdensities x ⩾ x○ (x = n/〈n〉) is then given by

The high velocity dispersions σv ∼ (30–140) km s−1 measured in the molecular disks of ULIRGs (DS98; see also Swinbank et al. 2011 for a recently discovered such disk at z ∼ 2.3) versus those in spiral disks (∼(5–10) km s−1) correspond to Ms(ULIRGs) ∼ (3–30) × Ms(spirals). For Ms(spiral) = 10 then σρ(spirals) ∼ 2, while σρ(ULIRGs) ∼ 2.55–3.33 (using Equation 12), which dramatically extends the gas density pdf expected in ULIRGs toward high values where much of the gas mass will now lie. Indeed, for the GMCs in spiral disks only ∼3% of the mass will be found at overdensities of x > x○ = 500 (=5 × 104 cm−3 for typical GMC with 〈n〉 = 100 cm−3), while for ULIRGs the estimate is ∼45% (Figure 6). This difference can be even larger since the average molecular gas density in disks is lower (〈n〉 ∼ (100–500) cm−3) than that in ULIRGs, where it can reach up to at least ∼104 cm−3 (Greve et al. 2009). Thus, gas at n ⩾ 5 × 104 cm−3 corresponds to an overdensity of x○ = 100–500 (containing ≲10% of gas mass) for GMCs in spirals, and only x○ = 5 (and 90% of the mass) for GMCs in ULIRGs. The typical CO SLED available for LIRGs in our sample (J = 1–0, 2–1, 3–2) would then be insensitive to the state of the molecular gas in ULIRGs and thus unable to constrain the corresponding Xco factor, while it would remain adequate for such a task for the molecular gas in isolated spirals.
Figure 6. Gas mass fraction expected at overdensities x ⩾ x○, where x = n/〈n〉 (see Equation (13)) in supersonic turbulent gas, from spiral disks (Ms = 10) up to ULIRGs (Ms = 300).
Download figure:
Standard image High-resolution imageInterestingly, the few cases of near-Galactic Xco in ULIRGs were found when either higher-J CO lines were available or when a dense and warm gas phase was massive enough to be obvious even in low-J CO SLEDs (Paper I and Section 4). The Xco for such dense gas can be high, approaching and even surpassing Galactic values. Indicatively, for n = 5 × 104 cm−3 and warm gas with a thermalized CO J = 1–0 line Tb, 1–0 ∼ Tkin ∼ (100–150) K, it is Xco ∼ (4–6)K−1vir Xl (Equation (3)), which for self-gravitating gas (Kvir = 1) corresponds to Galactic values.
Moreover, for the highly turbulent, high-pressure ISM environments in ULIRGs, the notion of molecular gas reservoirs reducible to ensembles of discrete GMCs may no longer apply, with much of the gas in the pre-merger GMCs redistributed in continuous disks of ∼(100–300) pc diameter (DS98; Sakamoto et al. 2008). A warm, low-density, molecular gas phase with large Kvir (>10) can then be generated alongside the denser one by the tidal disruption of GMC "envelopes" in merger environments, the high pressures turning intercloud Cold Neutral Medium (CNM) H i into H2, and the effects of supersonic turbulence driven at the largest scales (e.g., Ossenkopf 2002). Such a diffuse phase, even if it contains little mass, can easily dominate the emergent global CO J = 1–0, 2–1 line emission of ULIRGs, yielding "cold" CO(2–1)/(1–0) ratios and low Xco factors (a result of its low densities, high temperatures, and large Kvir values). These low Xco values, while appropriate for the diffuse gas mass, may not be appropriate for the bulk of the molecular gas that now resides at much higher densities.
Thus, in the very turbulent molecular gas reservoirs of ULIRGs, a combination of (1) most of their mass residing at high densities (with potentially large Xco factors) and (2) the existence of a diffuse, warm, and unbound phase with low mass (and low Xco) dominating low-J CO SLEDs (and the average Xco determined from them) can easily result in a systematic underestimate of their total molecular gas mass. All current observational studies likely suffer from this bias, which is expected to be most prominent in ULIRGs with high molecular gas surface densities. This includes the present study, as mostly low-J CO SLEDs (up to J = 3–2) are typically available per LIRG. The only exceptions are the few ULIRGs for which a highly excited CO J = 3–2 line, CO J = 4–3, 6–5 lines, and/or HCN lines from the literature allowed the revelation of their massive dense gas reservoirs (see Section 4). Studies of Xco in similar systems at high redshifts (e.g., Tacconi et al. 2008) will be similarly affected. Only molecular SLEDs with high critical densities can overcome such an Xco bias in ULIRGs to reveal how many actually lie on the high end of the Xco distributions shown in Figures 1 and 4.
3.1.1. Numerical Simulations and the Xco Factor: Important Caveats and Ways Forward
Recently, GMC-sized numerical simulations that include H2 and CO formation/destruction and radiative transfer in MHD turbulent models were used to explore Xco and its dependence on average ISM conditions (Shetty et al. 2011a, 2011b). These simulations include chemistry and thermodynamical effects but not SF-driven heating (via photons or CRs) or turbulent heating, which could be dominant in ULIRGs. Currently, such studies explore Galactic-type GMCs whose Xco values they found to be similar to the one observed. Extending such numerical models to ISM conditions expected in ULIRGs is not straightforward, as GMC boundary conditions such as ambient radiation fields, surface pressure, external gravitational field, and its tidal terms (important for galactic centers of spirals and ULIRGs) are either set to be constant in GMC-sized simulations or, in the case of tidal fields, are omitted altogether. Nevertheless, in any realistic setting of GMCs in galaxies these boundary conditions do change, sometimes more quickly than internal cloud evolutionary timescales, especially so in ULIRGs.
Galaxy-sized numerical studies of Xco in disks and mergers recently presented by Narayanan et al. (2011) address the aforementioned important point of coupled GMC–galaxy evolution by tracking the evolution of GMC boundary conditions, but do so by necessarily adopting some subresolution methods to follow in-cloud physics and keep the problem computationally tractable. They recovered Galactic Xco values for disks, but ∼5–10 times lower ones in mergers, with a large spread that makes a single ULIRG-appropriate Xco factor impractical. Nevertheless, for ULIRGs in particular, these simulations do not have (and could not have) the resolution necessary to track gas with n ⩾ 104 cm−3 (where most of their gas mass resides). Resolving the all-important kinematic state of such a dense gas component (self-gravitating or not?) within their compact gas reservoirs (∼(100–300) pc) is currently impossible with galaxy-sized simulations, as is the explicit tracking of the large turbulence-regulated range of densities expected in ULIRGs. There the average densities can surpass ∼104 cm−3, while the high levels of turbulence will maintain ≳60% of the mass at n ⩾ 105 cm−3 (Figure 6). With the Tkin values computed in such simulations likely to be lower limits (as CR and mechanical supernova remnant (SNR) driven heating are omitted), gas densities and the corresponding kinematic states remain the only parameters affecting the average Xco in metal-rich environments that can push its values either way. Indeed, while additional heating can only raise Tkin and thus the CO J = 1–0 brightness temperature (reducing Xco), high densities (>104 cm−3) and self-gravitating states (Kvir ∼ 1) will act to raise Xco.
GMC-sized simulations of the much more turbulent and denser molecular gas in ULIRGs are necessary for computing the corresponding Xco, but these must now use new initial conditions with (1) much higher volume-averaged gas densities (current ones use ∼150 cm−3), (2) higher velocity dispersions (current ones use σv ∼ 2.4 km s−1), and (3) higher background CR energy densities (and thus non-negligible CR gas heating). Such simulations and their emergent line intensities can be compared to the much richer molecular SLEDs available now than in the past. These include the typical CO lines from J = 1–0 up to J = 4–3 observed from the ground, rising up to J = 13–12 using the HSO (van der Werf et al. 2010), further complemented by multi-J observations of heavy rotor molecules like HCN, HCO+, CS (e.g., Greve et al. 2009) as sensitivities vastly improve in the era of ALMA. Thus, rather than simply trusting the Xco yielded by GMC-sized simulations as is currently done, these simulations will yield Xco values as by-products of the much more difficult task of reproducing relative strengths of molecular line luminosities with critical densities ranging from ∼100 cm−3 up to ∼107 cm−3. These encompass the entire range of hierarchical structures in turbulent GMCs, from a highly turbulent low-density possibly non-self-gravitating "GMC-envelope" phase at large scales up to self-gravitating compact dense gas cores with dissipated supersonic turbulence (e.g., Ossenkopf 2002). GMC-sized numerical simulations exploring a dense grid of ULIRG-type GMC boundary conditions and determining the corresponding Xco grid can then be used to inform galaxy-sized numerical simulations where such boundary conditions are tracked, while resolving the hierarchical structures of individual GMCs (and thus determining f(x○) from the simulations) remains out of reach.
3.2. High Xco in ULIRGs: Past Observational Evidence Ignored?
As discussed in the previous sections, by relying on low-J CO SLEDs, most observational studies of Xco may have imparted a bias toward low values in ULIRGs. Nevertheless, past observations of heavy rotor molecules such as HCN J = 1–0 have given early hints of a large-scale M(H2) − n(H2) redistribution in these merger systems, which placed large fractions (≳50%) of their total molecular gas mass at much higher densities (⩾104 cm−3) than that in isolated gas-rich disk systems (e.g., Solomon et al. 1992; Gao & Solomon 2004). However, a single transition of a heavy rotor molecule cannot set constraints on the gas temperature and density (let alone on its average dynamic state) useful enough to constrain well the corresponding Xmol factor. Thus, these early estimates of the dense molecular gas mass in ULIRGs are necessarily very uncertain, as they relied on an XHCN factor derived for n ∼ ncrit[HCN(1–0)], self-gravitating gas, and HCN J = 1–0 brightness temperatures typical of the IR color temperatures of SF galaxies (e.g., Gao & Solomon 2004). Indicatively for n(H2) = 5 × 104 cm−3, Tb, 1–0(HCN) = 40 K, and Kvir = 1, Equation (3) applied for HCN J = 1–0 yields XHCN = 15 Xl, which can be higher still for higher gas densities, as indicated by multi-J HCN, CS, and HCO+ line observations of Arp 220 and NGC 6240 and where XHCN ∼ (20–35) Xl (Greve et al. 2009).
For such high XHCN values and L'HCN, 1–0/L'co, 1–0 = 1/4–1/6 observed in ULIRGs (e.g., Solomon et al. 1992; Gao & Solomon 2004; Garciá-Burillo et al. 2012), it is easy to show that X(2 − ph)co (Equation (10)) will yield near-Galactic or higher values in such systems. Indeed, assuming that the only contribution to the (h)-phase in Equation (10) comes from the HCN-bright gas (i.e., the HCN emission from the low-excitation (l)-phase is negligible), we can determine ρ(l − h)co from

where r(obs)CO/HCN = L'co, 1–0/L'HCN, 1–0 in ULIRGs(=4–6) and r(h)CO/HCN is equal to that of the (h)-phase. The latter can be found using a radiative transfer model for densities typical of HCN-bright gas, an assumed gas temperature, and setting K(h)vir = 1. For n = 105 cm−3 and Tkin = 100 K using an LVG model for HCN and CO lines, we compute Tb, HCN1–0 = 41 K and T(h)b, co1–0 = 96 K. Thus, r(h)CO/HCN = 2.34, while XHCN ∼ 20 Xl and X(h)co ∼ 8.6 Xl, with ρ(l − h)co ∼ 0.71–1.56. Setting X(l)co = 0.5 Xl (Figures 1 and 4) for the low-excitation (CO-bright but HCN-dark) phase, we obtain X(2 − ph)co ∼ (4–5) Xl, i.e., Galactic values. These are now due to much higher fractions of dense HCN-bright gas than in the Galaxy, with large XHCN (and X(h)co). If such X(2 − ph)co values are the norm in the high-pressured turbulent gas disks of ULIRGs, their dynamical masses would be dominated by the molecular gas, quite unlike isolated SF spirals. Indeed, for all the ULIRGs in the DS98 study whose dynamic mass is reasonably well constrained (with both CO 1–0 and 2–1 imaging), Galactic Xco values would yield Mgas/Mdyn ∼ 0.6–1, compatible with the obvious limitation of Mgas/Mdyn ⩽ 1 within the observational uncertainties of Mdyn estimates. Regarding the latter, it is worth pointing out that the low signal-to-noise ratio (S/N) for extended CO line emission regions in current interferometric maps as well as dynamically unrelaxed, non-circular, molecular gas motions (expected in strong mergers) typically cause underestimates rather than overestimates of the actual Mdyn. Surprisingly, such a bias was deduced even for gas-rich but otherwise isolated disks with numerical simulations showing an observationally determined Mdyn to be typically ∼30% less than the actual value (Daddi et al. 2010a). Needless to say, this state of affairs becomes worse still in CO-imaged systems at high redshifts.
Multi-J observations of heavy rotor molecules and/or high-J CO lines in ULIRGs can produce dense gas mass estimates via a (radiative transfer model)-constrained Xmol factor. However, the few such studies available (Papadopoulos 2007; Papadopoulos et al. 2007; Krips et al. 2008; Graciá-Carpio et al. 2008; Greve et al. 2009) are a testimony to how hard it is to overcome the degeneracies inherent in radiative transfer models of optically thick line emission from heavy rotor molecules. The n–Tkin solution degeneracies of the LVG radiative transfer models typically used in such studies translate to a wide range of XHCN factors, ranging from ∼10 Xl and reaching up to ∼(50–90) Xl (Krips et al. 2008; Graciá-Carpio et al. 2008). Tellingly, even the two studies with the largest numbers of dense gas tracer lines available per ULIRG, those of Mrk 231 (Papadopoulos et al. 2007) and of Arp 220, NGC 6240 (Greve et al. 2009) cannot overcome such degeneracies, yielding LVG solutions that produce XHCN ∼ (10–20) Xl and ∼(17–37) Xl, respectively. Nevertheless, most XHCN values deduced in such studies are high enough to yield dominant amounts of dense gas mass in ULIRGs, with a near-Galactic or even larger than Galactic X(2 − ph)co.
This apparent contradiction of the low Xco factor advocated for ULIRGs by past low-J CO line studies (e.g., Solomon et al. 1997; DS98; Yao et al. 2003), and the larger effective X(2 − ph)co implied by heavy rotor molecular line emission (mostly HCN) in such galaxies has not been noted much in the literature. In the rare cases where this discrepancy has been discussed, the argument went for a downward revision of XHCN by ∼1/5 rather than a boost of the widely adopted ULIRG value of Xco ∼ 1 Xl (e.g., Papadopoulos et al. 2007). It must be noted, however, that the possibility of HCN-based molecular gas mass estimates raising the effective Xco in ULIRGs is mentioned in the seminal interferometric work by DS98.
3.2.1. What Could Lower Xco in ULIRGs
Lower XHCN values than those reported in existing studies are possible if higher gas temperatures and/or unbound gas motions (Kvir > 1) prevail for the bulk of the dense gas in ULIRGs. In the most detailed such study, Greve et al. (2009) do find warm and non-virial LVG solutions for the HCN-bright gas in Arp 220 and NGC 6240 with n(H2) = 3 × 105 cm−3, Tkin = (45–120) K (Arp 220), and Tkin = (60–120) K (NGC 6240), while Kvir ∼ 2, 5 for Arp 220 and NGC 6240, respectively. Choosing Tkin = 100 K as indicative for both systems (for a given density and Kvir high Tkin's yield small Xmol's), we find Tb, HCN1–0(Arp 220) ∼ 60 K, T(h)b, co1–0(Arp 220) ∼ 96 K, and Tb, HCN1–0(NGC 6240) ∼ 40 K, T(h)b, co1–0(NGC 6240) ∼ 86 K (for their corresponding Kvir values). Then, from Equation (3): XHCN ∼ 12 Xl, X(h)co ∼ 7.6 Xl for Arp 220 and XHCN ∼ 7.3 Xl, X(h)co ∼ 3.4 Xl for NGC 6240. Thus, using r(obs)CO/HCN = 5.9(Arp 220), 12.5(NGC 6240), and the LVG-computed: r(h)CO/HCN ∼ 1.6(Arp 220), 2.15(NGC 6240), we find ρ(l − h)co ∼ 2.68(Arp 220), 4.81(NGC 6240). Then, from Equation (10) and the aforementioned numbers (and setting X(l)co = 0.5 Xl), it is X(2 − ph)co(Arp 220) ∼ 2.4 Xl and X(2 − ph)co(NGC 6240) ∼ 1 Xl.
In the aforementioned example, the warm "end" of a degenerate range of LVG solutions to a multi-J heavy rotor molecular line data set yields an Xco ∼ (1/5)Xco, Gal for NGC 6240, while reducing that of Arp 220 to ∼ (1/2)Xco, Gal. Nevertheless, such sets of solutions, with Kvir > 1 and Tkin significantly higher than Tdust, are not typically considered optimal in most studies. Indeed, Kvir ∼ 1 is often used as a constraint on the LVG solution range of molecular lines tracing dense gas (e.g., HCN, CS), while solutions with Tkin ∼ Tdust are considered to be more appropriate for such a gas component since gas-dust thermal coupling is expected to be strong (e.g., see the dense gas model in Mrk 231 by Papadopoulos et al. 2007). On the other hand, recent theoretical and observational work has shown that in ULIRGs large temperatures with Tkin > Tdust are possible even with most of the gas mass at high densities (n ⩾ 105 cm−3) because of dominant CR and/or turbulent heating, both powered by the large SNR number densities in these galaxies (Papadopoulos 2010c; Paper I; Rangwala et al. 2011). Nevertheless, while high Tkin certainly contributes to lowering X(h)co of the dense gas phase, it is the high Kvir values that are mostly responsible for this, a result also noted by previous studies (e.g., DS98). Indeed, setting Kvir = 1 for the aforementioned LVG solutions for the dense gas phase in Arp 220 and NGC 6240 brings their X(2 − ph)co factors back up to ∼4.5 Xl(Arp 220) and ∼3.3 Xl(NGC 6240).
Clearly, good constraints on the dynamic state of the dense gas in ULIRGs is of utmost importance, since, while Kvir ∼ 1 may be a safe assumption for the SF-fueling dense gas component of individual GMCs in typical spirals, it may not be for the extreme ISM environments of ULIRGs. Strong tidal fields and/or "bottom"-stirred ISM by strong SF feedback (via multiple SNR shocks and the radiative feedback implied by Eddington-limit-regulated SFRs) could induce Kvir > 1 even for the dense gas in such systems (see Paper I), lowering XHCN and hence the corresponding X(2 − ph)co factor.
