Abstract
The central mechanisms underlying pain chronicity remain elusive. Here, we identify a reciprocal neuronal circuit in mice between the anterior cingulate cortex (ACC) and the ventral tegmental area (VTA) that mediates mutual exacerbation between hyperalgesia and allodynia and their emotional consequences and, thereby, the chronicity of neuropathic pain. ACC glutamatergic neurons (ACCGlu) projecting to the VTA indirectly inhibit dopaminergic neurons (VTADA) by activating local GABAergic interneurons (VTAGABA), and this effect is reinforced after nerve injury. VTADA neurons in turn project to the ACC and synapse to the initial ACCGlu neurons to convey feedback information from emotional changes. Thus, an ACCGlu–VTAGABA–VTADA–ACCGlu positive-feedback loop mediates the progression to and maintenance of persistent pain and comorbid anxiodepressive-like behavior. Disruption of this feedback loop relieves hyperalgesia and anxiodepressive-like behavior in a mouse model of neuropathic pain, both acutely and in the long term.
This is a preview of subscription content, access via your institution
Access options
Access Nature and 54 other Nature Portfolio journals
Get Nature+, our best-value online-access subscription
$29.99 / 30 days
cancel any time
Subscribe to this journal
Receive 12 print issues and online access
$209.00 per year
only $17.42 per issue
Buy this article
- Purchase on Springer Link
- Instant access to full article PDF
Prices may be subject to local taxes which are calculated during checkout







Similar content being viewed by others
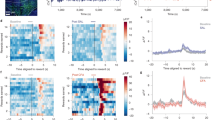
Data availability
The data that support the findings of this study are available from the corresponding author upon reasonable request. Source data are provided with this paper.
Code availability
The present study did not use any customized code or mathematical algorithm.
References
Bliss, T. V., Collingridge, G. L., Kaang, B. K. & Zhuo, M. Synaptic plasticity in the anterior cingulate cortex in acute and chronic pain. Nat. Rev. Neurosci. 17, 485–496 (2016).
Qaseem, A. et al. Nonpharmacologic and pharmacologic management of acute pain from non-low back, musculoskeletal injuries in adults: a clinical guideline from the American College of Physicians and American Academy of Family Physicians. Ann. Intern. Med. 173, 739–748 (2020).
Zhuo, M. Cortical excitation and chronic pain. Trends Neurosci. 31, 199–207 (2008).
Zhuo, M. Long-term potentiation in the anterior cingulate cortex and chronic pain. Phil. Trans. R. Soc. B 369, 20130146 (2014).
Sandkühler, J. Understanding LTP in pain pathways. Mol. Pain 3, 9 (2007).
Gangadharan, V. & Kuner, R. Pain hypersensitivity mechanisms at a glance. Dis. Model. Mech. 6, 889–895 (2013).
Li, X. Y. et al. Alleviating neuropathic pain hypersensitivity by inhibiting PKMζ in the anterior cingulate cortex. Science 330, 1400–1404 (2010).
Woolf, C. J. & Salter, M. W. Neuronal plasticity: increasing the gain in pain. Science 288, 1765–1769 (2000).
Bhave, G. & Gereau, R. W. T. Growing pains: the cytoskeleton as a critical regulator of pain plasticity. Neuron 39, 577–579 (2003).
Kuner, R. & Flor, H. Structural plasticity and reorganisation in chronic pain. Nat. Rev. Neurosci. 18, 20–30 (2016).
Tappe-Theodor, A. & Kuner, R. A common ground for pain and depression. Nat. Neurosci. 22, 1612–1614 (2019).
Zhou, W. et al. A neural circuit for comorbid depressive symptoms in chronic pain. Nat. Neurosci. 22, 1649–1658 (2019).
Zhuo, M. Neural mechanisms underlying anxiety–chronic pain interactions. Trends Neurosci. 39, 136–145 (2016).
Ji, G., Fu, Y., Ruppert, K. A. & Neugebauer, V. Pain-related anxiety-like behavior requires CRF1 receptors in the amygdala. Mol. Pain 3, 13 (2007).
Massaly, N. et al. Pain-induced negative affect is mediated via recruitment of the nucleus accumbens kappa opioid system. Neuron 102, 564–573 (2019).
Koga, K. et al. Coexistence of two forms of LTP in ACC provides a synaptic mechanism for the interactions between anxiety and chronic pain. Neuron 85, 377–389 (2015).
Xiao, X., Ding, M. & Zhang, Y. Q. Role of the anterior cingulate cortex in translational pain research. Neurosci. Bull. 37, 405–422 (2021).
Doan, L., Manders, T. & Wang, J. Neuroplasticity underlying the comorbidity of pain and depression. Neural Plast. 2015, 504691 (2015).
