Abstract
This study determines the influence of a prenatal high-sucrose (HS) diet on angiotensin II (Ang II)-mediated pressor response and determine the underlying mechanism. Pregnant rats were provided with a 20% sucrose solution diet throughout gestation. Blood pressure and vascular response to Ang II were measured in 5-month-old adult offspring. Currents of L-type Ca2+ channels (Cav1.2) were measured in smooth muscle cells of small mesenteric arteries from the offspring. Ang II-mediated pressor response was higher in the offspring exposed to prenatal high sugar compared with the control. In mesenteric arteries from the HS offspring, AT1 receptors (AT1R), not AT2 receptors, mediated the increased vasoconstriction; protein kinase C (PKC) antagonist GF109203X suppressed the Ang II-increased vasoconstriction; PKC agonist phorbol 12,13-dibutyrate produced a greater contractile response that was reversed by the Cav1.2 antagonist nifedipine. The expression of PKCα was increased, whereas PKCδ was unchanged; KCl-induced vasoconstriction was stronger and was suppressed by nifedipine; nifedipine also reduced the enhanced vasoconstriction by Ang II. In addition, the Cav1.2 of smooth muscle cells in mesenteric arteries from the HS offspring showed larger current density, although its expression was unchanged. The data suggest that a HS diet during pregnancy alters Ang II-mediated pressor response and microvessel tone acting through the PKC/Cav1.2 pathway in the offspring that may in part be because of alterations in AT1Rs, PKCα and Cav1.2.
Similar content being viewed by others
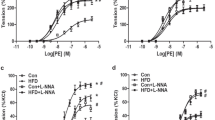
Introduction
Previous studies have shown that many adverse environmental factors during prenatal life may influence the development of adult diseases (for example, hypertension, diabetes mellitus and so on).1, 2, 3 A number of prenatal insults (for example, nicotine, hypoxia, low-protein diets and so on) may ‘program’ certain cardiovascular problems and increase susceptibility to cardiovascular diseases in later life.3, 4, 5, 6 Overconsumption of sucrose during pregnancy has been shown to lead to obesity and glucose intolerance in female mouse offspring as well as hypertension in both sexes1 and may be linked to insulin resistance and hyperglycemia in rats.7 Prenatal high-sucrose (HS) diets may also be responsible for a number of adverse effects on fetal development.2, 8 However, the underlying mechanisms that contribute to the development of hypertension in offspring exposed to high sugar in utero are unclear.
When administered during pregnancy in rats, captopril, an angiotensin-converting enzyme inhibitor, has been shown to prevent the development of hypertension programmed by a maternal low-protein diet, suggesting a key role for the renin–angiotensin system (RAS) in the development of hypertension.3 In addition, a specific AT1 receptor (AT1R) antagonist, losartan, could eliminate the increase in blood pressure (BP) that results from exposure to a maternal low-protein diet in rats, indicating that angiotensin II (Ang II), a critical hormone in the regulation of cardiovascular homeostasis, has an important role in the prenatal programming of hypertension.9 In this study, we investigated BP and pressor responding to Ang II in offspring exposed to prenatal high sugar.
Ang II can stimulate vasoconstriction via three major pathways: release of Ca2+ from intracellular stores, transmembrane influx of Ca2+ predominantly through L-type Ca2+ channels (Cav1.2) and activation of one or more isoforms of protein kinase C (PKC).10 PKC has been shown to increase intracellular Ca2+ via activation of Cav1.2, which in turn induces vasoconstriction.11 Our earlier study demonstrated that overconsumption of sugar during pregnancy resulted in an enhanced vascular response to Ang II.12 White et al.13 showed that the enhanced responsiveness of mesenteric arteries from streptozotocin-diabetic rats to alpha-adrenergic agonists has a greater dependency on extracellular calcium. Other laboratories have demonstrated that aortic vascular smooth muscle cells from a mouse model of type 1 diabetes show an increase in Cav1.2 current.14 Therefore, the present study was designed to test whether the PKC/Cav1.2 pathway is involved in the increased Ang II-mediated pressor response and vasoconstriction in the HS offspring.
Methods
Animals
Pregnant Sprague–Dawley rats from the Animal Center of Soochow University were housed in a controlled environment of 22 °C with a 12-h light/dark cycle. They were randomly divided into two groups: (1) the control group was fed with standard food and (2) the HS group was provided with the same food and 20% sucrose solution from gestational day 1.8 After delivery, all rats were provided tap water and standard rat food for 5 months. All procedures were approved by the Institutional Animal Care Committee and conformed to the Guide for the Care and Use of Laboratory Animals.
Measuring blood glucose and body weight
Pregnant rats (on gestational day 21) as well as the male adult offspring at 5 months of age were anesthetized with a mixture of ketamine (75 mg kg−1) and xylazine (10 mg kg−1; Hengrui Medicine, Jiangsu, China) administered intraperitoneally. Fetal blood samples were collected following decapitation. Maternal and offspring blood samples were obtained from the abdominal aorta. Blood samples were collected into ice-cold plastic tubes containing heparin. Blood glucose was determined by a Nova analyzer (Nova Biomedical, Waltham, MA, USA) and body weight was measured.
Measurement of BP
Offsprings were implanted with catheters in their femoral arteries for recording of BP as described.15 After 3 days of recovery from surgery, BP was measured continuously in conscious and free-moving rats. Following baseline recording for ∼60 min, Ang II (100 ng kg−1; Sigma, St Louis, CA, USA)16 in 0.2 ml saline was injected intravenous via the catheter, and BP was recorded for an additional 120 min using the Power-Lab system and software (AD Instruments, Bella Vista, New South Wales, Australia).