3.3. Cold SF-Quiescent Molecular Gas and Large Xco in LIRGs
Gas-rich disks containing a starburst in their central ∼(1–2) kpc but also having large amounts of SF-quiescent ISM at larger galactocentric radii are often found in LIRGs with LIR < 1012 L☉, and such configurations are even expected for weakly perturbed systems (e.g., Maiolino et al. 1997 and references therein). Large amounts of cold (∼(10–15) K) molecular gas beyond a warm component (∼(35–40) K) confined within a star-forming central ∼1 kpc has been shown to be a general feature of LIRGs using CO J = 2–1, 1–0 lines (Papadopoulos & Seaquist 1998). Sensitive dust submm continuum and CO J = 1–0 imaging in individual LIRGs found cold dust and concomitant molecular gas with low CO J = 1–0 brightness extending out to radii of at least ∼3 kpc (Papadopoulos & Seaquist 1999; Papadopoulos & Allen 2000). Submm continuum and H i imaging studies of nearby spirals also suggest cold molecular gas as a general feature of their disks at large galactocentric distances (Thomas et al. 2004).
Extended, SF-quiescent, molecular gas reservoirs in the disks of LIRGs can be undetected because of low CO J = 1–0 line brightness and/or lack of CO in the lower metallicity environments found at large galactocentric distances. Two SF-idle GMCs with n ∼ 102 cm−3 and potentially very low kinetic temperatures (∼5 K) have been found in M 31 (Allen & Lequeux 1993; Loinard et al. 1995; Allen et al. 1995). For Kvir ∼ 1–2 (i.e., self-gravitating or nearly so), such clouds will have T(l)co, 1–0 ∼ 1.4 K and a corresponding X(l)co ∼ 19 Xl. In the global CO SLEDs of LIRGs, such a cold SF-idle component, even if massive, will be completely inconspicuous, outshined by the much more CO-luminous SF molecular gas where typical T(h)co, 1–0 ⩾ 15 K. The global Xco of an LIRG, computed using models of its global CO SLED, will then invariably be biased by the average ISM properties of its central starburst, often having a genuinely low X(h)co ∼ (1/3–1/5)Xco, Gal factor (e.g., Papadopoulos & Seaquist 1999; Papadopoulos & Allen 2000). Thus, its adoption for the entire LIRG can greatly underestimate the contribution from a massive, cold, SF-quiescent component where a Galactic Xco applies, as indicated by submm, H i, and CO J = 1–0 imaging for such galaxies.
We can compute an indicative X(2 − ph)co in such a LIRG for a given ρ(l − h)co by setting X(l)co = Xco, Gal for the cold, extended, SF-quiescent component, and X(h)co = (1/5)XGal, co for the warm, nuclear, star-forming one. Using the results by Papadopoulos & Seaquist (1998), where "stacked" CO(2–1)/(1–0) ratios for LIRGs were used to determine a generic warm versus cold molecular gas distribution in their disks (their Figure 6), we set Lh ∼ 0.9 kpc as the diameter of the warm SF component and Ls ∼ 20 kpc as that of the entire CO-bright gas distribution in the "average" LIRG. With a warm/cold CO brightness temperature ratio of tb = T(h)co, 1–0/T(l)co, 1–0 ∼ 3, it is ρ(l − h)co = (1/tb) × [(Ls/Lh)2–1] ∼ 164. Thus, X(2 − ph)co ∼ X(l)co = Xco, Gal, i.e., it is dominated by the cold disk component. Given that ρ(l − h)co can vary greatly among LIRGs, it is instructive to also obtain X(2 − ph)co in a LIRG where the relative distributions of the warm SF versus the cold SF-idle gas are well known. For the Sy2 NGC 1068: Lh ∼ 2.7 kpc and Ls ∼ 6 kpc (Papadopoulos & Seaquist 1999), while tb ∼ 5. Thus, ρ(l − h)co ∼ 0.79 and X(2 − ph)co ∼ 0.6Xco, Gal, which is ∼3× higher than X(h)co (which would be deduced from its global CO SLED).
3.4. Molecular Gas Inventories in LIRGs: Critical Observations
Highly turbulent molecular gas in the merger-driven starbursts of ULIRGs and extended, cold, SF-quiescent gas in the disks of isolated or slightly perturbed LIRGs represent the two extremes where current CO line studies may systematically underestimate the total molecular gas mass. In both cases, global CO SLEDs often show a low-excitation component, seen as small CO J = 1–0, 2–1 line flux "excess" (often with a subthermal CO (2–1)/(1–0) ratio) on top of the dominant CO line emission of a warm and dense SF gas phase with high CO line excitation to much higher J levels. In ULIRGs, such a diffuse low-excitation phase is typically unbound, warm, and contains little mass. In the disks of LIRGs, it consists of SF-quiescent, gravitationally bound (or nearly so), Galaxy-type GMCs extending beyond a central starburst. In the latter case, spatially resolving the CO SLEDs and/or dust emission of the nuclear SF region versus the extended cold disk can yield proper molecular gas mass estimates not dominated by the (usually) low Xco of the CO-bright nuclear SF region. In practice, CO and 13CO J = 1–0, and J = 2–1 imaging of those SF and SF-idle areas of LIRGs is adequate to determine the nature of the residing ISM (SF: r21 ∼ 1, R10, 21 ∼ 10–15, SF-quiescent: r21 ∼ 0.4–0.6, R10, 21 ∼ 3–6), the corresponding Xco values, and the areas over which they apply.
In the upcoming era of ALMA, such spatial separations of SF and non-SF ISM in LIRG disks along with estimates of the corresponding molecular line ratios and Xco values will be straightforward. For ULIRGs, though, the low-density and the high-density gas components can be concomitant or very closely associated, especially if the former is the outcome of the disruption of GMC outer layers. Thus, high-resolution CO observations, even with ALMA, may be unable to separate their distributions and their corresponding CO line ratios, leaving their Xco factors still uncertain. In such galaxies, with the bulk of the molecular gas mass at n ≳ 104 cm−3, observations of rotational transitions of heavy rotor molecules such as HCN are of paramount importance, even without spatial information. For example, a high global HCN/CO J = 1–0 brightness temperature ratio of ∼1/4–1/6 would immediately indicate an unusually high Mdense/Mtot(H2), contrasting the ∼10× lower such ratio in disk GMCs. Then, multi-J observations of heavy rotor molecules can be used to determine the corresponding Xmol, X(h)co factors, and, eventually, Mtot(H2).
High-resolution interferometric imaging of at least two rotational transitions of a heavy rotor molecule (e.g., HCN J = 1–0, 3–2) along with at least one of its isotopologues (e.g., H13CN J = 1–0) remains an invaluable resource for better determining the distribution and mass of the dense gas in the compact disks of ULIRGs. This can be achieved using radiative transfer models of the emergent HCN line emission as a function of position within these disks, as was done earlier for the CO J = 1–0, J = 2–1 interferometric study by DS98. The focus will now be on the dense gas where much of the molecular gas mass resides, while its all-important average dynamic state (i.e., the Kvir) will be determined as part of the modeling. Imaging of the rare isotopologues can greatly reduce the radiative transfer modeling degeneracies affecting Kvir(r) and yield improved constraints on the dense gas surface densities Σdense(r) of the gas disks in ULIRGs. Their total gas mass can then be determined by integrating the resulting Σdense(r) over the best-fit disk models.
Here, we must note that any molecular line observations that involve the [C/13C] isotope ratio such as CO/13CO or HCN/H13CN, while necessary for reducing LVG modeling degeneracies and better constraining Kvir, involve the additional assumption of the [C/13C] abundance ratio (which we assumed to be 50 in our LVG models). The latter can be particularly uncertain in ULIRGs where large CO/13CO line ratios have also been attributed to a higher [C/13C] abundance than that in spiral disks, a result of early starburst ages and/or accretion of relatively unprocessed (by star formation) molecular gas (Henkel & Mauersberger 1993). If enhanced [C/13C] abundances are indeed the norm in ULIRGs, this will have the general effect of increasing the various Xco, XHCN factors since the deduced Kvir values per gas phase can now be lower. This will be so simply because larger [C/13C] abundances rather than low CO line optical depths (and thus high Kvir, see Equation (11) in Paper I) can be also responsible for the observed high CO/13CO line ratios in ULIRGs.
3.4.1. Molecular Gas Mass Estimates of ULIRGs: the Promise of Herschel
In ULIRGs, a massive, warm, and dense gas phase can have a luminous CO SLED that remains prominent up to very high-J rotational transitions. Since J = 1–0, 2–1 transitions can have significant contributions from a low-excitation diffuse gas component containing a small fraction of the total molecular gas mass, this leaves only J = 3–2 and higher-J CO lines as useful probes of the bulk of the molecular gas mass and its properties. The SPIRE/Fourier transform spectroscope (FTS) aboard the HSO can provide access to CO lines from J = 4–3 up to J = 13–12 for local luminous ULIRGs (e.g., van der Werf et al. 2010; Rangwala et al. 2011) and thus yield a critical data set for obtaining better total molecular gas mass estimates in such systems.
Once a fully sampled global CO SLED from J = 1–0 up to J = 13–12 is available, a multi-component analysis can decompose it into a series of gas components, constraining their properties and using them to obtain the corresponding Xco factors. Such multi-phase models could eventually be produced by theoretical advancements in GMC-sized numerical simulations (see Section 3.1.1). Thus, HSO observations of high-J CO lines will allow better inventories of molecular gas mass in ULIRGs, since much of that mass resides in a dense and presumably SF and warm phase. We note, however, that serious degeneracies will remain, especially regarding the all-important dynamic state of each gas component upon which the corresponding Xco factor strongly depends. Multi-J observations of the much fainter (especially in ULIRGs) 13CO isotopologue lines, necessarily using the much larger millimeter/submm telescopes available from the ground (and thus limited by the atmosphere up to J = 6–5, 7–6), are important for reducing those degeneracies and better constraining Kvir or equivalently (for a given density and temperature), the escape probabilities βJ + 1 J per gas component that enter the expressions of the Xco factors (Equations (3) and (6)).
4. PROBING THE EXTREMES: INDIVIDUAL (U)LIRGs, THEIR MOLECULAR GAS, AND XCO
Several (U)LIRGs in our sample merit an individual study either because a larger than average number of available CO lines permits it (see Table 7 in Paper I), and/or because very high line excitation makes their CO SLEDs irreducible to superpositions of star-forming and non-SF molecular gas (see also Paper I for a discussion on the implication about ISM power sources). Finally, there are LIRGs whose global CO(J + 1 − J)/(1–0) ratios suggest the cold SF-quiescent clouds found at large galactocentric distances in the Galaxy and the disk of M 31. Such globally "cold" yet star-forming galaxies are very few, as expected for an IR-selected (and thus SFR-selected) LIRG sample; they also deserve a closer look as they represent the low-excitation range in LIRGs. The detailed model(s) per galaxy and the associated discussion are in the Appendix B, while here we summarize the most important findings.
The extreme range of CO SLEDs found for the molecular gas reservoirs of LIRGs discussed in Paper I is now marked by individual objects. On the high end are galaxies like IRAS 00057+4021, Arp 299, IRAS 12112+305, and others whose extremely high CO line excitation implies large amounts of very dense (∼(1–3) × (104–106) cm−3) and (often) very warm (Tkin ≳ 100 K) gas. The large fd = M(n ⩾ 104 cm−3)/Mtot(H2) deduced for such (U)LIRGs independently recovers, using mid/high-J CO lines, a well-known result for merger-driven starbursts obtained using the HCN/CO J = 1–0 ratio as an fd proxy (Gao & Solomon 2004). These earlier studies (see also Graciá-Carpio et al. 2008; Krips et al. 2008) found ∼10 times higher dense gas mass fractions in ULIRGs than in isolated spirals. Insofar as the dense molecular gas phase closely tracks the dense and warm gas associated with SF sites, the mid-J/high-J CO lines of ULIRGs are also expected to show clear indications of a high fd, as it is indeed found, quite unlike what is expected from typical GMCs (fd, GMC ∼ 0.02–0.03).
Furthermore, the very warm and strongly unbound (Kvir ⩾ 20) states uncovered for the massive dense gas reservoirs of some LIRGs can make their global CO SLEDs surpass even those expected for SF "hot" spots in the Orion A and B clouds. While in some distinct cases this can be due to strong AGN feedback (e.g., Mrk 231), for all other galaxies with such extreme ISM conditions (e.g., IRAS 00057+4021, IRAS 08572+3915, IRAS 23365+3604) the cause is unclear. It remains to be explored whether the much higher SFR densities of the compact SF regions of ULIRGs (e.g., Sakamoto et al. 2008) can create such extraordinary ISM states where turbulence injection by SNRs no longer remains confined in small regions but encompasses much more molecular gas mass (see discussion in Paper I). This along with CRs (also SNR-generated) may set up powerful global mechanisms that can volumetrically heat large amounts of gas, unhindered by the large dust extinctions and high average gas densities that will keep photon-dominated regions (PDRs) very localized around SF sites.
On the low-excitation end only a few cases of low global CO line ratios are found (e.g., IRAS 05189−2524, IRAS 03359+1523). This is expected for IR-selected (and thus SFR-selected) galaxies (see Dunne et al. 2000 on the limitations of such samples in finding (cold-ISM)-dominated systems). In Arp 193, a low-excitation ISM state and the lack of large amounts of dense and warm gas is actually suggested by HCN rather than CO lines. Indeed, despite the presence of a young merger with substantial IR luminosity and a significant HCN/CO J = 1–0 line ratio, its global HCN line emission is consistent with the absence of a dense gas phase, possibly a case of strong SF feedback momentarily dispersing its dense gas supply (Papadopoulos 2007). Such IR-luminous/(dense gas) deficient galaxies will be rare (see discussion in Appendix B.17) and thus valuable for studying the effects of SF feedback on the dense ISM where the initial conditions of star formation are set. The case of IRAS 05189−2524, on the other hand, stands out as one where a massive cold molecular gas-rich disk is implied, but unlike other LIRGs (e.g., I Zw 1) there are no morphological indications whatsoever for the presence of such a disk in this very compact ULIRG/AGN system. Moreover, its "warm" CO(6–5)/(3–2) ratio, warm IR "colors," and compact size in cm, near-IR, and optical wavelengths indicate the presence of only a warm, dense, SF gas phase; thus, this object represents a cautionary tale about such conclusions drawn for similar high-z ULIRG/AGN systems using only high-J CO lines (e.g., Tacconi et al. 2006).
We note that massive cold molecular gas disks are implied for other LIRGs as well (e.g., I Zw 1, VII Zw 031, NGC 7469), but without strongly affecting their global CO SLEDs or CO/13CO line ratios, which remain dominated by the warm SF phase. This simply mirrors, for molecular lines, a result well known for dust continuum emission, though in practice global CO SLEDs are somewhat more sensitive to the presence of cold diffuse gas than the global dust SEDs are to the concomitant cold dust mass (Papadopoulos & Allen 2000).
4.1. Effects of Average ISM Conditions on the Xco
In Table 1, we tabulate the total molecular gas mass estimates as produced by Equations (3) and (10), the corresponding Xco factors, the minimum molecular gas mass implied for Eddington-limited star formation (Section 2.3), and the mass of the high-excitation (h)-phase when a two-component model is used to interpret the CO, 13CO lines (see Appendix B). A mere inspection of the third column demonstrates that in several galaxies (∼40%) significantly larger Xco factors than the so-called (U)LIRG values of ∼(0.6–1) Xl may apply. For ULIRGs, this is due to large and even dominant fractions of their molecular gas mass being at much higher densities than that in disk-dominated LIRGs, while for the latter it is because extended cold molecular disks (with a Galactic Xco) can contain much of the molecular gas mass while remaining inconspicuous in the global CO SLEDs used to constrain the global Xco. For ULIRGs, this low-mass bias includes some very well known systems such as Mrk 231 and Arp 220. For lower IR luminosity galaxies, underestimates of their total molecular gas by the Xco deduced from one-phase radiative transfer models of their CO SLEDs can be important in disk-dominated systems (e.g., NGC 7469) and also in seemingly compact star-forming galaxies that nevertheless have "cold" CO SLEDs and Galactic Xco values (IRAS 05189−2524).
Table 1. Molecular Gas Masses and Xco Factors
Name | Mtot(Xco)a | M(2 − ph)tot(X(2 − ph)co)b | MSFc | Mh − exd | Remarks |
---|---|---|---|---|---|
(109 M☉) | (109 M☉) | (109 M☉) | (109 M☉) | ||
IRAS 00057+4021 | 4.5 (1.1) | 3.4–8.2 (0.83–2.0) | 1.2 | 1.6 | Degenerate X(l)co values |
I Zw 1 | 8.6 (1.5) | 9.5 (1.65) | 0.4 | 2.8 | Galactic X(l)co adopted |
NGC 828 | 4.6–6.3 (0.8–1.1) | 4–14 (0.7–2.5) | 0.3 | 0.3 | Degenerate X(l)co |
IRAS 02483+4302 | 1.6 (0.45) | 4.3–8.6 (1.2–2.4) | 1.4 | 3.3 | Degenerate X(l)co values |
IRAS 03359+1523 | 22 (2.5) | ⋅⋅⋅ | 0.8 | ⋅⋅⋅ | SF-quiescent ISM |
VII Zw 031 | 14.5 (1.25) | 39 (3.4) | 2.0 | 3.1 | Cold extended disk? |
IRAS 05189−2524 | 13.7 (3.5) | 9.4 (2.4) | 3.6 | 3.6 | (h)-phase SLED assumed |
IRAS 08030+5243 | 14.3–42.9 (1.5–4.5) | ⋅⋅⋅ | 2.4 | ⋅⋅⋅ | Degenerate one-phase solutions |
IRAS 08572+3915 | 4.8–9.6 (3–6) | ⋅⋅⋅ | 3.9 | ⋅⋅⋅ | Highly excited CO J = 6–5 line |
Arp 55 | 2.9–9.2 (0.25–0.8) | ⋅⋅⋅ | 0.9 | ⋅⋅⋅ | Degenerate one-phase solutions |
UGC 05101 | 1.9–2.9 (0.4–0.6) | ⋅⋅⋅ | 1.6 | ⋅⋅⋅ | |
NGC 3310 | 0.03–0.15 (0.4–2.2) | ⋅⋅⋅ | 0.1 | ⋅⋅⋅ | Degenerate Xco values |
IRAS 10565+2448 | 3.8–4.8 (0.6–0.75) | 4.8 (0.75) | 2.6 | 2.6 | (h)-phase SLED assumed |
Arp 299 | 1.2 (0.42) | ⋅⋅⋅ | 1.9 | ⋅⋅⋅ | |
IRAS 12112+0305 | 15.2–53.5 (1.5–5.3) | 7.1–34.3 (0.7–3.4) | 4.8 | 4 | Unconstrained X(l)co |
Mrk 231 | 1.8–3.2 (0.25–0.45) | 21–49 (3–7) | 5.5 | 8–25 | CO 6–5, HCN lines used in two-phase model |
Arp 193 | 1.6–3.5 (0.35–0.75) | 3.3–8.1 (0.7–1.8) | 0.9 | 1.7–7.1 | CO 3–2, HCN lines used in two-phase model |
NGC 5135 | 1.1–1.4 (0.35–0.45) | ⋅⋅⋅ | 0.3 | ⋅⋅⋅ | |
Mrk 273 | 10.4 (2.0) | 13 (2.5) | 3.3 | 6 | Cold extended disk? |
3C 293 | 2.3–7.4 (0.5–1.6) | 13.4–18 (2.9–3.9) | 0.05 | 1.1–3.5 | Galactic X(l)co adopted |
IRAS 14348−1447 | 11–17 (0.65–1) | 24–30 (1.4–1.7) | 5.6 | 5.6 | Degenerate (l)-phase |
Zw 049.057 | 0.30–0.52 (0.35–0.6) | ⋅⋅⋅ | 0.47 | ⋅⋅⋅ | |
Arp 220 | 1.85 (0.30) | 14.7–27.5 (2.4–4.5) | 4 | 12.6–25.2 | HCN lines used in two-phase model |
NGC 6240 | 2.5 (0.30) | 8.4–27.8 (1–3.3) | 1.5 | 4.9–24.5 | HCN lines used in two-phase model |
IRAS 17208−0014 | 5.2 (0.40) | 9.8–33 (0.75–2.5) | 6.2 | 3.5–6 | Degenerate X(l)co values |
" " | " | 34–77 (2.6–5.9) | " | 27–70 | HCN lines used in two-phase modele |
IRAS 22491−1808 | 6.3–22 (0.70–2.4) | ⋅⋅⋅ | 5.2 | ⋅⋅⋅ | Degenerate Xco values |
NGC 7469 | 2.5 (0.72) | 6.5 (1.88) | 0.65 | 1.65 | Cold extended disk present |
IRAS 23365+3604 | 7.3–9.5 (1–1.3) | 3.7–22 (0.5–3) | 2.5 | 1.4 | Unconstrained X(l)co |
Notes. aTotal molecular gas mass from Equation (3) and the best one-phase LVG model parameters (the corresponding Xco value in Xl units), with average values adopted in cases of significant LVG solution range degeneracy. bTotal molecular gas mass from Equation (10) and a two-phase fit (the corresponding Xco value in Xl units). cThe minimum molecular gas mass necessary for an Eddington-limited star formation rate (see Section 2.3). dThe gas mass of the (h)-phase in a two-phase model when this is used. If a standard (h)-phase SLED and mass normalization is used (see Section 2.4) then Mh − ex = MSF. eFor LVG solutions with Kvir(HCN) ∼ 1–6 (see Appendix B.24).