Goffer, Y. et al. Calcium-permeable AMPA receptors in the nucleus accumbens regulate depression-like behaviors in the chronic neuropathic pain state. J. Neurosci. 33, 19034–19044 (2013).
Chen, W. H., Lien, C. C. & Chen, C. C. Neuronal basis for pain-like and anxiety-like behaviors in the central nucleus of the amygdala. Pain 163, e463–e475 (2022).
Zhang, Q. et al. Chronic pain induces generalized enhancement of aversion. eLife 6, e25302 (2017).
Llorca-Torralba, M. et al. Pain and depression comorbidity causes asymmetric plasticity in the locus coeruleus neurons. Brain 145, 154–167 (2022).
Yamauchi, N. et al. Chronic pain-induced neuronal plasticity in the bed nucleus of the stria terminalis causes maladaptive anxiety. Sci. Adv. 8, eabj5586 (2022).
Huang, S. et al. Dopamine inputs from the ventral tegmental area into the medial prefrontal cortex modulate neuropathic pain-associated behaviors in mice. Cell Rep. 31, 107812 (2020).
Mohebi, A. et al. Dissociable dopamine dynamics for learning and motivation. Nature 570, 65–70 (2019).
Carta, I., Chen, C. H., Schott, A. L., Dorizan, S. & Khodakhah, K. Cerebellar modulation of the reward circuitry and social behavior. Science 363, eaav0581 (2019).
Chaudhury, D. et al. Rapid regulation of depression-related behaviours by control of midbrain dopamine neurons. Nature 493, 532–536 (2013).
Matthews, G. A. et al. Dorsal raphe dopamine neurons represent the experience of social isolation. Cell 164, 617–631 (2016).
Markovic, T. et al. Pain induces adaptations in ventral tegmental area dopamine neurons to drive anhedonia-like behavior. Nat. Neurosci. 24, 1601–1613 (2021).
Yang, H. et al. Pain modulates dopamine neurons via a spinal–parabrachial–mesencephalic circuit. Nat. Neurosci. 24, 1402–1413 (2021).
Baliki, M. N. & Apkarian, A. V. Nociception, pain, negative moods, and behavior selection. Neuron 87, 474–491 (2015).
Serafini, R. A., Pryce, K. D. & Zachariou, V. The mesolimbic dopamine system in chronic pain and associated affective comorbidities. Biol. Psychiatry 87, 64–73 (2020).
Kuner, R. & Kuner, T. Cellular circuits in the brain and their modulation in acute and chronic pain. Physiol. Rev. 101, 213–258 (2021).
Mitsi, V. et al. RGS9-2-controlled adaptations in the striatum determine the onset of action and efficacy of antidepressants in neuropathic pain states. Proc. Natl Acad. Sci. USA 112, E5088–E5097 (2015).
Mitsi, V. & Zachariou, V. Modulation of pain, nociception, and analgesia by the brain reward center. Neuroscience 338, 81–92 (2016).
Boersma, K. et al. Efficacy of a transdiagnostic emotion-focused exposure treatment for chronic pain patients with comorbid anxiety and depression: a randomized controlled trial. Pain 160, 1708–1718 (2019).
Huang, S., Borgland, S. L. & Zamponi, G. W. Peripheral nerve injury-induced alterations in VTA neuron firing properties. Mol. Brain 12, 89 (2019).
Wang, C. et al. Synaptotagmin-11 is a critical mediator of parkin-linked neurotoxicity and Parkinson’s disease-like pathology. Nat. Commun. 9, 81 (2018).
Wang, L. et al. Modulation of dopamine release in the striatum by physiologically relevant levels of nicotine. Nat. Commun. 5, 3925 (2014).
Li, C. et al. Modulation of depression-related behaviors by adiponectin AdipoR1 receptors in 5-HT neurons. Mol. Psychiatry 26, 4205–4220 (2021).
Fitzgerald, P. J., Giustino, T. F., Seemann, J. R. & Maren, S. Noradrenergic blockade stabilizes prefrontal activity and enables fear extinction under stress. Proc. Natl Acad. Sci. USA 112, E3729–E3737 (2015).
Chen, T. et al. Top–down descending facilitation of spinal sensory excitatory transmission from the anterior cingulate cortex. Nat. Commun. 9, 1886 (2018).
Hutchison, W. D., Davis, K. D., Lozano, A. M., Tasker, R. R. & Dostrovsky, J. O. Pain-related neurons in the human cingulate cortex. Nat. Neurosci. 2, 403–405 (1999).
Smith, M. L., Asada, N. & Malenka, R. C. Anterior cingulate inputs to nucleus accumbens control the social transfer of pain and analgesia. Science 371, 153–159 (2021).
Ma, Q. A functional subdivision within the somatosensory system and its implications for pain research. Neuron 110, 749–769 (2022).
Meda, K. S. et al. Microcircuit mechanisms through which mediodorsal thalamic input to anterior cingulate cortex exacerbates pain-related aversion. Neuron 102, 944–959 (2019).
Borgkvist, A., Mrejeru, A. & Sulzer, D. Multiple personalities in the ventral tegmental area. Neuron 70, 803–805 (2011).
Bushnell, M. C., Ceko, M. & Low, L. A. Cognitive and emotional control of pain and its disruption in chronic pain. Nat. Rev. Neurosci. 14, 502–511 (2013).
Tracey, I. & Mantyh, P. W. The cerebral signature for pain perception and its modulation. Neuron 55, 377–391 (2007).
Lancon, K., Qu, C., Navratilova, E., Porreca, F. & Seguela, P. Decreased dopaminergic inhibition of pyramidal neurons in anterior cingulate cortex maintains chronic neuropathic pain. Cell Rep. 37, 109933 (2021).
Darvish-Ghane, S., Quintana, C., Beaulieu, J. M. & Martin, L. J. D1 receptors in the anterior cingulate cortex modulate basal mechanical sensitivity threshold and glutamatergic synaptic transmission. Mol. Brain 13, 121 (2020).
Liu, S. et al. Optogenetic activation of dopamine receptor D1 and D2 neurons in anterior cingulate cortex differentially modulates trigeminal neuropathic pain. Mol. Neurobiol. 57, 4060–4068 (2020).
Li, M. et al. Impaired D2 receptor-dependent dopaminergic transmission in prefrontal cortex of awake mouse model of Parkinson’s disease. Brain 142, 3099–3115 (2019).
Wang, L. et al. Cocaine induces locomotor sensitization through a dopamine-dependent VTA–mPFC–FrA cortico-cortical pathway in male mice. Nat. Commun. 14, 1568 (2023).
Missale, C., Nash, S. R., Robinson, S. W., Jaber, M. & Caron, M. G. Dopamine receptors: from structure to function. Physiol. Rev. 78, 189–225 (1998).
Ren, D. et al. Anterior cingulate cortex mediates hyperalgesia and anxiety induced by chronic pancreatitis in rats. Neurosci. Bull. 38, 342–358 (2022).
Gao, S. H. et al. The projections from the anterior cingulate cortex to the nucleus accumbens and ventral tegmental area contribute to neuropathic pain-evoked aversion in rats. Neurobiol. Dis. 140, 104862 (2020).
Sogabe, S., Yagasaki, Y., Onozawa, K. & Kawakami, Y. Mesocortical dopamine system modulates mechanical nociceptive responses recorded in the rat prefrontal cortex. BMC Neurosci. 14, 65 (2013).
Darvish-Ghane, S., Yamanaka, M. & Zhuo, M. Dopaminergic modulation of excitatory transmission in the anterior cingulate cortex of adult mice. Mol. Pain 12, 1744806916648153 (2016).
Schwartz, N. et al. Chronic pain. Decreased motivation during chronic pain requires long-term depression in the nucleus accumbens. Science 345, 535–542 (2014).
Yang, H. et al. Nucleus accumbens subnuclei regulate motivated behavior via direct inhibition and disinhibition of VTA dopamine subpopulations. Neuron 97, 434–449 (2018).
Schapira, A. H. V., Chaudhuri, K. R. & Jenner, P. Non-motor features of Parkinson disease. Nat. Rev. Neurosci. 18, 435–450 (2017).
Cichon, J., Sun, L. & Yang, G. Spared nerve injury model of neuropathic pain in mice. Bio Protoc. 8, e2777 (2018).
Boccella, S. et al. Spared nerve injury as a long-lasting model of neuropathic pain. Methods Mol. Biol. 1727, 373–378 (2018).
Liu, Y. et al. Touch and tactile neuropathic pain sensitivity are set by corticospinal projections. Nature 561, 547–550 (2018).
Zhang, Q. et al. Differential co-release of two neurotransmitters from a vesicle fusion pore in mammalian adrenal chromaffin cells. Neuron 102, 173–183 (2019).
Solie, C., Girard, B., Righetti, B., Tapparel, M. & Bellone, C. VTA dopamine neuron activity encodes social interaction and promotes reinforcement learning through social prediction error. Nat. Neurosci. 25, 86–97 (2022).
Song, Q. et al. Selective phosphorylation of AMPA receptor contributes to the network of long-term potentiation in the anterior cingulate cortex. J. Neurosci. 37, 8534–8548 (2017).