Download table as: ASCIITypeset image
The computed X(2 − ph)co and M(2 − ph)tot in Table 1 also make clear that in the case of ULIRGs, global molecular line SLEDs can reveal the aforementioned mass bias, provided that they include mid/high-J CO and/or heavy rotor molecular lines (e.g., HCN). For less IR-luminous galaxies with nuclear starbursts and cold SF-quiescent gas-rich disks, global SLEDs cannot easily identify the presence of the latter except in a few cases (e.g., IRAS 05189−2524). This becomes possible only when additional spatial information (e.g., CO 1–0, submm dust continuum, or cm imaging) is available for such a disk (e.g., NGC 7469). In the absence of such information, even good one-phase models of global CO SLEDs for a (starburst)+(cold disk) system may be unable to reveal the disk component, leaving the deduced average Xco dominated by the starburst phase (e.g., IRAS 02483+4302). In the era of ALMA routine, CO multi-J and 13CO line imaging will provide much better molecular gas mass estimates for disk-dominated LIRGs with strong ISM excitation gradients (see Section 3.4). For ULIRGs, on the other hand, multi-J observations of heavy rotor molecules (e.g., HCN, CS) and their rare isotopologues are necessary for confirming the high total Xco and molecular gas masses implied by our results in Table 1 by constraining the all-important dynamic state of the massive dense gas phase as discussed in Section 3.2.1.
5. CONCLUSIONS
In this work, our large CO, 13CO line survey of LIRGs detailed in Paper I (Papadopoulos et al. 2012) is used to examine the impact of the wide range of average ISM conditions found for these galaxies on their total molecular gas mass estimates via the so-called Xco = M(H2)/L'CO, 1–0 factor. Our sample includes some of the most prominent local ULIRGs (e.g., Arp 220, Mrk 231, IRAS 17208−0014), often used as templates for merger-driven starbursts at high redshifts, as well as less IR-luminous disk-dominated galaxies. We find that one-phase radiative transfer models of the global CO, 13CO line ratios yield 〈Xco〉 ∼ (0.6 ± 0.2) M☉ (K km s−1 pc2)−1, similar to that obtained by past studies. The average gas temperature and density strongly influence Xco, but the average gas dynamic state is the most important influencing factor, with unbound gas corresponding to low Xco values and self-gravitating gas corresponding to higher ones.
Nevertheless, higher Xco ∼ (2–6) M☉ (K km s−1 pc2)−1 values are deduced for (U)LIRGs whenever adequate molecular line data exist to determine the mass contribution of gas at densities n ⩾ 104 cm−3 (high-J CO lines from our survey and/or HCN lines from the literature). Theoretical expectations for the highly turbulent molecular gas in the merger-induced starbursts of ULIRGs indicate that, with most of the gas at such high densities, the aforementioned large Xco values may be the rule rather than the exception in such systems. Past observational studies were unable to determine this, yielding instead much lower Xco values (and often considered as ULIRG-appropriate standard ones), because the molecular lines used (mostly CO J = 1–0, 2–1) could not constrain the properties of the dominant (i.e., the dense) gas phase in ULIRGs. Our results indicate that only high-J CO lines and multi-J observations of heavy rotor molecules (e.g., HCN, CS) can overcome this mass bias, placing the HSO and ALMA front and center in the quest for improved molecular gas mass estimates in ULIRGs in the local and the distant universe. Of particular importance are good constraints on the dynamic state of the dense molecular gas in such systems (self-gravitating or not), since strongly unbound states for this phase seem to be the only possible way that Xco in ULIRGs could be much lower than a Galactic value. Observations of high-density tracing rare isotopologues (e.g., high-J13CO, H13CN) will be of crucial importance in yielding such constraints. On the theoretical front, GMC-sized numerical simulations of the turbulent molecular gas with ULIRG-type boundary conditions are needed in order to obtain the dense gas mass fraction and its temporal evolution. This in turn can inform galaxy-sized simulations of merger systems which recently have included molecular gas but cannot explicitely track the dynamic state and the mass of the dense gas.
We also find LIRGs where underestimates of their total molecular gas are the result of a massive, cold, gas-rich, disk existing beyond their central starburst. In such cases the global CO SLED and the Xco factor determined from it remain dominated by the central starburst and its often low Xco. Spatially resolving the CO, 13CO line and dust continuum emission of such disks in LIRGs with strong ISM excitation gradients is indispensable in accounting for their total molecular gas mass. In such cases, provided that adequate resolution is employed to separate the cold gas disk from the nuclear starburst, even low-J CO and 13CO lines (J = 1–0, 2–1, 3–2) are adequate to yield much improved molecular gas mass estimates.
Finally, our study unfolds the wide range of the global CO SLEDs of LIRGs presented in Paper I over a subsample of individual systems. We find galaxies whose extreme CO SLEDs indicate ISM conditions that surpass those expected for star-forming regions and suggest ISM energy sources other than photons from PDRs (as already discussed in Paper I). These can be AGNs, extreme turbulence, and/or very large CR energy densities. Moreover, we find LIRGs where extreme SF feedback may have momentarily fully disrupted their dense molecular gas reservoirs, and LIRGs where large amounts of cold SF-quiescent molecular gas are present despite the absence of an extended disk, their compact near-IR/cm emission size, and "warm" CO (6–5)/(3–2) ratios. The latter type of objects can be particularly worrying if encountered at high redshifts, where such characteristics can readily lead toward large underestimates of their total molecular gas mass. The wide range of the average ISM conditions and the intriguing possibilities that may lie behind it make our subsample of individually studied LIRGs an excellent target for future ALMA and NOEMA (for the northern objects) molecular line imaging observations leading toward a complete understanding of ISM energetics, AGNs, and SF feedback on the molecular gas in galaxies.
We thank the referee Santiago Burillo for his comments, particularly for bringing to our attention two important issues, namely, the uncertainties of [CO/13CO] abundance ratio in ULIRGs and the reliability of dynamic mass estimates, which resulted in the corresponding clarifications included in this paper. Padelis P. Papadopoulos dedicates this long-coming work to his 10-month-old son Λωνiδα-
, for late night inspirations, and to his wife Mαργαρiτα for her enduring support. The project was funded by the John S. Latsis Public Benefit Foundation. The sole responsibility for the content lies with its authors. Y.G.'s research is partially supported by the China NSF grants No. 11173059, 10833006, and 10621303.
APPENDIX A: THE XCO FACTOR IN AN LVG SETTING
The CO J = 1–0 line luminosity L'CO(1–0) (in K km s−1 pc2, see Equation (5), Paper I) can be re-expressed as
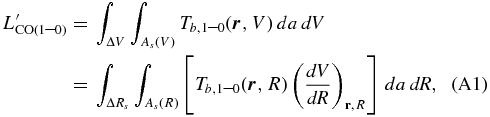
where any given velocity V is assumed to correspond uniquely to a source surface As(V), emitting at . In turn, this can be parameterized by a "depth-in-the-source" parameter R(V) so that these iso-velocity surfaces completely scan the entire source volume for a range ΔRs (which corresponds to the FWZI of the source velocity field) without any radiative coupling (the LVG assumption). The latter implies that the line luminosities emanating from these surfaces simply add up. Thus, we can write

where 〈 · · · 〉 denotes averaging over the entire source volume ΔVs and for an assumed constant velocity gradient. Thus, for
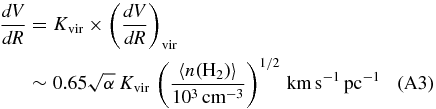
and M(H2) = μm(H2)〈n(H2)〉ΔVs (μ = 1.36 accounts for the He mass), Equations (A1), (A2), and (A3) along with substituting astrophysical units yield for the XCO factor
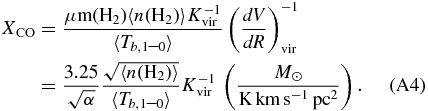
The last expression (used in the main text with the averaging symbols omitted for simplicity) for α = 1.5 becomes identical to that derived by Solomon et al. (1997, their Equation (21) for f = 1). For virialized gas motions (Kvir = 1) and typical conditions in Galactic GMCs with 〈n(H2)〉 = (100–500) cm−3 and Tb, 1–0 = 10 K, this expression yields XCO ∼ (3–6) M☉(K km s−1 pc2)−1, in good accord with the average Galactic value and its uncertainties. Finally, expression (A4) is valid for any optically thick molecular line emission used as a mass tracer of a particular gas phase (e.g., HCN J = 1–0 tracing dense gas with n(H2) > 104 cm−3), while multi-J observations of the particular molecule and its isotopologues can be used to constrain 〈n(H2)〉, Tb, 1–0, and Kvir.
A.1. The Optically Thin Approximation for XCO
From standard formalism, the integrated line luminosity for an optically thin CO J + 1 → J line, omitting the cosmic microwave background for simplicity, is given by

where NJ + 1 is the total number of CO molecules at the J + 1 state and AJ + 1 J is the Einstein coefficient of the J + 1 → J transition. This line luminosity can be re-expressed in terms of L'J + 1 J (in Ll = K km s−1 pc2 units, see Equation (A1)) using

which combined with Equation (A5) yields

The total molecular gas mass is then given by

where RCO = [H2/CO] = 104 is the CO abundance and μ = 1.36 accounts for He mass. The molecule population at the J = 0 level obviously cannot be estimated from a line transition, and we thus compute it from N0 = (g0/g1)exp[E1/(kBTex, 10)]N1, where gJ = 2J + 1 denotes the J-state degeneracy factor, E1/kB ∼ 5.5 K, and Tex, 10 is the excitation temperature of the CO J = 1–0 transition. Substituting this in Equation (A8) yields

which after substituting the expressions from Equation (A7) yields
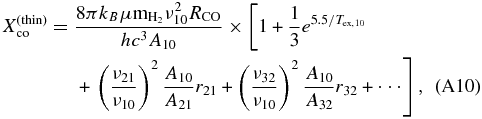
where rJ + 1 J are the observed L'CO(J + 1 − J)/L'CO(1–0) ratios (Equations (5) and (6)). The Einstein coefficients scale as a function of J as

while νJ + 1 J = (J + 1)ν10. Thus, Equation (A10) finally becomes
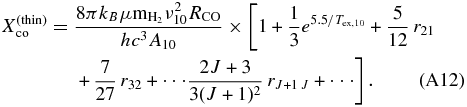
Substituting the various physical constants and introducing astrophysical units yield
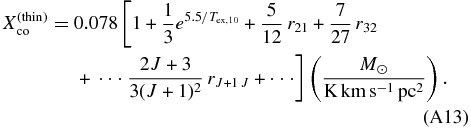
In the literature, the LTE approximation is often used by setting ∑k = 0Nk = ZLTE/g1N1 and ZLTE ∼ 2(kBTk/E1) = 2[Tk/(5.5 K)] in Equation (A8), which then yields

For optically thick CO emission where the optical depths arise in small gas cells with respect to molecular cloud sizes, CO line emission remains effectively optically thin (i.e., it still traces the entire emitting gas mass) but with Aik → βikAik in all the previous equations, with βik = [1 − exp (− βik)]/τik being the photon escape probability (for a spherical cloud). In such a case, Equation (A10) can be trivially modified for finite CO line optical depths to yield
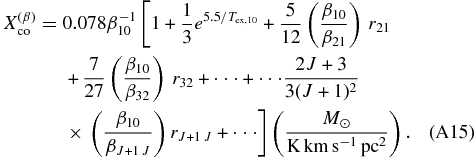
APPENDIX B: MODELS OF INDIVIDUAL LIRGs
Here, we describe the radiative transfer models in selected galaxies from our sample. The details of the LVG radiative transfer code used and the parameter space explored by its [Tkin, n, Kvir] variables can be found in Paper I. In the several cases where a one-phase gas LVG model manifestly fails to reproduce the available line ratios, we use a two-phase approximation (see Section 2.4) to better represent the underlying average molecular gas properties, and we compute the corresponding X(2 − ph)co (Equation (10)). The (h)-phase properties and its X(h)co are constrained using CO J = 4–3, 3–2, and 13CO lines, with the 6–5 transition placing constraints as a lower limit of the true emergent line luminosity (see Paper I), with higher CO J = 6–5 luminosities typically yielding even denser gas LVG solutions with larger X(h)co. The "residual" CO line ratios (determined from Equations (8) and (9)) are used as inputs into our LVG radiative transfer code in order to constrain the physical properties and corresponding X(l)CO factor of the low-excitation (l)-phase. In the cases where a two-phase ISM model is necessary but CO line observations are inadequate to constrain the (h)-phase, we assume one based on the model defined by the Orion star formation "hot spots," their SLED, and molecular gas mass normalization (see Section 2.4). Finally, in the very few cases where heavy rotor molecular lines (mostly HCN transitions) exist, they are often used to determine the (h)-phase. For all the LIRGs studied, their measured CO line ratios, computed SF-powered IR luminosities L(*)IR, and the dust temperatures used to constrain the LVG model (i.e., Tkin/Tdust ≳ 1) can be found in Paper I.
B.1. IRAS 00057+4021
There are only a few studies of this LIRG, and the only available CO J = 1–0 interferometric map shows a very compact nearly face-on source with most of the gas in a ≲ 1'' (∼864 pc) core (DS98). Its luminous CO J = 4–3 line emission cannot be accounted for by one-phase LVG models, with the best such model: Tkin = 90 K, n(H2) ∼ 3 × 102 cm−3, Kvir = 1, reproducing well the observed r21 and r32 and the R21 lower limit, but yielding r(lvg)43 = 0.48, which is ∼1/3 of the observed value. Moreover, the fact that r43 > r(h)43(Orion) (see Section 2.4) and r43 > r32 makes obvious that a superposition of low-excitation (SF-quiescent) and high-excitation Orion-type SF "hot" spots cannot produce such a highly excited global CO SLED, and that even extreme GMCs consisting solely of such "hot" spots are inadequate. Thus, the average state of the molecular gas in IRAS 00057+4021 "out excites" even the SF regions in Orion A and B. The existence of such ULIRGs and the implications for an ISM energy input other than far-UV/optical/IR photons from SF sites (i.e., turbulent and/or CR heating) have already been noted in Paper I (see also Rangwala et al. 2011).
Using r43 − σ = 0.89 along with the other CO line ratios still does not yield an average ISM state that can reproduce them. The best LVG solution ranges are then Tkin = (35–65) K, n(H2) = 103 cm−3, Kvir ∼ 1–4, and Tkin = (70–150) K, n(H2) = 3 × 102 cm−3, Kvir ∼ 1. These reproduce the observed r32 and r21 and the R21 lower limit, but yield r43 (lvg) ∼ 0.45–0.50, which is still significantly lower than r43 − σ. A two-phase model using an Orion-defined (h)-phase (and SLED) gives r(l)21 = 0.91, r(l)32 = 0.53, and r(l)43 = 0.83 (Equations (8) and (9)) and remains unable to fit these residual line ratios, failing to account for the J = 4–3 line (r(l)43 (lvg) ∼ 0.3–0.4). Using only the J = 3–2, J = 4–3 transitions as constraints (r43/32 = 2.23 ± 0.97) of the (h)-phase and restricting LVG solutions with Tkin ⩾ Tdust(=37 K) and Kvir ⩾ 1 yields Tkin ≳ 95 K, n(H2) = 3 × 104 cm−3, and Kvir ∼ 20. The fit yields no upper limit on Tkin, as expected since the maximum possible r43/32 (attained for optically thin thermalized CO lines) is (4/3) for 16.6/Tkin → 0. Using the lower r43/32 = 1.26 value (=(observed)−σ) as input in the LVG code yields identical results. Thus, a very warm, dense, and strongly kinematically stirred gas phase is present in IRAS 00057+4021. An estimate of its mass can be obtained from the measured L'CO(4–3) = (5.84 × 109) Ll and
× L'CO(4–3), where 〈r(h − ex)43〉 = 3.4 is the average CO(4–3)/(1–0) ratio computed from the range of LVG solutions compatible with the observed one. Thus, for
= (1.72 × 109) Ll and X(h)co ∼ 0.92 Xl (computed from the LVG solutions compatible with r43/32), it is Mh − ex(H2) ∼ 1.6 × 109 M☉, comparable to the total (H i + H2) gas mass of the Milky Way and the minimum MSF(H2) = L(*)IR/
g, * ∼ 1.2 × 109 M☉ expected for "fueling" star formation in this LIRG. In Table 1, we comprehensively list all these gas mass estimates for IRAS 00057+4021, along with Mtotal(H2) computed using one-phase and two-phase LVG models (Equations (3) and (10)). We must note, however, that for IRAS 00057+4021 and most (but not all) LIRGs where two-phase models are used, the large degeneracy that exists for LVG solutions of the "residual" CO line ratios of the (l)-phase (cool and near-virial versus warm and strongly gravitationally unbound states) translates to a large range of X(l)co, and thus X(2 − ph)co, values (Table 1). This in effect is the low-J CO SLED degeneracy described in Section 3.4, which the global CO SLEDs available in our study cannot "break."
The high-excitation (h)-phase in IRAS 00057+4021 contains ∼(20–50)% of Mtot(H2) (Table 1), which is at least ∼10× larger than what would be expected if its ISM were reducible to typical Galactic GMCs. Moreover, its r(h)21 (lvg) ∼ 2.5, R(h)21 (lvg) ∼ 23 ratios (for Tkin = 100 K, n(H2) = 3 × 104 cm−3, and Kvir = 22) are higher than those in the Orion SF "hot spots" near H ii regions and O, B star associations (Sakamoto et al. 1994). This further corroborates the irreducibility of the observed CO SLED of IRAS 00057+4021 to ordinary photon-powered ISM states. On the other hand, strong mechanical feedback from SNR-driven shocks could in principle yield such highly excited CO SLEDs (Arikawa et al. 1999; Bolatto et al. 2003), but just like radiative feedback from O, B stars, such SNR-driven "hot" spots involve only small fractions of a typical GMC mass. Indicatively, in the Galactic SNRs W 44 and IC 443, a warm, dense, and very kinematically stirred component with r21 ∼ 1.3–4 (Seta et al. 1998) involves only ∼1% of the impacted GMCs, with negligible effect on their global CO line ratios (〈r21〉GMC ∼ 0.6). We must also note, however, that IRAS 00057+4021 is a Seyfert 2 and its AGN, if X-ray luminous, can induce such extreme conditions to a large molecular gas mass, if distributed close to it (Schleicher et al. 2010).
B.2. IRAS 00509+1225 (I Zw 1)
This LIRG hosts an optically and X-ray luminous QSO in the center of a molecular gas-rich disk, the site of a vigorous circumnuclear starburst (Barvainis et al. 1989; Eckart et al. 1994 and references therein). The latter has been imaged interferometrically (Schinnerer et al. 1998; Staguhn et al. 2004), revealing a disk of ∼8–12 (∼12–14 kpc) with cold ISM but increasing ISM excitation toward its central ∼1.5 (∼2 kpc) SF circumnuclear ring.
In terms of global CO line excitation, while its r21 = 0.84 ± 0.24 ratio can be (barely) compatible with that of an SF-quiescent disk like the Milky Way (∼0.6), its luminous J = 3–2 line with r32 = 1.16 ± 0.40 is clearly dominated by much higher ISM excitation, which is typical of an SF phase (even r32 − σ = 0.76 is 2.5× higher than r32 = 0.3 typical for SF-quiescent ISM). A one-phase LVG model yields an acceptable fit within the measurement uncertainties, with r21 (lvg) ∼ 1, r32 (lvg) ∼ 0.90–0.95, and R10 ∼ 8, R21 ∼ 5–7, while Kvir ∼ 1–2 (a result of the modest R10 and R21). The best one-phase LVG solutions yield Xco ∼ 1.5 Xl (Equation (3)) and a total molecular gas mass M(H2) ∼ 8.5 × 109 M☉. The minimum dense gas mass fueling an Eddington-limited SF is MSF(H2) = 4 × 108 M☉, which is only ∼5% of the Mtot(H2) and typical for ordinary SF GMCs found in galactic disks. Thus, I Zw 1 stands as an example of an LIRG with star formation occurring in an isolated gas-rich disk, fueled by ordinary molecular clouds.
The reasonable one-phase LVG fit of the observed global CO line ratios in I Zw 1 makes a two-phase decomposition unnecessary. Nevertheless, the available observational information on the presence of a cold molecular gas-rich disk and a circumnuclear ring starburst allow such a decomposition as a useful test of the limitations of global CO SLEDs and their fit by a single average ISM state, to yield reliable total molecular gas mass estimates. Using the CO J = 3–2, 6–5, and 13CO J = 1–0 lines to constrain the (h)-phase, we find the best LVG solution range at Tkin = (65–75) K, n = 104 cm−3, and Kvir = 13 that yield X(h)co = 0.65 Xl. Adopting a Galactic X(l)co = 5 Xl for the computed residual CO J = 1–0 emission, which we attribute to the extended gas disk, yields X(2 − ph)co = 1.65 Xl (Equation (10)), similar to that computed from the one-phase LVG model. We caution, however, that the CO J = 6–5 line luminosity, and along with it the current two-phase decomposition, is highly uncertain.
B.3. NGC 828
This is a disturbed spiral galaxy with a prominent dust lane and Hα emission in its center and two sources symmetrically around the center source along the major axis (Hattori et al. 2004). Very Large Array imaging of its cm continuum emission shows it is elongated along the disk, as would be expected for star formation, with no signs of AGN activity such as a radio core or jets (Parma et al. 1986). Its single-dish CO J = 1–0 detection showed abundant molecular gas (Sanders et al. 1986), while subsequent interferometric CO 1–0 imaging with OVRO recovered all the single-dish flux and showed it to be extended ∼10'' × 20'' (∼7.5 kpc × 3.6 kpc), with the longest dimension lying along the optical disk (Wang et al. 1991). The same study obtained a dynamic mass of Mdyn ∼ 4 × 1010 M☉ within a CO disk radius of 3.9 kpc (for the adopted cosmology). However, at ∼4'' (1.4 kpc) southeast of its nucleus, the CO-derived velocity field deviates from that of a normal rotating spiral, possibly indicating the presence of another molecular gas concentration brought in as part of a merger (Wang et al. 1991).
The available CO lines are consistent with a star-forming disk with a well-excited CO J = 3–2 line (r32 = 0.70 ± 0.15; Paper I) but whose modest R10 = 10 ± 2 and R21 = 12 ± 3 ratios indicate that any merger activity has not disturbed the average dynamic state of the molecular clouds. This is reflected in the one-phase LVG solutions found for Tkin ⩾ 35 K, which invariably have Kvir ∼ 2–4. These solutions remain highly degenerate, however, with Tkin = (35–50) K, n = 103 cm−3, and Kvir ∼ 4 almost as good as Tkin = (55–150) K, n = 3 × 102 cm−3, and Kvir ∼ 2. The corresponding Xco ∼ (0.8–1.1)Xl yields Mtot ∼ (4.6–6.3) × 109 M☉, while MSF ∼ 3 × 108 M☉ is necessary to fuel an Eddington-limited star formation.
Thus, the dense and warm gas fueling SF sites in this low IR luminosity galaxy will amount to only ∼5%–6.5% of the total molecular gas mass, which is also consistent with its small HCN/CO J = 1–0 ratio of r(obs)HCN/CO = 0.022 (Gao & Solomon 2004). Nevertheless, global CO SLED can still be easily dominated by even small amounts of star-forming gas whose often modest Xco may be much smaller than that of an inconspicuous SF-quiescent cold gas reservoir. For NGC 828, there is a priori knowledge of an extended molecular gas disk with ∼8 kpc diameter. However, a two-phase fit with an assumed (h)-phase SLED and mass normalization (see Section 2.4) leaves the (l)-phase properties largely undetermined with X(l)co ∼ (0.7–2.5) Xl (∼X(2 − ph)co, since the (l)-phase CO J = 1–0 luminosity dominates) and Mtot ∼ (4–14) × 109 M☉.
B.4. IRAS 02483+4302
This two-nuclei system is a gas-poor/gas-rich merger of an elliptical (nucleus A) going through the disk of a former spiral containing nucleus B (Kollatschny et al. 1991) and all of the molecular gas detected in a CO J = 1–0 interferometer map (DS98). The optically bright nucleus A hosts an AGN with a Sy2 spectrum and is located 38 (∼3.76 kpc) to the west of nucleus B. The latter is where star formation occurs in this system, "activated" by the merger event that also likely triggered the QSO activity in the gas-poor merger progenitor.
Its CO line ratios up to J = 3–2 can be well fitted by a wide range of conditions with even a cold Tkin = 15 K but highly unbound (Kvir = 22) phase yielding a good fit. Most solutions are found at warmer temperatures, though, with Tkin = (35–55) K and Tkin = (90–120) K, low densities of n ∼ (3 × 102–103) cm−3, and strongly unbound states Kvir = 7–40. Such a wide degeneracy of LVG solutions representing the average conditions of the molecular gas in IRAS 02483+4302 is typical for LIRGs where rJ + 1, J < 1, and no CO/13CO ratios are available to constrain line optical depths (and thus Kvir). For this LIRG, a lower limit of R10 ≳ 13 is not enough to eliminate the aforementioned degeneracy, though it does preclude SF-quiescent and virialized states. For all the LVG solutions compatible with its global line ratios, we obtain Xco = (0.25–0.70) Xl (Equation (3)). Restricting the admissible range of LVG solutions with Tkin/Tdust ≳ 1 and Kvir ≲ 20 yields Xco ∼ 0.45 Xl and a total molecular gas mass M(H2) ∼ 1.6 × 109 M☉, similar to that obtained using the optically thin, LTE, approximation M(LTE)thin ∼ 2 × 109 M☉ (Equation (5) for Tkin = 55 K), a result of the low/moderate optical depths of the LVG solutions used to deduce Xco in this LIRG.
The minimum gas mass for an Eddington-limited star formation in IRAS 02483+4302 is MSF(H2) ∼ 1.4 × 109 M☉, which is ∼90% of its total molecular gas mass (for Xco = 0.45 Xl). This is rather puzzling, as the SF phase, with its expected high densities (⩾104 cm−3) and near gravitationally bound states (Kvir ∼ 1–5), can easily have CO lines thermalized and with substantial optical depths up to at least J = 3–2. Thus, if the SF phase indeed dominates the total molecular gas mass in this ULIRG, one would expect M(LTE)thin ≪ M(H2) rather than M(LTE)thin ∼ M(H2), as the latter implies small/moderate optical depths for the bulk of the molecular gas mass. Moreover, the physical states compatible with the global CO line ratios of IRAS 02483+4302, while highly degenerate, are hardly indicative of a dense SF gas phase dominating its total molecular gas mass. It is also telling that all one-phase LVG solutions fail to reproduce the CO J = 6–5 line luminosity of this LIRG, indicating the presence of another, potentially massive, gas component whose higher CO line excitation becomes prominent beyond J = 3–2. Using only its CO J = 3–2, 6–5, and the upper limits on the 13CO J = 1–0, 2–1 lines as constraints of the (h)-phase in a two-phase model still yields a significant range of solutions, though all have n ∼ (104–105) cm−3 with Tkin ⩾ 60 K, which is typical of a dense and warm SF-related phase. Most (but not all) have virial states (Kvir ∼ 1) with X(h)co ∼ (1.5–2.5) Xl. From the corresponding 〈r(h)65〉lvg ∼ 0.90 and 〈X(h)co〉lvg ∼ 2.2 Xl, we obtain L(h)co, 1–0 = 1.5 × 109 Ll and Mh − ex = 3.3 × 109 M☉. Higher CO J = 6–5 line luminosities will only make this mass larger (both via a higher L(h)co, 1–0 and a higher deduced 〈X(h)co〉lvg).
The "residual" global CO line ratios of the (l)-phase in this LIRG cannot distinguish between a cold, SF-quiescent gas at near-virial dynamic states (and Galactic X(l)co) and a warm and highly unbound phase expected in the highly turbulent environments of mergers (and with a low X(l)co because of the high Kvir). Thus, for ρ(l − h)co = 1.43 computed from our two-phase model, X(h)co = 2.2 Xl and X(l)co = (0.5–2.5) Xl, we obtain X(2 − ph)co = (1.2–2.4) Xl. Higher, near-Galactic, values remain possible if high-resolution CO imaging were to reveal the (l)-phase as an extended cold, SF-quiescent gas reservoir, something that global CO SLEDs cannot easily do.
B.5. IRAS 03359+1523
Two interacting galaxies ∼10'' (∼6.9 kpc) apart can be discerned in optical images, with only the eastern source being bright in radio wavelengths (Condon et al. 1990; Goldader et al. 1997) and containing most (≳75%) of the CO J = 3–2 emission (Leech et al. 2010; Paper I). This system has the lowest CO(3–2)/(1–0) ratio (=0.18 ± 0.05), indicating the lowest-excitation global CO line excitation in our sample, though we cannot exclude the possibility that significant CO J = 3–2 flux was "missed" by the two-point observations of this two-nuclei system.
The best LVG solution indicates SF-quiescent gas (Tkin = 15 K, n = 3 × 102 cm−3, and Kvir ∼ 2), yielding r32 ∼ 0.22 and R21 ∼ 18, which is somewhat higher than the observed value (=12 ± 3). The corresponding Xco ∼ 2.5 Xl gives Mtot ∼ 2.2 × 1010 M☉, while the LTE approximation for Tkin = 15 K gives M(LTE)thin = 1.8 × 109 M☉, which is ∼10× smaller than Mtot as expected given the large CO line optical depths (τ10 ≳ 14) of the corresponding LVG solution. The SF-related molecular gas mass MSF(H2) = 8.2 × 108 M☉ amounts to only ∼4% of M(H2), consistent with the low average CO line excitation of this system and ensembles of ordinary GMCs.
B.6. VII Zw 031
This galaxy has one of the highest CO J = 1–0 luminosities in our sample and, while early ground-based images were suggestive of an elliptical system (Sanders & Mirabel 1996), near-IR NICMOS images clearly show a spiral disk with very bright asymmetric arms configured tightly around its nucleus over scales of R ∼ (1–1.4) kpc (half-light radii), with numerous star clusters on its disk (Scoville et al. 2000). This is one of the few LIRGs where there is no evidence of what has triggered its starburst activity, namely, neither a nearby companion system (indicating an early merger stage) nor tidal tails (signs of an advanced or post-merger system). High-resolution interferometric imaging indicates a nearly face-on source with a rapidly rotating gaseous ring on scales of few hundred parsecs (DS98).
The low-J CO SLED with r21 = 0.72 ± 0.12 and r32 = 0.43 ± 0.19 indicates a rather low average line excitation, compatible even with SF-quiescent ISM (r21 ∼ 0.5–0.6, r32 ∼ 0.25–0.30). A radiative transfer model of only the CO J = 1–0, 2–1, 3–2, and 13CO J = 1–0, 2–1 lines yields Tkin = (30–65) K, n = 3 × 102 cm−3, and Kvir ∼ 2 (near-virial). The corresponding Xco ∼ 1.25 Xl factor yields M(H2) = 1.5 × 1010 M☉, while the minimum molecular gas mass needed to fuel an Eddington-limited star formation in this LIRG is MSF(H2) ∼ 2 × 109 M☉. On the other hand, a luminous CO J = 4–3 with r43 = 1.46 ± 0.45 (Paper I) is highly excited (and cannot be reproduced by such low densities), and the reason why one-phase LVG solutions, while reproducing the observed r32, 21 and R10, 21 line ratios within their measurement uncertainties, they yield r(lvg)43 ≲ 0.20–0.26, i.e., ∼6–7 times lower than observed. The CO J = 6–5 line luminosity with r65 = 0.22 ± 0.07 is also much higher than predicted by these models (r(lvg)65 = 0.01–0.02). Interestingly, the 13CO J = 1–0, 2–1 lines, with modest R10, 21 ∼ 10 and r21(13CO) ∼ 0.72 ratios, also indicate a low/average-excitation state.
Thus, only the CO J = 4–3 and J = 6–5 line luminosities signify the presence of another potentially massive warmer and denser gas phase, demonstrating once more the importance of high-J CO line measurements. Moreover, the r43 > r32 inequality sets the global CO SLED of VII Zw 031 apart from those reducible to a mixture of a dense and warm gas phase (with a CO SLED typical of Orion hot spots) and a cooler, diffuse one associated with non-SF gas and a low-excitation SLED. As in IRAS 00057+4021, this implies large amounts of hot and dense gas, maintained in a strongly unbound dynamic state as to keep the average optical depth of even high-J CO lines below unity (see also the discussion in Paper I). We note, however, that in VII Zw 031 the CO J = 3–2 line luminosity is highly uncertain because of the large system temperature, though even a CO J = 3–2 line three times stronger would maintain the aforementioned inequality and its implications for extraordinary ISM conditions in this galaxy.
We use the CO (4–3)/(3–2) ratio r43/32 to constrain the (h)-phase in a two-phase model while using the CO J = 6–5 luminosity only as a lower limit. We also assume the minimum r43/32 = (L'CO(4–3)−σ)/(L'CO(3–2)+σ) = 1.67 value consistent within the measurement uncertainties in order to place limits on the lowest possible average-excitation level for the (h)-phase. This will still be high, as even that minimum value is close to the theoretical maximum of (4/3)2, attained only for a hot gas phase with fully optically thin and thermalized CO lines. We find good solutions only for Tkin ⩾ 95 K, which keep improving up to the maximum Tkin = 150 K considered, while n = 3 × 104 cm−3 and Kvir = 22. No good solutions can be found for lower temperatures, as these have unphysical Kvir < 1 values. Over the good solution range, the corresponding 〈r(h)43〉 = 3.4 and r(h)65 ≳ 2. The corresponding 〈X(h)co〉 = 0.9 Xl, and from ∼ 3.45 × 109 Ll (we used the CO(4–3)σ value) we estimate Mh − ex = 3.1 × 109 M☉. As expected, with a 13CO(2–1)/(1–0) ratio of ∼0.73 (subthermal), an (h)-phase model cannot successfully include the 13CO lines, while their modest global R10, 21 ∼ 10 (Table 8, Paper I) are typical of disk GMCs rather than of those in starbursts (where R10, 21 ⩾ 15). This, along with the fact that there is very little CO J = 2–1 and 3–2 emission left after subtraction of the corresponding (h)-phase line emission, suggests a cold disk-like (l)-phase. Assuming an X(l)co = 4.5 Xl (i.e., Galactic), and for ρ(l − h)co = 2.34, we obtain X(2 − ph)co = 3.4 Xl and a total gas mass of M(2 − ph)tot = 3.9 × 1010 M☉. Thus, despite its prominence in CO J = 4–3 and 6–5 lines, the high-excitation component amounts to only ∼8% of the total molecular gas mass in VII Zw 031. Interestingly, the CO J = 2–1 interferometric map by DS98 resolves out half of the 30 m single-dish flux, indicating extended emission beyond the ∼2''(2 kpc) nuclear region where the bright CO J = 1–0 emission is distributed. Indeed, in the DS98 interferometric image, faint CO J = 1–0 emission can be seen out to a radius of ∼4'' (4 kpc). New sensitive CO and 13CO J = 1–0 and 2–1 interferometric observations with similar u–v coverage (thus enabling reliable line ratio maps) can be used to discern the existence of such a massive cold molecular gas disk in this intriguing object.