Botta, P. et al. Regulating anxiety with extrasynaptic inhibition. Nat. Neurosci. 18, 1493–1500 (2015).
Zhang, G. W. et al. Medial preoptic area antagonistically mediates stress-induced anxiety and parental behavior. Nat. Neurosci. 24, 516–528 (2021).
Zhu, X. et al. A central amygdala input to the parafascicular nucleus controls comorbid pain in depression. Cell Rep. 29, 3847–3858 (2019).
Keyes, P. C. et al. Orchestrating opiate-associated memories in thalamic circuits. Neuron 107, 1113–1123 (2020).
Shao, J. et al. Cav3.1-driven bursting firing in ventromedial hypothalamic neurons exerts dual control of anxiety-like behavior and energy expenditure. Mol. Psychiatry 27, 2901–2913 (2022).
Zhu, X. et al. Distinct thalamocortical circuits underlie allodynia induced by tissue injury and by depression-like states. Nat. Neurosci. 24, 542–553 (2021).
Zweifel, L. S. et al. Activation of dopamine neurons is critical for aversive conditioning and prevention of generalized anxiety. Nat. Neurosci. 14, 620–626 (2011).
Sellmeijer, J. et al. Hyperactivity of anterior cingulate cortex areas 24a/24b drives chronic pain-induced anxiodepressive-like consequences. J. Neurosci. 38, 3102–3115 (2018).
Vandewauw, I. et al. A TRP channel trio mediates acute noxious heat sensing. Nature 555, 662–666 (2018).
Xu, Z. Z. et al. Inhibition of mechanical allodynia in neuropathic pain by TLR5-mediated A-fiber blockade. Nat. Med. 21, 1326–1331 (2015).
Chaplan, S. R., Bach, F. W., Pogrel, J. W., Chung, J. M. & Yaksh, T. L. Quantitative assessment of tactile allodynia in the rat paw. J. Neurosci. Methods 53, 55–63 (1994).
Beaulieu-Laroche, L. et al. TACAN is an ion channel involved in sensing mechanical pain. Cell 180, 956–967 (2020).
Kraeuter, A. K., Guest, P. C. & Sarnyai, Z. The open field test for measuring locomotor activity and anxiety-like behavior. Methods Mol. Biol. 1916, 99–103 (2019).
Kraeuter, A. K., Guest, P. C. & Sarnyai, Z. The elevated plus maze test for measuring anxiety-like behavior in rodents. Methods Mol. Biol. 1916, 69–74 (2019).
Bourin, M. & Hascoet, M. The mouse light/dark box test. Eur. J. Pharmacol. 463, 55–65 (2003).
King, T. et al. Unmasking the tonic–aversive state in neuropathic pain. Nat. Neurosci. 12, 1364–1366 (2009).
De Felice, M. et al. Capturing the aversive state of cephalic pain preclinically. Ann. Neurol. 74, 257–265 (2013).
Acknowledgements
We thank M. Zhuo (Xi’an Jiaotong University, China) for CaMKIICre-transgenic mice and insightful comments, W. Zeng (Tsinghua University, China) for ThCre-transgenic mice, M. Luo (National Institute of Biological Sciences, China) for ThGFP-transgenic mice, Q. Yang (the Fourth Military Medical University) for optogenetics-coupled optical fiber photometry recordings, Y. Hao (Xi'an Jiaotong University, China) for assistance in confocal imaging, E. Wang (I-Harbour High School Affiliated to Xi'an Jiaotong University, China) for artwork and I.C. Bruce (Peking University) and C. Luo (the Fourth Military Medical University, China) for reading the manuscript. This work was supported by the National Natural Science Foundation of China (to C.W. (32171233, 31670843 and 31400708), X.K. (81974203), H.X. (81901308), Q.S. (32000704) and Y.C. (32300819)), the NIH–NINDS and NIDCR (to F.W., NS 091296 and DE 029946), the Natural Science Foundation of Shaanxi Province of China (to C.W. (2023-ZDLSF-23, 2021TD-37 and 2019JC-07), Q.S. (2020-JC-QN-1423) and N.D. (2023-JC-QN-0236)), the Natural Science Foundation of Sichuan Province of China (to X.K. (2020YJ0337) and H.X. (2020YJ0378)), the Sichuan Science and Technology Program (to X.K., 2022YFS0615), the Shaanxi Postdoc Funding (to H.X. (2023BSHTBZZ15), Y.C. (2023BSHYDZZ39) and C.Z. (2023BSHEDZZ67)) and the China Postdoctoral Science Foundation (to Q.S. (2018M640972) and N.D. (2022M712543)).