B.7. IRAS 05189−2524
This is a Sy2 galaxy, which appears to be a late stage merger with a very red compact nucleus and a tidal tail (Farrah et al. 2003; Veilleux et al. 2002). This (U)LIRG is the most compact and has the warmest mid-IR colors (f25 μm/f60 μm = 0.25) out of a nearly complete ULIRG sample selected for their "warm" mid-IR colors (f25 μm/f60 μm > 0.2). Such systems are thought to represent a critical stage in the so-called ULIRG→(optically luminous QSO) transition scenario (Sanders et al. 1988b, 1988c). The AGN in this ULIRG is X-ray luminous with Lx(2–10 keV) ∼ 1.3 × 1043 erg s−1 (Dadina 2007). NICMOS near-IR imaging reveals an unresolved nucleus in all three near-IR bands, with a half-light radii of ∼(100–140) pc, while CO J = 1–0 observations show large amounts of molecular gas (Sanders et al. 1991). No interferometric CO images are yet available for this southern ULIRG.
Unlike all other mergers/starbursts, IRAS 05189−2524 has a small R21 = 6 ± 2 ratio, typical of the self-gravitating (or nearly so) GMCs in spiral disks. As a result, all LVG solutions within the expected temperature range (i.e., Tkin/Td ≳ 1) have Kvir ∼ 1–2, indicating virialized gas motions quite unlike the much higher Kvir found in merger/starbursts. The observed r21 = 0.67 ± 0.15 together with the modest R21 are actually perfectly compatible with an SF-quiescent phase (where r21 ∼ 0.6), although an SF-active one (r21 ∼ 0.8–1) is certainly not excluded within the measurement uncertainties. The best LVG solutions converge toward conditions typical of Galactic GMCs but at elevated temperatures with Tk = (30–55) K, n(H2) = 3 × 102 cm−3, and Kvir ∼ 1 and yielding r21 = 0.86–0.91, r32 = 0.50–0.67, r43 = 0.30–0.40, and R21 = 9. Using values within the rJ + 1, J ± σ range as constraints does not change the basic picture of low-density, somewhat warm gas in a virialized or nearly so dynamic state. For the best solution, it is Xco ∼ 3.5 Xl, which yields Mtot(H2) = 1.37 × 1010 M☉.
Denser and warmer gas must of course be present, associated with the vigorous star formation in IRAS 05189−2524 and the minimum mass needed to fuel it, MSF(H2) ∼ 3.6 × 109 M☉. The CO J = 6–5 line also indicates gas with significantly higher excitation than that indicated by the lower-J CO lines since, for the optimal LVG solutions for the low-J CO SLED segment, r65 (lvg) ∼ 0.01–0.05, which is much lower than the observed r65 = 0.37 ± 0.14. Using a two-phase model with an Orion-derived SLED and an Eddington-limited mass normalization for the (h)-phase (see Section 2.4) yields (l)-phase ratios of r(l)21 = 0.45, r(l)32 = 0.28, and R(l)21 = 3.5, with the best LVG solution being Tkin = 15 K, n ∼ 300 cm−3, and Kvir ∼ 2.2, i.e., typical of SF-quiescent GMCs found in the disk of the Galaxy. The corresponding X(l)co = 2.5 Xl, which for a computed = 2.3 × 109 Ll, yields a total mass of cold SF-quiescent molecular gas of ∼6 × 109 M☉. For the estimated ρ(l − h)co = 1.43 and X(h)co = 2.2 Xl (see Section 2.4), we find X(2 − ph)co ∼ 2.4 Xl and an M(2 − ph)tot ∼ 9.4 × 109 M☉, with ∼65% of this gas mass in a cold SF-quiescent phase.
This merger ULIRG/AGN with its large molecular gas reservoir, nearly-Galactic Xco (∼3–6 times higher than the so-called ULIRG values of ∼(0.6–0.8) Xl) and most of its molecular gas mass in a cold reservoir, stands as the clearest counterexample to standard views regarding this class of galaxies in the local universe. Moreover, unlike I Zw 1 (another prominent "warm" LIRG considered midway in a supposed ULIRG→(optical QSO) transition), whose known spiral disk can be the repository of its large SF-quiescent molecular gas reservoir, the very compact size of IRAS 05189−2524 in cm continuum, near-IR, and optical wavelengths (Condon et al. 1990; Scoville et al. 2000; Surace et al. 1998) seems to preclude such a configuration. This compactness and the "warm" CO(6–5)/(3–2) ratio of ∼0.63 (i.e., dominated by the SF phase) in an ULIRG/AGN whose molecular gas reservoir is dominated by a cold SF-quiescent component offer a cautionary tale regarding similar ULIRG/AGN objects at high redshifts, where such characteristics were often used (and still are) to argue for the presence of only a dense and warm SF gas reservoir (Tacconi et al. 2006). Future ALMA observations of this southern ULIRG and similar objects would thus be very interesting for revealing the distributions of their large molecular gas reservoirs and especially of their cold phases.
B.8. IRAS 08030+5243
This LIRG is one of the very few (=3) appearing as a single undistorted nucleus in a large near-IR (2.2μm) and optical imaging survey of 56 ULIRGs selected from the IRAS 2 Jy sample (Murphy et al. 1996). Its CO line ratios reveal a low/average-excitation state, but lack of 13CO observations permits a very wide range of conditions to reproduce its low global r32 and r21 ratios. In turn, these conditions yield a large range of Xco values, from Xco ∼ 4.6 Xl (for Tkin = 15 K, n ∼ 300 cm−3, and Kvir ∼ 1) to Xco ∼ (1.5–1.7) Xl (for Tkin = (55–90) K, n ∼ 100 cm−3, and Kvir ∼ 1) and also down to Xco ∼ 0.61 Xl (for Tkin = [20 K, (40–50) K], n ∼ [103, 300 cm−3], and Kvir ∼ [13, 7], respectively). For a set of very warm (Tkin = (95–130) K) and strongly unbound (Kvir = 22) diffuse gas (n = 3 × 102 cm−3) solutions, the Xco factor can become as low as ∼0.3 Xl. It is worth remembering that such gas may indeed exists in most ULIRGs as an "envelope" phase of a much denser phase, a result of disrupted GMCs during a strong merger. Its high brightness in low-J CO lines (a result of its high Tkin and Kvir values) is responsible for the low Xco deduced for such systems (which nevertheless can have most of their molecular gas mass in a much denser phase with high Xco; see the discussion in Section 3.2).
Demonstrating the importance of 13CO line measurements, we note that the CO/13CO J = 1–0 ratio changes dramatically among the aforementioned LVG solution groups, from R10 ∼ 5 for cold virialized gas to R10 ∼ 30 for the warm highly non-virial ISM states. Nevertheless, lack of such measurements makes it impossible to constrain the LVG solution groups and thus Xco in this ULIRG. Values as low as Xco = 0.3 Xl are likely excluded, however, as they yield Mtot ∼ 3 × 109 M☉, comparable to MSF(H2) = 2.4 × 109 M☉. In such a case, the global CO SLED would be that of dense warm SF gas (i.e., highly excited) rather than of low excitation.
B.9. IRAS 08572+3915
This is another prominent ULIRG from the "warm" ULIRG sample (Sanders et al. 1988b), consisting of a close pair of interacting spirals with their nuclei separated by 5'' (∼5.6 kpc) and clearly discernible in cm, near-IR, and optical images (Condon et al. 1990; Scoville et al. 2000; Surace et al. 1998). Its NW nucleus is unresolved in near-IR and is the only one detected in CO J = 1–0 interferometer maps, where it appears unresolved with θco ⩽ 21(∼2.3 kpc) (Evans et al. 2002). Mid-IR (12–25 μm) imaging showed all the mid-IR emission also emanating from the NW nucleus and a region ≲330 pc in diameter (Soifer et al. 2000; and for the adopted cosmology).
The discovery of very luminous CO J = 6–5 emission toward the NW nucleus (Paper I, Figure 2), corresponds to the second highest r65 ratio in our entire sample (∼1), indicating extreme ISM excitation conditions with average gas densities of ∼(105–106) cm−3 and LVG fits that keep improving past Tkin = 150 K. The 3σ upper limit on r32 ≲ 2.54 is certainly compatible with such conditions, albeit without imposing any useful additional constraints (high Tsys because of νsky(3–2) near the 325 GHz atmospheric absorption feature prevented sensitive CO J = 3–2 observations of this system). The high r65 ratio measured for the NW nucleus of this ULIRG is actually perfectly compatible with a pure Orion "hot spot" CO SLED (see Section 2.4), further emphasizing the truly extraordinary levels of ISM excitation. The corresponding molecular gas mass is at least MSF(H2) ∼ 3.9 × 109 M☉ (assuming an Eddington-limited SF). A Galactic Xco ∼ (3–6) M☉ (K km s−1 pc2)−1 factor is deduced from the LVG solutions with Tkin ⩾ 65 K, which corresponds to M(H2) ∼ (4.8–9.6) × 109 M☉. Thus, MSF/Mtot ∼ 0.41–0.81, which is among the highest such fractions for the individually studied LIRGs (see Table 1).
B.10. Arp 55
This is another clear merger having double nuclei as IRAS 08572+3915, but more widely separated (∼12'', 9.3 kpc) as seen in radio continuum maps (Condon et al. 1990) as well as optical and CO J = 1–0 interferometric maps (Sanders et al. 1988a). A tidal tail emerging from the eastern nucleus (also the most gas-rich one) is clearly visible in Hα emission (Hattori et al. 2004). The two nuclei have been separately detected in CO J = 3–2 (Leech et al. 2010; Paper I), while the NE nucleus may have been tentatively detected also in CO J = 6–5 (Paper I), with an implied high CO(6–5)/(3–2) brightness temperature ratio of r65/32 = 0.75 indicating a highly excited molecular ISM phase. On the other hand, the global HCN/CO J = 1–0 brightness temperature ratio is ∼0.03 (Gao & Solomon 2004), which is typical of the molecular gas found in isolated spiral disks and quite unlike the much higher such ratios in merger/starbursts.
Using the global r21 and r32 and the lower limit on the CO/13CO J = 2–1 ratio (see Paper I) yields a large range of possible average ISM states, all with n ∼ (1–3) × 103 cm−3 and most with surprisingly large Kvir (∼70–126). Restricting Kvir to values ⩽40 yields a much narrower range of solutions with Tkin = (45–70) K and corresponding Xco ∼ (0.25–0.8) Xl. This still considerable Xco range yields Mtot ∼ (2.9–9.2) × 109 M☉, while MSF ∼ 0.9 × 109 M☉. The latter is ∼1/10–1/3 of the total molecular gas mass, leaving ample room for an SF-quiescent molecular gas reservoir. In Arp 55, however, SF-quiescent is not the cool and mostly gravitationally bound gas found in the GMCs of isolated disks. Indeed, the large temperatures and Kvir values needed to fit its highly excited CO lines and R21 ≳ 13 suggest warm and strongly unbound gas that, unlike that phase in most other ULIRGs, contains most of the molecular gas mass. The low HCN/CO J = 1–0 ratio then may not be due to the prevalence of Galactic-type SF-quiescent GMCs rather than to a disrupted ISM state that has, momentarily, a low (dense)/(total) molecular gas mass fraction. Short periods during which the dense gas reservoir of an LIRG can be strongly disrupted by SF feedback while its IR luminosity remains intact are expected in strongly evolving mergers (Loenen 2009). In such a scenario, Arp 55 would be one of the few mergers "caught" in a very short act (see also Arp 193). Interestingly, its HCN(3–2)/(1–0) ratio is <0.3 (Juneau et al. 2009), which is much lower than in some classical (U)LIRGs such as Arp 220 and NGC 6240 (Greve et al. 2009). More sensitive 13CO and higher-J HCN observations of Arp 55 are necessary to confirm such a tantalizing picture of global ISM dynamics.
B.11. IRAS 09320+6134 (UGC 05101)
Near-IR NICMOS observations show a single extremely red and unresolved nucleus with θs ⩽ 022 (∼170 pc), surrounded by a strongly perturbed spiral structure (Scoville et al. 2000) with a tidal tail and a large ring seen in the optical (Sanders et al. 1988c). The half-light source radius at 2μm is ∼450 pc, while an interferometric CO J = 3–2 image reveals a gas distribution of ∼2''(1.5 kpc) in size (Wilson et al. 2008). Its disturbed morphology is interpreted as the result of an interaction with another gas-rich spiral (Sanders et al. 1988c) and even with a gas-poor dwarf galaxy 17'' to the southeast (Majewski et al. 1993). This ULIRG contains an AGN luminous in hard X-rays (Imanishi et al. 2003) and is deeply dust-enshrouded along most lines of sight, with N(H)>1024 cm−2 (Imanishi et al. 2001).
Its CO SLED betrays the presence of a high-excitation gas component already from the low-J lines with r21 = 1.23 and r32 = 0.93 (Paper I). These ratios along with the high CO/13CO J = 2–1 ratio of R21 = 18 can be well fitted by two ranges of LVG solutions, namely Tkin = (35–65) K, n = 3 × 103 cm−3, Kvir = 22, and Tkin = (100–130) K, n = 103 cm−3, Kvir = 4. The corresponding average Xco factors are Xco ∼ 0.4 Xl and Xco ∼ 0.6 Xl, respectively. We note that a small range of solutions with Tkin = (25–30) K, n = 104 cm−3, and Kvir = 40 also exists, but these are unlikely to be good representations of the average ISM state in this ULIRG since Tkin/Tdust < 1 (considered unlikely, see Paper I). Moreover, their very large Kvir values make it even less likely that these modest gas temperatures can correspond to such highly unbound dynamic states where the molecular gas can be significantly heated by the dissipated supersonic turbulence (see Paper I). Nevertheless, adopting this narrow cooler gas solution range would not make much difference when it comes to the corresponding Xco factor, which is ∼0.5 Xl. The corresponding total molecular gas mass then is Mtot ∼ (1.9–2.9) × 109 M☉, while MSF ∼ 1.6 × 109 M☉.
The large fraction of SF gas mass in UGC 05101 (∼(55–84)%) is further corroborated by the high HCN/CO J = 1–0 brightness temperature ratio of ∼0.2 (Gao & Solomon 2004; for typical spiral disks this ratio is ∼0.02–0.03) insofar as HCN J = 1–0 is a linear proxy for dense gas mass and most of that gas is involved in star formation. This dense gas reservoir is likely confined to the small (near-IR)-bright nucleus fueling a compact starburst. Such compact ∼100 pc sized gas disk/starburst configurations have been revealed for Arp 220 (Sakamoto et al. 2008; Matsushita et al. 2004), where extremely high extinctions (N(H) ⩾ 1025 cm−3) correspond to significant dust optical depths even at submm wavelengths (Sakamoto et al. 2008; Papadopoulos et al. 2010a). These can be responsible for Compton-thick AGNs and the suppression of high-J CO lines in such systems. This may be the case also for UGC 05101, as indicated by its low CO(6–5)/(1–0) yet high HCN/CO J = 1–0 ratio (see Figure 11 of Papadopoulos et al. 2010b).
Despite the good one-phase fit obtained for the global CO SLED (and R21) of this ULIRG, the observed CO line emission may still be dominated by a phase that does not contain the bulk of its molecular gas mass. Indeed, neither the modest densities nor the potentially highly unbound average dynamic states implied by the one-phase LVG fits would be typical of the HCN-bright star-forming phase. As discussed in Section 3.2, the Xco obtained from the global low-J CO SLED may thus be suitable only for a gas phase that does not contain much of the molecular gas mass. Using the observed r(obs)CO/HCN = 5 in the context of a two-phase model along with r(h)CO/HCN = 2.34 (see Section 3.2) yields ρ(l − h)co = 1.136 (Equation (14)). In the absence of multi-J HCN observations that could constrain XHCN, we set XHCN = 10 Xl, the smallest value produced by such studies (e.g., Papadopoulos 2007; Graciá-Carpio et al. 2008). Thus, X(h)co = (r(h)CO/HCN)−1XHCN ∼ 4.3 Xl, and for X(l)co = 0.5 Xl, it would be X(2 − ph)co = 2.3 Xl, which is ∼(4–6)× higher than that deduced from one-phase LVG models of the low-J CO SLED. Multi-J observations of HCN or other heavy rotor molecules (e.g., CS, HCO+) can confirm such potentially high masses of molecular gas at high densities.
B.12. NGC 3310
The young and intense star formation activity of this UV-bright galaxy is on par with M 82 and is the likely result of a recent merger with a smaller galaxy (Balick & Heckman 1981; Conselice et al. 2000; Elmegreen et al. 2002). Its gas and dust are warmed and disrupted by the presence of the starburst in its central 1–2 kpc. Tidal H i tails, unusually large H i velocity dispersions (∼40 km s−1) for a spiral galaxy (Kregel & Sancisi 2001), and large velocity offsets (up to 150 km s−1) between molecular cloud and adjacent H ii regions (Kikumoto et al. 1993) provide further evidence for a dynamically disturbed gas typical of mergers.
This galaxy has been extensively studied by Zhu et al. (2009) as a template for a highly excited ISM, indicated by r21, 32 > 1 ratios and warm IR "colors" (S100 μm/S60 μm = 1.28). Despite its low IR luminosity (L(*)IR ∼ 2.7 × 1010 L☉; Paper I), its global r21 = 1.47 and r32 = 1.21 ratios surpass those of Orion cloud SF "hot spots" and are among the highest observed in our sample. Its proximity allowed spatially resolved studies of its molecular gas with single-dish telescopes obtaining beam-matched observations of the CO(3–2)/(1–0) ratio (see Zhu et al. 2009 for details). These high global CO line ratios of NGC 3310 can be fitted well by a single phase provided Tkin ⩾ 35 K (∼Tdust), but remain highly degenerate over the [Tkin, n, Kvir] domain up to the highest temperature searched (150 K). Interestingly, for Tkin = (35–50) K a class of dense (n ∼ (1–3) × 104 cm−3) and strongly unbound (Kvir ∼ 20–40) states is possible, though only higher-J and/or 13CO line observations could reduce the wide degeneracy of the LVG solutions and designate such phases as those most likely representing the actual ISM conditions. Other solution ranges are Tkin = (70–90) K, n = 3 × 103 cm−3, and Kvir = 22, while near-virial solutions can be found at high temperatures Tkin = 95 K, (140–145) K, n = (1–3) × 104 cm−3, and Kvir = 4, 2.2. The large degeneracy of possible average ISM conditions translates to a significant one for the Xco factors, ranging from ∼0.4 Xl (for the highly unbound states with Kvir = 22–40) to ∼(1.2–2.2)Xl (for the near-virial states with Kvir = 4, 2.2).
For Xco = (0.4–2.2) Xl, the corresponding Mtot = (0.3–1.5) × 108 M☉, while MSF = 1.1 × 108 cm−3. The latter seems to rule out the lowest Xco values, while for even the highest ones it is MSF/Mtot ∼ 0.73, i.e., most of the molecular gas in NGC 3310 is directly involved in star formation (for an Eddington-limited SF process), leaving little room for a massive low-excitation molecular gas reservoir. Interestingly, the LVG solutions corresponding to the highest Xco values are those indicative of an SF phase with n ∼ 3 × 104 cm−3, Kvir = 2.2, and large Tkin = (140–145) K. We caution, though, that all this pertains only to the inner ∼40'' of this galaxy where the starburst takes place. A massive molecular gas reservoir with low densities, highly unbound gas motions, and low CO line excitation can still exist beyond it this is a situation actually encountered in M 82 in the form large-scale molecular gas outflows (Weiss et al. 2005).
B.13. IRAS 10565+2448
This is an ULIRG with H ii region-type line ratios (Armus et al. 1989) implying an optical spectrum dominated by young stars rather than by an AGN. Nevertheless, a weak contribution from an AGN seems necessary in order to fit its IR/submm dust continuum SED (Farrah et al. 2003). Early r-band (6550 Å) imaging has shown this object to be a possible triple merger galaxy system (Murphy et al. 1996), while near-IR imaging with the Hubble Space Telescope (HST) NICMOS camera shows a luminous primary galaxy interacting with a much fainter one ∼8'' (∼6.7 kpc) to the southeast (Scoville et al. 2000). The primary galaxy is compact with a half-light source diameter at 2 μm of ∼650 pc, the only source where luminous CO J = 1–0 line emission is detected (DS98) with a diameter of 15 (1.25 kpc). Moreover, this ULIRG along with VII Zw 031 and Arp 193 are the only galaxies where kinematic models of the CO emission indicate a rotating ring rather than a filled disk gas distribution (DS98).
Its low-J CO lines (J = 1–0, 2–1, 3–2) indicate the well-excited low-J CO SLED (r21 = 1.06, r32 = 0.80) expected for a starburst, while the large R10 = 15 and R21 ≳ 18 are typical for merger systems. Two ranges of one-phase LVG solutions that can be found for Tkin/Tdust ⩾ 1 (with Tdust = 40 K) are [Tkin, n, Kvir] = [(40–50) K, 3 × 103 cm−3, 7] and [Tkin, n, Kvir] = [(80–140)K, 103 cm−3, 4], with the best ones obtained for Tkin = 45 K and 115 K, respectively. Both also reproduce the CO J = 6–5 line luminosity with r(lvg)65 ∼ 0.15 (observed value: 0.18 ± 0.055).
The resulting Xco factors are Xco = 0.75 Xl (for Tkin = 45 K) and 0.60 Xl (for Tkin = 115 K), yielding a total molecular gas mass of Mtot = (3.8–4.80) × 109 M☉. The minimum such mass needed for Eddington-limited SF is MSF = 2.6 × 109 M☉, which is ∼54%–68% of Mtot. As in UGC 05101, the aforementioned large fraction of molecular gas mass directly involved in star formation in IRAS 10565+2448 is corroborated by a high HCN/CO J = 1–0 brightness temperature ratio of rHCN/CO ∼ 0.17 (Gao & Solomon 2004). However, all the one-phase LVG solutions obtained are hardly representative of a dominant dense and self-gravitating gas phase; they are instead more typical of the "cloud-envelope" warm and diffuse gas often found in the ISM of mergers. Moreover, all one-phase LVG fits fail to reproduce the R21 ≳ 18 lower limit, with R(lvg)21 = 12 being the highest value obtained (for the Tkin = 115 K solution). A two-phase model with an assumed (h)-phase alleviates this and yields an (l)-phase with Tkin = 65 K, n = 103 cm−3, and Kvir = 13 that has R21 ∼ 20 and X(l)co = 0.4 Xl. The computed X(2 − ph)co ∼ 0.75 Xl is within the range of the one-phase values, a result of the low X(l)co, the large luminosity contribution of the (l)-phase (ρ(l − h)co ∼ 4.5), and the modest X(h)co = 2.2 Xl of the (h)-phase (see Section 2.4).
Setting constraints on the (h)-phase and X(h)co by using the observed CO J = 3–2, 6–5, and 13CO J = 1–0 lines (assumed to be emanating mostly from that phase) does not change the aforementioned picture, at least when it comes to the values of X(2 − ph)co. Indeed while the corresponding LVG models do recover dense gas solutions (n ∼ 104 cm−3), substantial Kvir values (∼13) keep the corresponding X(h)co well below Galactic. In this model, the (l)-phase contribution and thus the influence of X(l)co on the X(2 − ph)co are negligible. We must stress, however, that other high-J CO line observations and, crucially, multi-J HCN observations are necessary to confirm this picture. If such luminous lines were to be discovered for this ULIRG, they could substantially raise X(h)co and thus X(2 − ph)co, as discussed in Section 3.2.
B.14. Arp 299
This is a spectacular merger of two galaxies, IC 694 and NGC 3690, with luminous CO J = 1–0 emission detected in the nuclei of both (which are 22''—4.4 kpc apart), and the interface between them (Sargent et al. 1987; Sargent & Scoville 1991). A long H i tidal tail is also seen extending out to ∼180 kpc (Hibbard & Yun 1999). Along with the Antennae galaxy, this (U)LIRG has been considered an early template for mergers, documenting their ability to rapidly funnel the gas in the disks of the progenitors into compact regions (Sargent & Scoville 1991). Indeed, the two nuclei in Arp 299 contain ∼80% of its molecular gas reservoir, fueling intense star formation, while the nucleus of IC 694 contains also an AGN (Sargent & Scoville 1991 and references therein) and is the primary source of the bolometric luminosity of this spectacular system (Charmandaris et al. 2002). The most detailed molecular line study of this template merger system is the interferometric study of CO, 13CO, and HCN J = 1–0 line emission by Aalto et al. (1997), which found most of the HCN-bright emission to be located in compact regions (≲310 pc) in the two nuclei of the merging galaxies, and unusually large CO/13CO line ratio variations (from ∼60 in the IC 694 nucleus to ∼5–10 in its disk). Actually, the state of the gas in the IC 694 nucleus with the largest HCN/CO and CO/13CO ratios exemplifies the effects of highly turbulent environments found in mergers, with much of the molecular gas mass "resettled" at high densities (boosting the HCN/CO ratio), while the violent disruption of GMCs creates a diffuse warm and highly unbound "envelope" phase dominating the low-J CO lines (boosting the CO/13CO ratios).
While we have no CO J = 6–5 measurements for this galaxy (it is too extended for the narrow James Clerk Maxwell telescope (JCMT) beam at 690 GHz), our CO J = 3–2 and J = 4–3 measurements indicate high CO line excitation. A one-phase LVG model of the global CO (3–2)/(1–0), (4–3)/(1–0), and CO/13CO J = 1–0 line ratios gives the best fit for Tkin = 30 K, n = 104 cm−3, and Kvir = 40, which indicates strongly unbound gas motions. Other average gas states are also possible (e.g., Tkin = 40 K, n = 3 × 103 cm−3, and Kvir = 22), but all have Kvir ≳ 22. The corresponding Xco factor over the LVG solution range is Xco = (0.35–0.42) Xl, with the largest value corresponding to the dense gas solution. These yield Mtot ∼ (1–1.2) × 109 M☉, while MSF ∼ 1.9 × 109 M☉, which clearly favors the higher Xco values and indicates that ∼100% of the molecular gas mass is associated with SF sites. Larger values of Xco (and Mtot) remain possible, however, if most of the molecular gas mass in Arp 299 is contained in the HCN-bright phase with large XHCN factors. Multi-J CO, 13CO and HCN, H13CN line imaging would be ideal in determining this as a function of position within a template merger whose ISM conditions seem to encompass the full range possible, from quiescent disks to compact starburst nuclei.
B.15. IRAS 12112+0305
This ULIRG consists of a strongly interacting pair of galaxies whose nuclei are ∼29 (∼4 kpc) apart (Carico et al. 1990; Scoville et al. 2000), while tidal tails and "plumes" are visible both in optical (Surace et al. 2000) and near-IR (Scoville et al. 2000) wavelengths. CO J = 1–0 interferometry finds the bulk (∼75%) of the emission emanating from the NE nucleus of this system (Evans et al. 2002), which is unresolved with a size of ≲2'' (≲2.7 kpc) and is also the only one tentatively detected in CO J = 6–5 (see Paper I). Thus, our one-phase LVG radiative transfer modeling pertains only to the NE nucleus of this strongly interacting system. The observed r32 = 1.58 ratio is the second highest in our sample (the highest one being found in another double-nuclei system: IRAS 22491−1808), and it indicates the presence of high-excitation molecular gas. This surpasses the corresponding ratio of the SF "hot spots" in the Orion molecular cloud, while the r32 > r21 inequality is another indication of a qualitatively different CO SLED, irreducible to a mixture of SF and SF-quiescent gas (see Paper I).
All one-phase LVG solutions for IRAS 12112+0305 indicate average gas states with n ∼ (3 × 104–106) cm−3. Nevertheless, lack of 13CO line observations leaves serious degeneracies with, e.g., [Tkin(K), n(cm−3), Kvir] = [60, 105, 13], [125, 104, 1] being nearly equivalent. The corresponding Xco ∼ (1.5–5.3) Xl, with Galactic values of ∼(4–5) Xl obtained for the warmest (=(120–150) K) and densest (=(105–106) cm−3) states. The presence of such high densities is corroborated by a high HCN/CO J = 1–0 ratio of rHCN/CO = 0.16 (HCN from Graciá-Carpio et al. 2008; CO from Paper I), though this is without offering any constraints on the temperatures of the dense gas or its all-important kinematic state. However, even the warmest and densest one-phase solutions remain rather poor fits of the "hot" r32 ∼ 1.6 and comparatively "cooler" r21 ∼ 0.9 ratio. Indicatively, such a solution (n = 106 cm−3) with Tkin = 150 K and Kvir = 4 yields r32 ∼ r21 ∼ 1 as the high density and modest Kvir act to keep the CO lines well thermalized but also optically thick up to high-J levels.
A two-phase model of the global CO line ratios in this intriguing ULIRG cannot use an Orion-derived (h)-phase CO SLED as the observed r32 > r(h)32(Orion). Using only the CO (3–2)/(2–1) ratio r32/21 = 1.76 ± 0.48 to constrain the high-excitation (h)-phase yields n = 3 × 104 cm−3, Kvir = 22, and Tkin ≳ 140 K (for Tkin = 140 K, r(lvg)32/21 = 1.28 ∼ r32/21 − σ), with a fit that keeps improving past Tkin = 200 K (where r32/21 = 1.41). This is expected since r32/21 > 1 indicates a thermalized J = 3–2 line (and thus n ≳ ncrit, 3–2 ∼ 104 cm−3) with low/modest optical depths (ensured in part by the large Kvir) and warm enough gas to populate the J = 3 level. The molecular gas mass of this phase can be estimated from ∼ 4 × 109 Ll (where r(h)32 ∼ 4) and the computed X(h)co ∼ 1 Xl, thus, Mh − ex(H2) ∼ 4 × 109 M☉, similar to that needed to fuel an Eddington-limited SF in this ULIRG of MSF(H2) ∼ 5 × 109 M☉. For the (l)-phase
∼ 6.1 × 109 Ll luminosity, there are no constraints on its corresponding X(l)co factor. Setting X(l)co = (0.5–5) Xl yields X(2 − ph)co = (0.7–3.4) Xl and a corresponding Mtot ∼ (7.1–34.3) × 109 M☉. Multi-J HCN and H13CN as well as 13CO line observations are critical for reducing the degeneracies of the radiative transfer models for the dense and the low-excitation gas and their Xco factors.
B.16. Mrk 231
This is an archetypal ULIRG/QSO and the most IR-luminous galaxy in the Revised IRAS Bright Galaxy Survey (RBGS; Sanders et al. 2003). It has a compact nucleus surrounded by irregular incomplete rings of star formation along with a small tidal arm that contains numerous blue star-forming "knots" (Farrah et al. 2003 and references therein). It has large amounts of molecular gas in a compact (085, 700 pc) nearly face-on disk (Bryant & Scoville 1996; DS98), which allows a nearly unobscured view toward an optically luminous AGN, which also classifies this object also as a Seyfert 1 galaxy. Mrk 231 was also the first ULIRG for which high-J CO lines (J = 4–3, 6–5) were detected (Papadopoulos et al. 2007), while recent SPIRE/FTS observations with the HSO revealed luminous high-J CO lines up to J = 13–12 emanating from AGN-induced X-ray Dissociated Regions (van der Werf et al. 2010). Its well-excited high-J CO lines, the largest HCN/CO J = 1–0 ratio (rHCN/CO ∼ 0.29) observed among ULIRGs (Papadopoulos et al. 2007), and a very high CO/13CO J = 2–1 ratio exemplify the extraordinary average molecular gas states possible in such systems (similar to the IC 694 core in the Arp 299 merger system). Finally, the availability of HCN J = 4–3, 1–0 measurements along with the high-J CO lines have allowed a detailed study of its dense gas phase (Papadopoulos et al. 2007) and make Mrk 231 a good case study for the effects of the large M(n > 104 cm−3)/Mtot fractions on the global Xco of ULIRGs.
A one-phase LVG fit predictably fails to converge on any average state, as it cannot accommodate both the high densities needed to excite the CO J = 3–2, 4–3, and 6–5 lines and maintain the very low optical depths needed for reproducing the very large R10 = 47 ratio. Indicatively, the best one-phase LVG solutions are as follows: Tkin = (115–150) K, n ∼ (300–103) cm−3, and Kvir = 7–40 yield r(lvg)21 ∼ 0.83–1.1 (obs = 0.89 ± 0.12), r(lvg)32 ∼ 0.5–0.7 (obs = 0.72 ± 0.13), r(lvg)43 ∼ 0.22–0.3 (obs = 0.8 ± 0.2), and r(lvg)65 ∼ 0.03 (obs = 0.42 ± 0.12, but not used for the LVG fit), while R(lvg)21 = 25–32 (obs = 47 ± 16). Thus, the average ISM state deduced by a one-phase LVG model can be responsible for up to J = 3–2 (and the 13CO J = 2–1) line emission but severely underpredicts the CO J = 4–3, and 6–5 line luminosities. The corresponding Xco ∼ (0.25–0.45) Xl is low, but it is within what was thought to be the ULIRG-appropriate range (for Mrk 231, DS98 give Xco ∼ (0.7–0.8) Xl). These yield Mtot ∼ (1.8–3.2) × 109 M☉, but a minimum MSF = 5.5 × 109 M☉ clearly favors the higher Mtot (and Xco) among those computed with the one-phase model.
For a two-phase model, we use the results by Papadopoulos et al. (2007) of warm (∼(85–140) K), diffuse gas (∼300 cm−3) that is highly unbound (Kvir ≫ 1), and dense gas (∼(1–3) × 104 cm−3, Tkin = (40–70) K) responsible for the bright CO J = 6–5, HCN J = 1–0, 4–3 lines. The latter phase is virialized with XHCN ∼ (10–25) Xl (for our numerical factor in Equation (3) rather than the one in Papadopoulos et al. 2007) and r(h)CO/HCN ∼ 2–2.54 (high value corresponding to the lower XHCN values). Thus, X(h)co = (1/r(h)CO/HCN)XHCN ∼ (3.94–12.5) Xl, while for the diffuse and HCN-dark phase X(l)co ∼ (0.4–1) Xl. For an r(obs)CO/HCN = 3.45, we then find ρ(l − h)co ∼ 0.36–0.73 (yielding X(h)co = (3.94–12.5) Xl) and an X(2 − ph)co ∼ (3–7) Xl (for the smallest X(l)co). Thus, a Galactic Xco factor applies in Mrk 231, a result of a large mass of dense gas with high X(h)co values, themselves a result of the high gas densities and their virial dynamical states.
B.17. Arp 193
This galaxy is a template of a young merger-induced starburst with an age of few × 107 yr and near-IR imaging showing a highly dust-enshrouded nucleus and tidal tails (Smith et al. 1995). A disturbed highly inclined disk is also seen in NICMOS near-IR imaging with reddening increasing toward the NW within the disk (Scoville et al. 2000), and a similar extinction distribution implied by a CO J = 1–0 interferometer map (Bryant & Scoville 1999). Extended radio continuum emission (∼3'' × 37) is seen at cm wavelengths with two nuclei ∼1'' apart and a spectral index of α ∼ 0.6 (Sν∝ν−α), consistent with SF-related non-thermal synchrotron emission (Clements & Alexander 2004). The latter study also finds significant decoupling of the H i and CO-bright H2 gas motions and distributions. The young age of this disk–disk merger/starburst is corroborated by several extra-planar highly luminous star clusters (Scoville et al. 2000) and many bright H ii regions (Clements & Alexander 2004).
High S/N CO J = 1–0 and 2–1 interferometric imaging confirmed a highly inclined 28 × 0
8 (∼1.3 kpc × 0.37 kpc) gas disk whose CO emission is best fit by a molecular ring (DS98). A compact southeast CO source coinciding with the secondary cm continuum emission peak in Arp 193 has the properties of a giant molecular core and is much warmer and denser than the rest of the disk. Along with a region in Mrk 273 and the western nucleus of Arp 220 (DS98) it is one of the most extreme SF regions in the local universe, forming stars very near or at the Eddington limit set by the radiation pressure from young massive stars on the dust of the dense molecular gas (i.e., LIR/Mdense(SF-region) ∼ (250–500) L☉/M☉). One-phase LVG solutions to its CO line ratios yield low-density solutions with n ∼ (1–3) × 103 cm−3 but large temperature degeneracies with Tkin = 40 K, (60–75) K, (80–115) K, and corresponding Kvir = 22, 4, and 13 values. Warmer solutions with Tkin = (120–150) K and even larger Kvir(=40) values also exist. Considering only the gas states with Kvir ≲ 20 yields Xco ∼ (0.35–0.75) Xl and Mtot ∼ (1.6–3.5) × 109 M☉, with the highest Xco (and Mtot) values corresponding to the states with the smallest Kvir. The minimum molecular gas mass for an Eddington-limited star formation is MSF ∼ 0.9 × 109 M☉.
A young starburst and the strong SF feedback expected in such extreme SF events could be responsible for Arp 193 being the only LIRG where, despite a large SF-powered IR luminosity (∼2.2 × 1011 L☉) and HCN/CO J = 1–0 ratio (∼0.044, with HCN J = 1–0 from Graciá-Carpio et al. 2008, and CO J = 1–0 from Paper I), the average state of its molecular ISM is compatible with a complete absence of a dense molecular gas reservoir (Papadopoulos 2007). This is indicated by its low HCN (4–3)/(1–0) and (3–2)/(1–0) line ratios of r43 ≲ 0.12 (3σ) and r32 = 0.22 ± 0.03 (Papadopoulos 2007; Graciá-Carpio et al. 2008). Using these as constraints yields a narrow temperature range of dense gas solutions with Tkin = (50–60) K, n = 3 × 104 cm−3, and Kvir = 14 (which is rather large for a dense gas phase). The widest range of solutions is at Tkin = (65–95) K, n = 3 × 103 cm−3, and Kvir ∼ 1. Unlike the former set of solutions, the latter also reproduces the CO(3–2)/HCN(1–0) line ratio of ∼17 ± 4 (which can be reasonably assumed to be an additional constraint on the denser SF gas phase7). Using the upper limit of the HCN(4–3)/(1–0) ratio as a detection (to obtain the densest possible LVG solutions) only pushes the aforementioned set of dense gas solutions to Tkin = (90–105) K, while the lower density/virial ones are now found at Tkin = (110–150) K (both sets of solutions can now reproduce the CO(3–2)/HCN(1–0) ratio).