Author information
Authors and Affiliations
Contributions
C.W., F.W., X.K. and Z.C. conceived the study and designed experiments with the help of Y.J., Q.Q., T.C. and Z.Z.; Q.S., A.W., H.X. and Y.G. designed studies and performed most experiments and data analysis; N.D., C.Z., X.D. and X.L. conducted some of the behavior experiments and data analysis; Q.W., M.G. and S.S. performed two-photon Ca2+ imaging and data analysis; Y.C. and B.W. performed some of the whole-cell patch and amperometric DA recording; J.H., J.Y., H. Wang., H.L. and X.W. performed some virus injections and stereotaxic surgery. Z.J., Y.Y. and S.H. performed some of the optogenetic behavior experiments; A.Z., H. Wu., X.C. and Y.Q. performed some of the immunochemistry experiments. C.W., A.W., F.W., X.K., Z.C. and Q.S. wrote the manuscript. All authors reviewed the manuscript and approved its submission.
Corresponding authors
Ethics declarations
Competing interests
The authors declare no competing interests.
Peer review
Peer review information
Nature Neuroscience thanks Jose Moron and the other, anonymous, reviewer(s) for their contribution to the peer review of this work.
Additional information
Publisher’s note Springer Nature remains neutral with regard to jurisdictional claims in published maps and institutional affiliations.
Extended data
Extended Data Fig. 1 SNI mouse model shows pain and anxiodepression-like behaviors.
a, Statistics of thermal pain response of Sham and SNI mice (Sham, n = 7; SNI, n = 8 mice). b, Representative tracking maps in the OFT (upper) and EPM (lower) tests of Sham and SNI mice. c, Statistics of locomotion of Sham and SNI mice (Sham, n = 8; SNI, n = 8 mice). d-f, Statistics of the dark-light box (DLB, d), sucrose preference test (SPT, e), and forced swimming test (FST, f) of Sham (n = 7) and SNI (n = 8 for DLB, n = 7 for SPT and FST) mice. g, Sample traces (left) and statistics (right) of current injection-evoked APs of lateral VTADA neurons of TH-GFP mice 6 weeks after SNI (Sham, n = 8; SNI, n = 10 cells from 3 pairs of mice). h, Schematic of the CFE recordings of DA release in the NAc and the mPFC. Left, schematic of the CFE recording of DA release in the NAc. Right, schematic of the injection of Cre-dependent chemogenetic activation virus (DIO-hM3Dq) in the VTA of TH-Cre mice and CFE recording of DA release in the mPFC in response to CNO. i, Sample traces (Iamp and current integration) and statistics of DA release in response to CNO application (100 \({\rm{\mu }}\)M, 7 s) in the mPFC 6 weeks after SNI (Sham, n = 5; SNI, n = 6 slices from 3 pairs of mice). j, Statistics of OFT behavior of SNI (n = 7) versus Sham (n = 7) mice 7 days after SNI surgery. k, Statistics of VTADA neuron firing rate of SNI versus Sham mice 7 days after SNI surgery (Sham, n = 12 neurons from 3 mice; SNI, n = 11 neurons from 3 mice). The data are presented as the mean ± s.e.m. Unpaired Student’s t-test, *P < 0.05, **P < 0.01, ***P < 0.001. Detailed statistics information can be found in the Source Data.
Extended Data Fig. 2 VTADA neurons modulate hyperalgesia and anxiodepression-like behaviors in neuropathic pain.
a, Upper, schematic of the injection of Cre-dependent chemogenetic inhibition virus (GFP) into the lateral VTA of TH-Cre mice. Lower, representative trace of AP recording from VTADA neurons in response to CNO application. b, Representative micrograph showing the co-localization of GFP and TH-staining in the VTA. Scale bar, 500 μm. c–g, Statistics of mechanical (c) and thermal (d) pain, OFT (e), EPM (f), and DLB (g) behaviors of the chemogenetic inhibitory group and control mice (Von Frey, cold/hot plate, and OFT: Control, n = 9; hM4Di, n = 10; EPM: Control, n = 11; hM4Di, n = 10 mice; DLB: Control, n = 8; hM4Di, n = 10 mice). h, Left, schematic showing the injection of chemogenetic activation virus into the VTA of TH-Cre mice with SNI. Right, representative trace of AP recording of VTADA neurons in response to CNO application. i-o, Statistics of mechanical (i) and thermal (j) pain, OFT (k), EPM (l), DLB (m), SPT (n), and FST (o) behaviors of the chemogenetic excitatory group and control virus-injected mice with SNI (Von Frey: Control, n = 8; hM4Di, n = 8; Cold/Hot plate: Control n = 13, hM4Di n = 15; EPM: Control, n = 8; hM4Di, n = 8; OFT: Control, n = 9; hM4Di, n = 10 mice; DLB: Control n = 8; hM4Di n = 9 mice, SPT and FST: Control n = 7, hM4Di n = 7 mice). The data are presented as the mean ± s.e.m. Unpaired Student’s t-test, *P < 0.05, **P < 0.01, ***P < 0.001. Detailed statistics information can be found in the Source Data.