For a two-phase model where the HCN-bright gas is set as the (h)-phase, we obtain X(2 − ph)co ∼ (0.70–1.75) Xl (with the low values corresponding to the dense/unbound solutions). This is ∼(0.9–2) × Xco (DS98) and yields Mtot ∼ (3.3–8.1) × 109 M☉. Thus, Arp 193 stands as a case where multi-J line observations of high-density tracers such as HCN do not significantly change the picture obtained by the low-J CO lines regarding the global Xco factor. This may be because this LIRG is a rare merger, "caught" in the act of having momentarily dispersed/consumed its dense gas mass while still having large SF-powered IR luminosities, a brief state of affairs is expected because of SF-feedback during the evolution of merger starbursts (Loenen 2009). We note, however, that high-resolution CO imaging revealing the "excess" CO J = 1–0 line emission of the (l)-phase to be a cold SF-quiescent phase can still push the global Xco toward near-Galactic values via a higher X(l)co.
B.18. NGC 5135
Optical and near-IR HST observations reveal this Sy2 galaxy to be a grand design spiral with star formation across its arms but being most intense in its central ∼1 kpc (Martini et al. 2003). The inner 1.6 kpc is also where most of its cm radio continuum emerges (Condon et al. 1996). Optical and UV studies by González Delgado et al. (1998) reveal a young nuclear starburst with gas likely provided by a bar instability. Its HCN/CO J = 1–0 line ratio of ∼0.087 (Gao & Solomon 2004), while not as high as in ULIRGs, is nevertheless ∼(3–4)× higher than in spiral disks. This indicates a significant dense gas mass fraction even in the absence of a strong merger and/or galaxy interaction. Lack of the latter makes the large R10 = 26 and R10 = 13 measured in NGC 5135 exceptions, as these are typical mostly in mergers. Interestingly, this galaxy is known for powerful gas outflows of hundreds of km s−1, driven by its young starburst (González Delgado et al. 1998).
One-phase models of its CO line ratios yield [Tkin, n, Kvir] = [(30–40)K, 3 × 103 cm−3, 22], with warmer (but also more degenerate) solutions such as [(60–110)K, 103 cm−3, 13] also remaining possible. For the entire range of solutions, the corresponding Xco ∼ (0.35–0.45) Xl, similar to X(thin)co(LTE) for Tkin = (30–70) K (the temperatures of the best solutions), as expected for the low/modest optical depths of the LVG solutions. For these Xco values Mtot = (1.1–1.4) × 109 M☉, while MSF = 3 × 108 M☉, which corresponds to ∼20%–30% of the total molecular gas mass. Nevertheless, with the distribution of the molecular gas unknown, a Galactic Xco for disk-distributed gas not intimately involved with star formation remains a distinct possibility. In such a case, the true Xco and Mtot can easily be ∼(10–15) times higher, reducing MSF/Mtot to only a few %, which is more typical of spiral disks. As described in Section 3.4, CO line imaging observations rather than global CO SLEDs can be used to determine the existence of such a cold molecular gas disk.
B.19. Mrk 273
This extraordinary ULIRG shows up as a double-nuclei system in NICMOS near-IR observations where the nuclei are only ∼1'' (740 pc) apart (Scoville et al. 2000). A strikingly long tidal tail (∼1', 44 kpc) and a decoupling of stellar and gas motions (Tacconi et al. 2002) are the telltale signatures of a strong merger. Its northern nucleus contains a compact hard X-ray luminous Sy2 AGN (Xia et al. 2002), but the dust emission SED of this ULIRG is dominated by a starburst (Farrah et al. 2003). This galaxy actually contains the most extreme starburst region among all ULIRGs imaged in the DS98 study, with an IR luminosity of ∼6.7 × 1011 L☉ emanating from its core of only ∼035 × (<0
2) (260 pc × (<149 pc)) diameter. This makes the compact nuclear CO line source of Mrk 273 akin to the west nucleus of Arp 220, and along with Arp 193 these three ULIRGs host the most prodigious SF events in the local universe (DS98). For the core of Mrk 273, the IR brightness is σIR ∼ LIR/(πR2co) ∼ 1013 L☉ kpc−2, i.e., the Eddington limit for radiation-pressure-regulated star formation (Thompson 2009). In the framework of an Eddington-limited SF, this makes the compact nuclear starburst in Mrk 273 a maximal SF event, and its astounding CO line width of ∼1060 km s−1 (DS98) may be a direct outcome of radiation pressure becoming so dynamically important as to affect bulk gas motions.
The global CO line ratios, while typical of a star-forming ISM, are not typical of an extreme starburst, as Mrk 273 certainly is. The R21 = 7 ± 2 ratio in particular is low, found mostly for GMCs in SF-quiescent spirals (R21 ⩾ 20 is observed for extreme starbursts). The compact starburst core of Mrk 273 is surrounded by extended CO line emission (∼3''–7'', i.e., (2.2–5.1) kpc; DS98) where ordinary star-forming and even SF-quiescent ISM could contain significant fractions of the total molecular gas mass. The optimum LVG solutions obtained for the global CO ratios are [Tkin, n, Kvir] = [30–70,3 × 102, 1]. Apart from the elevated temperatures, such conditions are typical of Galactic GMCs, while the Xco ∼ 2 Xl of the best LVG solution (at Tkin = 45 K) is higher than the so-called ULIRG value (∼0.8 Xl). The corresponding Mtot ∼ 1010 M☉, while a minimum mass of MSF ∼ 3.3 × 109 M☉ is needed to fuel an Eddington-limited star formation in this system.
The total molecular gas mass of Mrk 273 can be higher still if the mass contributions of a colder low-density and SF-quiescent phase and a warmer denser SF one are accounted separately. A clear indication of a highly excited SF gas phase is given by the CO J = 6–5 line, which, while not as bright as in some other (U)LIRGs (e.g., Mrk 231, NGC 6240), yields an r65 that is ∼5× higher than that anticipated from the best LVG fit of the lower-J CO lines. A high HCN/CO J = 1–0 ratio of rHCN/CO = 0.14 (using the CO J = 1–0 value from Paper I and the HCN J = 1–0 from Graciá-Carpio et al. 2008) and a well-excited HCN J = 3–2 line with r32(HCN) = 0.49 ± 0.11 (Graciá-Carpio et al. 2008) offer more evidence for the presence of a dense gas phase. A two-phase decomposition using only the CO 3–2, 6–5, and 13CO J = 2–1 lines to constrain the high-excitation phase yields Mh − ex(H2) ∼ 6 × 109 M☉ and an effective X(2 − ph)co ∼ 2.5 Xl. The latter yields Mtot(H2) = 1.3 × 1010 M☉, of which ∼53% corresponds to the low CO brightness (l)-phase. Thus, despite its spectacular starburst, Mrk 273 is an example of an ULIRG that also contains large amounts of cooler low CO-excitation gas. Use of the two available HCN lines to determine the properties of the (h)-phase does not change this picture and can actually raise X(2 − ph)co up to ∼(4–5.5) Xl.
B.20. 3C 293
This powerful radio galaxy was the first F-R II source (i.e., with edge-brightened radio lobes; see Fanaroff & Riley 1974) to be detected in CO locally (z = 0.046), revealing large amounts of molecular gas distributed in a rotating disk of 7'' (∼6.2 kpc) centered in its nucleus, and with a large CO line width of ∼900 km s−1 (Evans et al. 1999). Its disturbed morphology may be due to an interaction with a companion galaxy ∼40''(35 kpc) to the southwest, while a powerful optical/IR-luminous jet interacts with the ambient ISM (Floyd et al. 2006). The SF efficiency of the galaxy is low (∼8 L☉/M☉), and along with an SFR ∼ (6–7) M☉ yr−1, it is typical of ordinary spirals. The modest global CO r21 = 0.74 and r32 = 0.44 ratios (Paper I) are also consistent with an SF-quiescent ISM. Thus, the high-excitation levels of the CO J = 4–3, and 6–5 discovered in this system were certainly a surprise. Their interpretation as being due to an AGN-driven jet–ISM interaction provided the first known example where "mechanical" AGN feedback globally affects galaxy-sized molecular gas reservoirs (Papadopoulos et al. 2008, 2010b), though similar examples with lower-power AGNs and much smaller molecular gas reservoirs were reported much earlier (Matsushita et al. 2004).
The physical conditions compatible with the luminous CO J = 4–3, 6–5 lines were studied in detail by Papadopoulos et al. (2010b; see their Table 4), where dense (∼3 × (104–105) cm−3), warm Tkin ∼ (75–300) K and strongly unbound Kvir ∼ 15–50 states have been deduced. The corresponding X(h)co ∼ (0.8–1.5) Xl, with computed r(h)43 = 2–3.2 and = (1/r(h)43) × L'43 = (1.46–2.33) × 109 Ll. This AGN-excited gas will have Mh − ex ∼ (1.1–3.5) × 109 M☉, which is much higher than the MSF ∼ 5 × 107 M☉ of warm and dense gas expected to be fueling its star formation. Setting a Galactic X(l)co = 5 Xl for the remaining (l)-phase CO J = 1–0 luminosity of the SF-quiescent disk phase yields an effective X(2 − ph)co = (2.9–3.9) Xl, which are near-Galactic values. Indicatively, a one-phase fit of only the CO 1–0, 2–1, and 3–2 lines (any LVG fit including the CO J = 4–3 and/or J = 6–5 predictably fails) yields maximum values of Xco = (1.2–1.6) Xl, obtained for the solutions with the lowest Kvir values (∼2–4), but values as low as Xco ∼ 0.5 Xl are also probable.
B.21. IRAS 14348−1447
This is an impressive ULIRG/merger of two gas-rich spirals with their two nuclei 34 (5.2 kpc) apart and clear indications of tidal features and strongly disrupted disks seen in HST optical and near-IR NICMOS images (Evans et al. 2000; Scoville et al. 2000). Interferometric imaging of CO J = 1–0 has shown that both nuclei contain copious amounts of molecular gas but remain unresolved with size ≲2
5 (3.8 kpc) (Evans et al. 2000). Using radio continuum measurements to obtain size estimates for the two star-forming cores of this ULIRG yields very compact regions with diameters of D ∼ 200 pc (Condon et al. 1991), similar to the compact molecular gas disks found in Arp 220 and Mrk 231 (DS98).
The observed CO J = 1–0, 2–1, 3–2, and 13CO J = 2–1 lines (Paper I) are compatible with a wide range of conditions having Tkin ≳ 35 K, with the best ones found for Tkin = (40–85) K, n = 103 cm−3, and Kvir = 4. Quite unlike most merger/ULIRGs, all good LVG solutions correspond to Kvir ∼ 1–4. Nevertheless, Xco ∼ (0.65–1) Xl, which is much lower than Galactic. The corresponding Mtot ∼ (1.1–1.74) × 1010 M☉, while the minimum mass needed for Eddington-limited star formation is MSF ∼ 5.6 × 109 M☉, which amounts to ∼30%–50% of the total molecular gas mass. In IRAS 14348−1447, as in other ULIRGs, such high-mass fractions of what is presumably a dense and warm gas phase are not reflected by the global CO SLED and 13CO lines available, dominated by lower-density gas. Unfortunately, neither high-J CO nor HCN line observations exist for this ULIRG to set independent constraints on the mass and physical properties of its dense SF molecular gas. A two-phase model with an assumed (h)-phase CO SLED (Section 2.4), while not necessary in this case (as a wide range of good one-phase fits exists), can be used to assess the impact of a massive dense gas phase on Xco. Apart from n ≲ 103 cm−3, the (l)-phase remains essentially unconstrained (as expected), with X(l)co ∼ (0.5–1.12) Xl. For the computed ρ(l − h)co = 0.85 this yields X(2 − ph)co = (1.4–1.7) Xl.
B.22. Zw 049.057
This galaxy has an SF-powered L(*)IR ∼ 1.2 × 1011 L☉ typical for SF spiral disks (e.g., NGC 7469) and is one of the lowest IR luminosity galaxies in the IRAS BGS. NICMOS near-IR imaging with the HST indeed shows a highly inclined disk-dominated system with a heavily dust-enshrouded nucleus (Scoville et al. 2000). Despite its disk-like appearance, its near-IR emission is better fitted by an r1/4 rather than by an exponential disk profile (Scoville et al. 2000), while its LIR/LB ratio is almost as extreme as the much more IR-luminous Arp 220 (Planesas et al. 1991). Early interferometric images of CO J = 1–0 found a large molecular gas mass within a 1.3 kpc sized region (see Planesas et al. 1991 for the cosmology adopted here).
Its CO SLED remains well excited up to J = 4–3 and also has substantial J = 6–5 emission (see Paper I), while both its 13CO J = 1–0, 2–1 lines are detected and yield R10 ∼ 16 and R21 ∼ 24, which is typical of merger-driven starbursts rather than disk-dominated LIRGs. One-phase LVG models reproduce all CO, 13CO line ratios up to CO J = 4–3, though they still underpredict the luminosity of the latter by ∼1.2σ. Unfortunately, even with this large set of lines, significant degeneracies remain. Indicatively, the two best solutions, [Tkin, n, Kvir] = [35, 3 × 103, 22], [105, 103, 13], yield CO line ratios [r21, r32, r43, r65, R10, R21]lvg = [1.05, 0.75, 0.41, 0.05, 22, 21], [1.05, 0.76, 0.40, 0.02, 21, 16]. Much warmer gas with [Tkin, n, Kvir] = [130–140, 103, 4] is also possible and yields [r21, r32, r43, r65, R10, R21]lvg = [1.06, 0.86, 0.60, 0.17, 14, 13], which is now in better agreement with the observed r65 (even if not used in the fit) but is a rather worse fit of R21. Nevertheless, the corresponding Xco values span a rather narrow range ∼(0.35–0.6)Xl, yielding Mtot ∼ (3–5.2) × 108 M☉. This is comparable to the minimum molecular gas mass required for Eddington-limited star formation of MSF(H2) ∼ 4.7 × 108 M☉, yet all the densities deduced from the LVG solutions of the global CO SLED are far from being representative of what is typically a much denser SF phase with small/modest Kvir values.
A two-phase decomposition of the average ISM state using the CO J = 3–2, 4–3, 6–5, and the 13CO transitions to determine the (h)-state converges to [Tkin, n, Kvir] = [110–140,103,4], with Tkin = 140 K corresponding to the best solution (and X(h)co = 0.6 Xl). Higher density solutions with n = 104 cm−3 exist over Tkin = (40–65) K but represent poorer fits and correspond to unbound states with Kvir ∼ 13. This is a general aspect of all (h)-phase LVG solutions (e.g., even if only the CO J = 4–3, 6–5, and 13CO lines are used), namely, the best fits occur at higher temperatures, lower densities, and more gravitationally bound states. High-density solutions (∼104 cm−3), which are more typical for the presumably star-forming (h)-phase, are found at lower temperatures (∼(35–70) K) and strongly unbound states (Kvir ∼ 13–40), but yield poorer fits. Moreover, the high Kvir of the denser/cooler LVG solutions yield similarly low X(h)co (e.g., for Tkin = 50 K, n = 104 cm−3, and Kvir ∼ 13, it is X(h)co = 0.7 Xl).
It is the combination of weak 13CO J = 1–0, 2–1 and well-excited high-J CO lines that nevertheless still has rJ + 1 J < 1, which forces LVG solutions to a parameter space with low CO line optical depths and modest gas densities. This is why Kvir is high toward the low-Tkin/high-n domain (as to keep CO line optical depths low), and why the need for high Kvir's is relaxed toward higher Tkin, which now becomes partly responsible for the low optical depths. Average gas densities toward the high Tkin regime cannot be high, as this would yield rJ + 1 J > 1 in the optically thin domain. If we omit the CO J = 6–5 line from the fit (as its luminosity can be highly uncertain) and also leave out the 13CO J = 1–0 line (which may have a larger contribution from a cooler disk phase), we obtain LVG solutions with densities of ∼(1–3) × 104 cm−3, which are more typical of SF gas, yet with Kvir ∼ 13–22 high enough to yield a low corresponding X(h)co ∼ (0.65–0.75) Xl. Moreover, with an essentially unconstrained (l)-phase and no evidence for a cold disk, a two-phase gas would yield X(2 − ph)co similar to that obtained from one-phase model.
Thus, the molecular gas reservoir in this LIRG is expected to be dominated by its SF component, yet neither the average densities nor the strongly unbound dynamic states deduced from its available global CO SLED are typical of the latter. If this is confirmed by future HCN or other heavy rotor molecular line observations (or more high-J CO and 13CO transitions), it may come to resemble the average ISM state deduced for Arp 193.
B.23. Arp 220 and NGC 6240
These are the two (U)LIRGs in our sample that currently have the best molecular line data tracing their dense gas (i.e., multi-J HCN, CS, HCO+ lines), which are used in a detailed study of its physical conditions (Greve et al. 2009). The presence of a massive dense gas phase in both of them is well established with large HCN/CO J = 1–0 line ratios (∼0.19(Arp 220), ∼0.08(NGC 6240)), well-excited HCN and CS higher-J lines, yet also the large CO/13CO line ratios typical for merger-driven extreme starbursts (∼43–45 for J = 1–0). The latter implies very low CO line optical depths, and thus runs counter to what is expected for a dominant high-density molecular gas reservoir (τJ + 1, J(CO) ≫ 1), necessitating the two-phase models used in such systems (Aalto et al. 1995; Papadopoulos & Seaquist 1998; this work).
Predictably, one-phase models of the CO and 13CO lines (see Paper I) fail to converge on any good set of solutions for both galaxies, yielding [Tkin, n, Kvir] = [(30–90) K, 103 cm−3, 40] (Arp 220) and [Tkin, n, Kvir] = [(15–40) K, 3 × 103 cm−3, 40] (NGC 6240). For Arp 220, even the CO J = 1–0, 2–1, 3–2 lines are impossible to fit with one phase since r21 = 0.67 ± 0.07 (see Paper I) indicates a diffuse gas phase that leaves even the J = 2–1 line subthermally excited, while r21 = 0.97 ± 0.14 marks the emergence of another denser and warmer phase from J = 3–2 and higher. The corresponding one-phase Xco ∼ 0.3 Xl for both systems, a low value due to the very large Kvir values "forced" by the extreme large CO/13CO line ratios obtained for these systems. This is comparable to X(thin)co(LTE) (for the Tkin = (30–40) K range of one-phase LVG solutions), as expected for optically thin CO line emission.