Extended Data Fig. 3 Inhibition of the ACCGlu–VTA projection relieves hyperalgesia and anxiety-like behaviors in neuropathic pain.
a,b, Schematic and representative micrograph showing the retrograde labeling of ACC neurons by injection of retrograde virus (mCherry) into the VTA. Scale bar, 300 μm. Statistics are shown on the right. c, Schematic of the injection of optogenetic inhibition virus (DIO-eNpHR) into the ACC and optical fiber delivery of L-stim (589-nm yellow light) in the VTA of SNI mice. d, e, Statistics of mechanical and thermal pain responses in the chemogenetic inhibitory and control groups. (Von Frey: Control, n = 8; NpHR, n = 8 mice; Cold/Hot plate, Control, n = 12; NpHR, n = 13 mice). f, Representative AP trace of a ACCGlu neuron in response to light stimulation (589 nm). g, h Statistics of OFT (e), and EPM (f) tests in the NpHR group and controls (OFT: Control, n = 11; NpHR, n = 11; EPM: Control, n = 10; NpHR, n = 10 mice). The data are presented as the mean ± s.e.m. Unpaired Student’s t-test, *P < 0.05, **P < 0.01, ***P < 0.001. Detailed statistics information can be found in the Source Data.
Extended Data Fig. 4 The ACCGlu–VTA projection mediates hyperalgesia and anxiodepressive-like behaviors in neuropathic pain.
a, Schematic showing the specific chemogenetic activation of ACCGlu neurons. b-h, Statistics of mechanical (b) and thermal (c) pain responses, OFT (d), and EPM (e), DLB (f), and CPA (conditioned place aversion, g,h) tests in the chemogenetic excitatory group and controls (Von Frey and Cold/Hot plate: Control, n = 14; hM3Dq, n = 13; OFT: Control, n = 14; hM3Dq, n = 13; EPM and DLB: Control, n = 12; hM3Dq, n = 12 mice; CPA: Control, n = 8; hM3Dq, n = 8 mice). i, Schematic showing the specific chemogenetic inhibtion of ACCGlu projection in the VTA in SNI mice. j, Statistics of thermal pain response in the chemogenetic inhibition and control groups (Control, n = 8; hM4Di, n = 8 mice). k, l, Statistics of EPM and CPP (conditioned place preference) tests in the chemogenetic inhibition and control groups (CPP = time in light side – time in dark side; Control, n = 8; hM4Di, n = 8 mice). m-q, Schematic and statistics of pain responses and anxiety-related behaviors of sham mice with (hM4Di) or without (Control) chemogenetic inhibition of ACCGlu-VTA projection (Control, n = 8; hM4Di, n = 8 mice). The data are presented as the mean ± s.e.m. Unpaired Student’s t-test, *P < 0.05, **P < 0.01, ***P < 0.001. Detailed statistics information can be found in the Source Data.
Extended Data Fig. 5 ACCGlu–innervated VTAGABA neurons mediate hyperalgesia and anxiety-like behaviors in neuropathic pain.
a, Left, schematic of the co-injection of CaMKII-Cre virus and the Cre-dependent anterograde trans-monosynaptic virus (rAAV-EF1α-DIO-EGFP-T2A-TK-WPRE-hGH, and H129ΔTK-ubc-tdTomato) into the ACC for the identification of ACCGlu-innervated neurons (GAD67-GFP) in the VTA. Right, representative micrograph showing the localization of anterograde virus (GFP and tdTomato) in ACCGlu neurons. Scale bar, 500 μm. b, Schematic and representative micrograph showing the specific expression of chemogenetic activation virus in ACCGlu neurons. c, Schematic of the injection of chemogenetic activation virus (VGAT1-DIO-hM3Dq) into the VTA and AAV2/1-Cre anterograde virus into the ACC. d-g, Statistics of mechanical (d) and thermal (e) pain responses, OFT (f), and EPM (g) tests following CNO application (0.5 mg/kg, i.p.) of mice receiving hM3Dq-expressing or control virus (Control, n = 8; hM3Dq, n = 8 mice). Behaviors were assessed 6 weeks after SNI. h-l, As in (c-g), except that AAV2/1-Cre anterograde virus was injected into the ACC and GAD67-DIO-hM4Di virus was injected into the VTA in SNI mice (n = 8 for each group). The data are presented as the mean ± s.e.m. Unpaired Student’s t-test, *P < 0.05, **P < 0.01. Detailed statistics information can be found in the Source Data.