This low Xco factor yields Mtot ∼ 1.85 × 109 M☉(Arp 220) and ∼2.5 × 109 M☉(NGC 6240), while the minimum molecular gas mass needed for fueling Eddington-limited SF in these extreme merger/starbursts is MSF ∼ 4 × 109 M☉(Arp 220) and ∼1.5 × 109 M☉(NGC 6240). Given that MSF/Mtot ≳ 0.6, and in the case of Arp 220 actually >1, it becomes obvious, even in the absence of heavy rotor molecular line observations that the true Xco factor for these two galaxies must be higher. From the analysis detailed in Section 3.2.1, the multi-J HCN LVG solutions in a two-phase LVG model yield X(2 − ph)co ∼ (2.4–4.5) Xl(Arp 220) and X(2 − ph)co ∼ (1–3.3) Xl(NGC 6240), with the high values corresponding to virial dynamic states for the dense gas. The new Mtot estimates are ∼(1.5–2.8) × 1010 M☉(Arp 220) and ∼(0.8–2.8) × 1010 M☉(NGC 6240). In the case of Arp 220, these amount to ∼40%–77% of the dynamic mass of its molecular gas disks (Mdyn from DS98), while for NGC 6240 it surpasses the total mass contained within its central radius of r ≲1'' (480 pc) and argues for significant molecular gas mass corresponding to the CO line emission seen beyond its central CO J = 2–1 peak (see Tacconi et al. 1999 for CO J = 2–1 for a high-resolution interferometry map). In the latter case, the deduced molecular gas mass may actually dominate the mass distribution out to much larger radii than deduced by Tacconi et al. (1999). Multi-J HCN line interferometric maps, along with at least one H13CN transition, at resolutions of ≲1'', will be valuable in determining the XHCN, the effective X(h)co, and X(2 − ph)co of these template merger systems.
B.24. IRAS 17208−0014
This classic ULIRG is the most luminous in our sample. It has a compact gas-rich nucleus (Planesas et al. 1991; DS98), a very disrupted disk, and tidal tails indicating a merger (Murphy et al. 1996; Scoville et al. 2000). In the interferometric study by DS98, this is the ULIRG whose compact gas disk (∼1.4 kpc) has the largest face-on velocity dispersion of σV ∼ 150 km s−1, indicating a highly turbulent gas environment. This is also suggested by the large R21 ≳ 35 ratio, which is expected in the high-pressure, highly turbulent ISM environments of strong mergers (Aalto et al. 1995). Its CO SLED is highly excited up to CO J = 4–3, while the weak CO J = 6–5 reported in Paper I is most likely due to pointing offsets, as ZEUS measurements at the Caltech Submillimeter Observatory (CSO) indicate a much stronger line (Stacey 2011).
No reasonable one-phase LVG fits were found for this source, while attempts to fit the CO J = 1–0 up to J = 4–3 and R21 = 35 yield highly unbound states (Kvir ∼ 13–70). The best such fit, [Tkin, n, Kvir] = [70,103,13], yields r21 = 0.96, r32 = 0.64, r43 = 0.32, the latter being ∼3× smaller than observed. The large L(*)IR of this system corresponds to MSF(H2) ∼ 6 × 109 M☉. On the other hand, Xco ∼ 0.4 Xl computed from the best LVG solution yields Mtot ∼ 5.2 × 109 M☉; thus, even the minimum mass expected for a dense and warm SF-fueling phase will dominate Mtot, as computed by a one-phase Xco factor. One-phase LVG solutions with their low densities and highly unbound dynamic states certainly do not hint at this, while their poor fit of the global CO line ratios of this ULIRG suggests that at least two main gas phases are necessary to represent the average ISM state in this system. The presence of a massive gas phase much denser than those implied by one-phase LVG fits is further corroborated by an rHCN/CO = 0.14 (HCN J = 1–0 from Graciá-Carpio et al. 2008; CO 1–0 from Paper I) and an HCN(3–2)/(1–0) ratio of r32(HCN) = 0.39 (Graciá-Carpio et al. 2008).
Using only CO J = 4–3, 3–2, and R21 = 35 (the lower limit) to constrain the (h)-phase in a two-phase model yields only warm Tkin = (90–150) K and dense n ∼ (3 × 104–105) cm−3 gas phases with Kvir ∼ 13–22 and X(h)co ∼ 0.85–1.40 (high values for the denser gas solutions). These solutions yield r(h)J + 1 J ∼ 2.3–3 for J + 1 = 2, 3, 4, indicating CO lines with small/moderate optical depths, a result of the high temperatures and large Kvir values which, along with the high densities, are necessary for well-excited CO lines up to high-J levels and a high global R21 ratio. The mass of such high-excitation gas can be computed from = (4–4.4) × 109 Ll, which yields Mh − ex(H2) ∼ (3.4–6) × 109 M☉. Thus, as in some other (U)LIRGs studied here (e.g., IRAS 00057+4021), this galaxy contains large reservoirs of dense gas in thermal and dynamic states much more extreme than those expected for dense gas in SF regions. As detailed in Paper I, these could be indicative of other power sources such as turbulent-heated regions (THRs) or CR-dominated regions (CRDR; e.g., Papadopoulos 2010c). Future high-resolution high-J CO, 13CO imaging of this system with ALMA would be very valuable for confirming and quantifying such mechanisms via determining the level of turbulence present in its disk (i.e., measuring σz(r) and the average CR energy density permeating its ISM).
Despite the prominence of the dense highly excited phase in the J = 3–2, 4–3 lines, the (l)-phase remains the main contributor of CO J = 1–0 line luminosity in IRAS 17208−0014, with ∼ (8.7–9) × 109 Ll (∼(66–68)% of the observed one). LVG fits of the corresponding "residual" ratio r(l)21 ∼ 0.5, while Tkin degenerate (as expected), all indicate low gas densities n ∼ (1–3) × 102 cm−3 and a wide range of Kvir ∼ 1–70. The corresponding X(l)co ∼ (0.5–3) Xl (over the Kvir = 1–20 range of LVG solutions) remains essentially unconstrained, with the lowest values corresponding to the most dynamically unbound states, as expected. Thus, for X(h)co = 1.4 Xl (densest gas LVG solutions), we find X(2 − ph)co ∼ (0.75–2.5) Xl.
Using the states compatible with the HCN(3–2)/(1–0) ratio in IRAS 17208−0014 to define the (h)-phase leads to even higher X(2 − ph)co ∼ (2.5–5.9) Xl, with still larger Mh − ex (see Table 1). These correspond to a high-density (∼(3 × 104–105) cm−3) phase with Kvir ∼ 1–6 (unphysical solutions with Kvir < 1 were not considered). Nevertheless, for Tkin = (75–105) K, LVG solutions with high-density gas (∼105 cm−3) but Kvir = 20 are also possible. These strongly unbound dense gas solutions yield X(h)co ∼ 0.8 Xl and similarly low X(2 − ph)co values (unless cold (l)-phase solutions corresponding to an SF-quiescent disk are considered). Observations of more HCN lines and, crucially, of at least one H13CN line can determine whether such extraordinary dynamic states are possible for the HCN-bright dense gas phase (see Section 3.4).
B.25. IRAS 22491−1808
This is an ULIRG with a spectacular morphology involving two nuclei separated by 16 (∼2.3 kpc) (Carico et al. 1990), two tidal tails, and numerous SF knots embedded in those tails (Scoville et al. 2000), indicating an advanced merger. It is also one of the very few ULIRGs where an AGN contributes more than half of the total IR luminosity (Farrah et al. 2003). In our sample it stands out as the galaxy with the most highly excited CO J = 3–2 line with r32 = 1.88, though a large applied correction makes this value somewhat uncertain (see Paper I). All one-phase LVG solutions correspond to dense gas n = (104–3 × 105) cm−3, with the best fits obtained for Tkin ⩾ 40 K. Unfortunately, lack of more CO line ratios and especially at least one 13CO line measurement permits a wide range of [Tkin, n, Kvir] states up to Tkin = 150 K and Kvir ∼ 1–20 to fit the observed ratios. The one-phase Xco values remain essentially unconstrained with Xco ∼ (0.7–2.4) Xl and the low values associated with strongly unbound states (Kvir ∼ 13–22), while the low values with states that have Kvir ∼ 1–7. The corresponding molecular gas mass is Mtot ∼ (6.3–22) × 109 M☉ while MSF ∼ 5.2 × 109 M☉.
Another extraordinary aspect of IRAS 22491−1808 is that many of the conditions compatible with its global CO line ratios indicate dense gas (∼3 × 105) cm−3 with temperatures Tkin = (100–130) K, significantly larger than those of the dust (Tdust = (32–49) K for emissivity of β = 1.5, 2). Such potentially strong decoupling of gas and dust temperatures, especially at high gas densities where Tkin → Tdust is expected, is another indicator of other dominant power sources than PDR photons. Turbulent and CR heating are capable of driving large Tkin/Tdust > 1 inequalities even for the very dense gas mass reservoirs found in ULIRGs (Paper I; Papadopoulos 2010c). These mechanisms can easily dominate when strong gas-rich/gas-rich spiral galaxy interactions drive the bulk of the molecular gas into compact regions in the two nuclei of the progenitors. This will inject large amounts of gas kinetic energy into ∼100 pc sized regions, fueling extreme turbulence, while high SFR densities will establish extreme CRDRs. Interestingly, two other double-nuclei systems, IRAS 12112+305 and IRAS 08572+3915, also show very highly excited (i.e., rJ + 1 J > 1) CO lines.
The IRAS 22491−1808 and similar systems are thus excellent cases for studying extreme ISM conditions and their drivers using ALMA. Such observations will be crucial for: (1) inferring CR energy densities (by finding starburst region sizes), (2) measuring velocity dispersions and the turbulence levels of molecular gas disks, and (3) directly providing key molecular line diagnostics for the presence of CRDRs and/or THRs (Papadopoulos 2010c; Bayet et al. 2011; Meijerink et al. 2011).
B.26. NGC 7469
This is a well-studied Sy 1 spiral galaxy whose AGN is surrounded by a nearly complete ring of powerful starburst activity contributing ∼2/3 of its LIR (Genzel et al. 1995; Riffel et al. 2006, and references therein). Interferometric CO J = 1–0 imaging found its central starburst to be very gas-rich (Meixner et al. 1990; Tacconi & Genzel 1996). Its starburst is thought to be triggered by an interaction with a neighboring galaxy IC 5283 that lies 83'' (∼27 kpc) away (projected distance). An Xco ∼ (1/5)Xco, Gal was inferred for its inner ∼1 kpc and was attributed to the effects of strong SF feedback on molecular clouds (Genzel et al. 1995). A small Xco for the starburst region of the disk in NGC 7469 is indeed corroborated by CO J = 3–2, 1–0 observations and 850 μm/450 μm dust continuum imaging (Papadopoulos & Allen 2000). However, the same observations also revealed faint CO J = 1–0 and 450 μm dust emission extending well beyond the starburst region and containing a massive SF-quiescent, cold dust, and gas reservoir with a Galactic Xco.
These two ISM components make a good one-phase LVG fit impossible for the "cold" r21 = 0.75 and the "warm" r43 = 0.83, r65 = 0.22 ratios observed. The best such solution with Tkin = 70 K, n(H2) = 103 cm−3, Kvir = 4 yields r(lvg)21 = 0.97, r(lvg)32 = 0.75, r(lvg)43 = 0.48, r(lvg)65 = 0.08, and R(lvg)21 = 11. The first two are higher than those observed (∼2σ higher in the case of r21), while for (4–3)/(1–0) and (6–5)/(1–0) the LVG-computed ratios are ∼1.7 and ∼2.75 times smaller than those observed. The Xco = 0.72 Xl value computed from the optimal one-phase LVG fit to the global CO SLED is dominated by the SF phase and corresponds to Mtot ∼ 2.5 × 109 M☉. The minimum SF gas mass expected, on the other hand, is MSF = 6.5 × 108 M☉.
Using only the CO J = 3–2, 4–3, 6–58 and the 13CO J = 2–1 lines to constrain the high-excitation (h)-phase in a two-phase models yields a typical LVG solution for Tkin = 60 K, n(H2) = 104 cm−3, and Kvir = 13 with a corresponding X(h)co = 0.66 Xl. From this solution, we compute = 2.51 × 109 Ll and
= 0.97 × 109 Ll, which yield ρ(l − h)co ∼ 0.39. Then, using the Galactic X(l)co = 5 Xl deduced for the extended CO J = 1–0 and submm continuum dust emission of the disk in NGC 7469 (Papadopoulos & Allen 2000), we obtain X(2 − ph)co ∼ 1.88 Xl, which is ∼2.6× higher than that obtained by the one-phase LVG fit of the global SLED.
Thus, the cold extended disk of NGC 7469, despite containing ∼3× more molecular gas mass than its central starburst, is nearly inconspicuous in the global CO SLED, resulting in a significant underestimate of the total molecular gas mass in this galaxy. Only the available spatial information about its presence could rectify this, which is a state of affairs mirrored in other such systems (e.g., NGC 1068). For disk-dominated LIRGs with strong excitation gradients induced by the presence of central starbursts, only CO, 13CO line, and dust continuum imaging of their ISM can avoid such pitfalls (see Section 3.4). This is quite unlike ULIRGs, where it is the low-excitation "cloud-envelope" phase that contains the smaller mass fraction and is concomitant or closely follows the distribution of a massive much denser gas component.
B.27. IRAS 23365+3604
Near-IR imaging of this LIRG reveals tidal tails indicating a merger, and a single nucleus embedded into a nearly face-on disk that is nearly ∼20 kpc (173) in diameter and shows disturbed spiral structure (Surace et al. 2000). The nucleus is classified as a LINER (Veilleux et al. 1995). The gas dynamics, as revealed by CO J = 1–0 interferometry (DS98), is strongly decoupled from stellar dynamics (Genzel et al. 2001), another hallmark of strong mergers. Its molecular gas disk is compact, with ∼1.2 kpc diameter and a face-on velocity dispersion of ∼100 km s−1 (DS98). Its CO SLED has a "cool" CO(2–1)/(1–0) ratio (=0.75) but a well-excited CO J = 3–2 line with a "warm" CO(3–2)/(1–0) (=0.82), while its large R21(=17) is typical for mergers. One-phase LVG models yield moderately good but rather degenerate fits with [Tkin, n, Kvir] = [30–65, 103, 13] and [70–150,3 × 102, 2.2] being the optimal solution ranges. The best solutions are obtained for Tkin = 50 K, 70 K with corresponding line ratios r(lvg)21 = 0.88, 0.84, r(lvg)32 = 0.56,0.55, r(lvg)43 = 0.24, 0.27, and R(lvg)21 = 20, 16. These are compatible with the measured values, though the models typically yield "warmer" r21 and "cooler" r32 ratios than those observed, in the latter case by ∼1.1σ. The corresponding Xco ∼ (1–1.3) Xl, which gives Mtot ∼ (7.3–9.5) × 109 M☉, while MSF ∼ 2.5 × 109 M☉.
The latter represents a substantial fraction of the total molecular gas mass, where much higher gas densities must be prevailing. A clear indication of another highly excited denser gas phase is provided by the CO J = 6–5 line, which is ∼9–16 times more luminous than those expected from the best LVG solutions. The presence of a dense gas phase able to emit such high-J CO lines is also indicated by a high HCN/CO J = 1–0 line ratio of r(obs)HCN/CO ∼ 0.10 (HCN from Graciá-Carpio et al. 2008; CO from Paper I). Using the CO J = 3–2, J = 4–3 (upper limit), J = 6–5, and 13CO J = 2–1 lines to constrain the high-excitation phase yields [Tkin, n, Kvir] = [40–80, 104, 40] as the optimum solution range, with Tkin = 60 K being the best. The corresponding CO SLED has r(h)21 = 1.94, r(h)32 = 1.90, r(h)43 = 1.50, and r(h)65 = 0.45, which is typical of low/modest optical depths (indicatively τ10 ∼ 0.2, τ32 ∼ 2.5), a result of the exceptionally large Kvir. A less optimal solution range with lower densities and more modest (but still high) Kvir values does exists: [Tkin, n, Kvir] = [90–150, 3 × 103, 22], but it could not reproduce the high HCN(1–0)/CO(6–5) ratio of RHCN/CO65 ∼ 0.48 ± 0.18 observed for this galaxy, as such a low-density phase would hardly emit any HCN J = 1–0 (RHCN/CO65 ≲ 0.08).
The mass of the dense, warm, and strongly unbound phase can be found from X(h)co ∼ 0.45 Xl (for Tkin = 60 K) and ∼ 3.15 × 109 Ll, which yields Mh − ex ∼ 1.4 × 109 M☉. This large gas mass with such high Kvir and high densities is rather surprising since such strongly unbound states are associated with cloud envelopes or intercloud diffuse molecular gas whose n ≲ 103 cm−3. The remaining CO J = 1–0 luminosity of the (l)-phase, on the other hand, is
∼ 4.15 × 109 Ll with an essentially unconstrained X(l)co. Setting X(l)CO = (0.5–5) Xl to encompass the range from a warm, diffuse, and unbound gas component to an underlying cold, self-gravitating, disk phase yields X(2 − ph)co ∼ (0.5–3) Xl and Mtot ∼ (3.7–22) × 109 M☉. The low X(l)CO (and thus Mtot) values are more probable because of the negligible CO J = 2–1 luminosity of the (l)-phase (a cold, self-gravitating, phase would have higher J = 2–1 luminosities).
Using the SNR–GMC interfaces as benchmark entities for the dense yet highly unbound (due to the kinetic energy injected by SNR shocks) molecular gas phase makes clear that a mass fraction of Mh − ex/Mtot ∼ 0.06–0.38 exceeds that expected per GMC for an SNR-impacted gas phase (∼1% of a GMC's mass). It is worth noting that only in the powerful radio galaxy 3C 293 were galaxy-sized molecular gas reservoirs at high densities inferred to be at such strongly gravitationally unbound states, likely caused by the powerful jet–ISM interaction. While no such AGN influence on the ISM is apparent in IRAS 23365+3604, it is interesting to note that it is one of the three ULIRGs (the others being Arp 220 and Mrk 273) where molecular gas velocity fields decoupled from those of stars and ionized gas are found, with molecular gas having ∼2 times larger line widths (Colina et al. 2005). High-J CO, 13CO and HCN and H13CN line observations are necessary for confirming such extraordinary dynamic states for the massive dense gas reservoirs residing in IRAS 23365+3604 and similar systems.
Footnotes
- 6
Xl = M☉ (K km s−1 pc2)−1.
- 7
The non-detection of CO J = 6–5 in this LIRG is likely due to a pointing offset of the JCMT.
- 8
The wide beam of the CSO was used to obtain the CO 6–5 measurement for this extended object.