Extended Data Fig. 6 VTADA-ACC projections inhibit VTA-projecting ACCGlu neurons via D2R.
a, Schematic and representative micrographs showing the retrograde labeling of VTADA neurons (TH-positive) by the injection of retrograde virus (mCherry) into the ACC. Scale bar, 150 μm. b, Sample traces (left) and statistics (right) showing the spontaneous action potential (AP) firing rate of VTADA neurons labelled by retrograde virus from the ACC of TH-GFP mice 6 weeks after SNI (Sham, n = 20; SNI, n = 20 cells from 4 pairs of mice). c, Schematic of the injection of anterograde (tdTomato) and retrograde (BFP) viruses into the VTA and CaMKII-GFP virus into the VTA of the TH-Cre mice. d, Representative micrograph showing tdTomato-positive neurons in the ACC (tdTomato-labeled ACCGlu neurons). Statistics of tdTomato-labeled glutamatergic (yellow) and non-glutamatergic (red) neurons in the ACC are shown on the right. e, Representative micrograph showing the tdTomato (anterograde) and BFP (retrograde) co-labeled neurons in the ACC. f, Schematic of the co-injection of retrograde virus (mCherry) and Cre-dependent chemogenetic activation virus (GFP) into the VTA of TH-Cre mice. g,h, Representative path-clamp recording traces and statistics of AP frequency of ACCGlu neurons in response to CNO application with or without (ACSF control, n = 23 cells from 4 mice) the treatment of haloperidol (an antagonist of D2R, n = 15 cells from 2 mice) or SCH23390 (an antagonist of D1R, n = 8 cells from 2 mice) in ACC slices. The data are presented as the mean ± s.e.m. Paired Student’s t-test, ***P < 0.001. Statistics are shown on the right. Scale bars, 150 μm. The data are presented as the mean ± s.e.m. Unpaired Student’s t-test, **P < 0.01. Detailed statistics information can be found in the Source Data.
Extended Data Fig. 7 The VTADA–ACC projection modulates anxiodepression-like behaviors and persistent pain.
a, Schematic of the injection of Cre-dependent chemogenetic inhibition virus into the VTA and CNO local administration in the ACC of TH-Cre mice. b-e, Statistics of thermal pain response, EPM, DLB and CPA in the chemogenetic inhibitory and control groups (Cold/Hot plate: Control, n = 10; hM4Di, n = 7; EPM: Control, n = 8; hM4Di, n = 8 mice; DLB: Control, n = 12; hM4Di, n = 11 mice; CPA: Control, n = 8; hM4Di, n = 8 mice). f–j, As in (a-e), except that Cre-dependent chemogenetic activation virus was injected into the VTA of SNI TH-Cre mice and CPP was used for the evaluation of behavior aversion (Control, n = 12; hM3Dq, n = 13 mice; CPP: Control, n = 8; hM4Di, n = 8 mice). k-o, Schematic and statistics of pain responses and anxiety-related behaviors of sham mice with (hM3Dq) or without (Control) chemogenetic activation of VTADA-ACC projection (Control, n = 8; hM3Dq, n = 8 mice). The data are presented as the mean ± s.e.m. Unpaired Student’s t-test, *P < 0.05, **P < 0.01, ***P < 0.001. Detailed statistics information can be found in the Source Data.
Extended Data Fig. 8 Synaptic connections within the ACCGlu–VTAGABA–VTADA–ACCGlu loop.
a, Schematic of virus injection for the functional connection of the ACCGlu–VTAGABA–VTADA–ACCGlu loop. The trans-monosynaptic anterograde-Cre/GFP virus is injected into the ACC to express Cre and GFP in the downstream VTAGABA neurons, which initiates the Cre-dependent retrograde trans-monosynaptic tracing rabies virus (DIO-TVA/RVG and RV-\(\Delta G\)-dsRed) and simultaniously turns on the Cre-dependent expression of WGA-Flpo (DIO-WGA-Flpo) that is trans-synaptically delivered into the downstream DA neurons to start the TH-driving Ch2R/mCherry expression. b, Immunofluorescence showing the co-localization of DsRed- and GFP-positive signals with GAD67 staining. The inset is enlarged on the right. Scale bar, 100 μm. c, Representative micrograph showing that TH-positive fibers densely surround the VTA-projecting ACCGlu neurons, which are labeled by the retrograde virus (DsRed). The inset is enlarged on the right. Scale bar, 40 μm. d, Schematic showing the effect of chemogenetic inhibition of VTADA terminals on VTA-projecting ACCGlu neurons in awake mice. e, Statistics showing the CNO effect on control virus-injected mice (n = 14 cells from 2 mice) as in (d). f, Statistics showing the effects of chemogenetic inhibition of VTADA terminals on VTA-projecting ACCGlu neurons in awake shame (n = 33 cells from 3 mice) vs SNI (n = 33 cells from 3 mice) mice. g, Schematic of virus injection for the functional connection of the ACCGlu–VTAGABA–VTADA–ACCGlu feedback loop. The Cre/GFP-carrying trans-monosynaptic anterograde virus is injected into the ACC to express Cre and GFP in the downstream VTAGABA neurons, which turns on the Cre-dependent expression of WGA-Flpo (DIO-WGA-Flpo) that is trans-synaptically delivered to the downstream DA neurons to start the Flpo-dependent Ch2R/mCherry expression driven by the TH-promoter. h, Statistics of cold/hot plate of the optogenetic activation group and control mice (Control, n = 8; ChR2, n = 8 mice). The data are presented as the mean ± s.e.m. Paired Student’s t-test for (e, f) and unpaired Student’s t-test for (h). n.s., P > 0.05, *P < 0.05, ***P < 0.001. Detailed statistics information can be found in the Source Data.
Extended Data Fig. 9 VTADA neuron excitation leads to a better performance in anxiety-like behaviors at the early stage after SNI surgery.
a, Schematic of the injection of Cre-dependent chemogenetic activation virus (GFP) into the VTA of TH-Cre mice, followed by the SNI surgery. b, Statistics of OFT behavior of chemogenetic activation (n = 7) and control (n = 7) groups 7 days after the SNI surgery. c, d, As in (a, b), except that CaMKII-Cre virus and Cre-dependent optical inhibitory virus were co-injected into the ACC and C57 mice were used (Control, n = 7; NpHR, n = 7). The data are presented as the mean ± s.e.m. Unpaired Student’s t-test, *P < 0.05, **P < 0.01. Detailed statistics information can be found in the Source Data.
Extended Data Fig. 10 Tuning down ACC–VTA–ACC feedback loop relieves hypersensitivity of thermal pain.
a, Statistics of thermal pain behaviors of SNI (nerve ligation removing) (n = 8 mice) vs sham (n = 7 mice), hM4Di vs control virus-injected (in the VTA) SNI mice (with nerve ligation removed), and hM3Dq vs control virus-injected (in the ACC) SNI mice (with nerve ligation removed) 3 weeks after repetitive CNO application (every second day for 2 weeks, n = 6 mice for each). b, Schematic of quinpirole local administration in the ACC (1 µg/µl, 200 nl) of SNI mice. c, Statistics of thermal pain behaviors of SNI mice following the local application of quinpirole vs saline (n = 7 mice for each). d, e, As in (b, c), except that haloperidol (50 µM, 200 nl) was applied in the VTA (saline, n = 7 mice; haloperidol, n = 8 mice). The data are presented as the mean ± s.e.m. Unpaired Student’s t-test, *P < 0.05, **P < 0.01. Detailed statistics information can be found in the Source Data.
Supplementary information
Source data
Source Data Fig. 1
Statistical source data.
Source Data Fig. 2
Statistical source data.
Source Data Fig. 3
Statistical source data.
Source Data Fig. 4
Statistical source data.
Source Data Fig. 5
Statistical source data.
Source Data Fig. 6
Statistical source data.
Source Data Extended Data Fig. 1
Statistical source data.
Source Data Extended Data Fig. 2
Statistical source data.
Source Data Extended Data Fig. 3
Statistical source data.
Source Data Extended Data Fig. 4
Statistical source data.
Source Data Extended Data Fig. 5
Statistical source data.
Source Data Extended Data Fig. 6
Statistical source data.
Source Data Extended Data Fig. 7
Statistical source data.
Source Data Extended Data Fig. 8
Statistical source data.
Source Data Extended Data Fig. 9
Statistical source data.
Source Data Extended Data Fig. 10
Statistical source data.
Rights and permissions
Springer Nature or its licensor (e.g. a society or other partner) holds exclusive rights to this article under a publishing agreement with the author(s) or other rightsholder(s); author self-archiving of the accepted manuscript version of this article is solely governed by the terms of such publishing agreement and applicable law.
About this article
Cite this article
Song, Q., Wei, A., Xu, H. et al. An ACC–VTA–ACC positive-feedback loop mediates the persistence of neuropathic pain and emotional consequences. Nat Neurosci 27, 272–285 (2024). https://doi.org/10.1038/s41593-023-01519-w
Received:
Accepted:
Published:
Issue Date:
DOI: https://doi.org/10.1038/s41593-023-01519-w