Measurement of vessel tone
Small segments of mesenteric arteries (fourth-order branch) were isolated in Krebs–Henseleit solution (mmol l−1: NaCl 115, NaHCO3 25, KCl 4.6, NaH2PO4 1.2, MgCl2 1.2, CaCl2 2.5 and glucose 10; pH 7.4) and mounted in a multimyograph system (Danish Myo Technology, Midtjylland, Denmark), maintained in oxygenated Krebs–Henseleit solution (95% O2, 5% CO2) at 37 °C.17, 18 After equilibration for 60 min, the vessel rings were contracted with cumulatively increasing concentrations of Ang II (10−11–10−5 mol l−1). Losartan (AT1R antagonist, 10 μmol l−1),19 PD123319 (AT2R antagonist, 10 μmol l−1),19 GF109203X (PKC antagonist, 1 μmol l−1)20 or nifedipine (Cav1.2 antagonist, 10 μmol l−1)21 were used for pretreating segments for 30 min before application of Ang II. Cumulative concentration-response curves to the PKC activator, phorbol 12,13-dibutyrate (PDBu, 10−9–10−5 l−1) or KCl (20–140 mmol l−1) were performed both in the absence and presence of nifedipine in mesenteric arteries. The vessel tone was normalized by the maximum contraction elicited by 60 mmol l−1 KCl or by the maximum response of the vessel to a specific agonist. Each vessel was used once only. Signals were recorded by Power-Lab system with Chart 5 software (AD Instruments, Castle Hill, NSW, Australia).
Isolation of vascular myocytes
Myocytes were isolated enzymatically from dissected mesenteric arteries.22 Vessels were cut into 1-mm strips in ice-cold solution (PSS) containing (mmol l−1) 137 NaCl, 5.6 KCl, 1 MgCl2, 0.42 Na2HPO4, 0.44 NaH2PO4, 4.2 NaHCO3 and 10 HEPES (pH 7.4). All enzymatic solutions contained 1 mg ml−1 bovine serum albumin. Isolated vascular smooth muscle cells were obtained by gentle trituration in PSS. The cells were stored at 4 °C for study within 6 h.
Electrophysiological measurement
Myocytes were plated directly on the bottom of the experimental chamber and visualized using an inverted microscope (OLYMPUS CKX41, Tokyo, Japan). Cells were washed with bath solution to remove debris. Patch pipettes were pulled from thin-wall glass capillary tubes using a micropipette puller (Model P-30, Sutter Instruments, Novato, CA, USA). The tip resistance of patch pipettes was 3∼4 MΩ when filled with pipette internal solution.
Negative inward current through Cav1.2 was evaluated using Ba2+ (IBa) as the charge carrier. The voltage-dependent Ca2+ channel current was isolated via eliminating the K+ current by inclusion of Cs+ in the patch pipette with TEA and 4-AP in the bath solution. T-Ca2+ current was eliminated by using a holding potential of −60 mV. IBa was evoked using several voltage protocols. For activation of the inward current, Ba2+ current was elicited by 250-ms voltage steps from a holding potential of −60 mV to test potentials in the range −50 to +70 mV with 10 mV increments. The activation data were fit to the Boltzmann distribution, G/Gmax=1−{1+exp[(V−V1/2)/k]}−1, where V1/2 is the voltage of half-maximal activation, G is the peak conductance at test voltage V, Gmax is the maximum conductance and k is the slope factor. To estimate the rate of Ca2+ channel inactivation, the voltage dependency of IBa inactivation was determined using a double-pulse protocol with a 1000-ms conditioning voltage step to potentials between −60 and +60 mV with 10 mV increments. This was followed by a 200-ms test pulse to +20 mV to evaluate inactivation of Cav1.2. The inactivation data were fit with a Boltzmann equation of the form I/Imax={1+exp[(V−V1/2)/k]}−1, where I/Imax is the relative current amplitude compared with the maximum current amplitude, k is the slope factor and V1/2 is the voltage at which there is half-maximal inactivation. Data were collected after the whole-cell configuration was obtained and current amplitude stabilized. Only cells with an input resistance >2 GΩ without substantial run-down were analyzed.
Whole-cell voltage-clamp was performed using a computer-controlled current and voltage clamp system (Multiclamp 700B, Digidata 1440, Axon Instruments, Foster City, CA, USA) driven by Clampex 10.1 and Multiclamp Commander 1. Pipette offset and whole-cell capacitance were compensated electronically. The cellular membrane potential was measured at gap-free mode in the whole-cell current-clamp configuration, and the whole-cell current was measured at episodic stimulation mode in the whole-cell voltage-clamp configuration using an Axonpatch 700B amplifier and Clampex 10.1. All of the whole-cell currents were normalized with cell capacitance to give current density.
Western blot analysis
Protein abundance of PKC α, δ and Cav1.2 α1c-subunits in mesenteric arteries were measured with western blot analysis normalized to β-actin.23, 24 The primary antibodies were the goat polyclonal antibody (Santa Cruz Biotechnology, Santa Cruz, CA, USA) against Cav1.2 α1c-subunits (1:200), rabbit polyclonal antibody (Santa Cruz Biotechnology) against PKCα (1:200) or PKCδ (1:200). The secondary antibody was donkey anti-goat antibody (1:4 000) or goat anti-rabbit antibody (1:4 000; Beyotime Biotechnology, Jiangsu, China). Immunosignals were visualized using chemiluminescence detection (Amersham Biosciences, Piscataway, NJ, USA) and the UVP imaging system (EC3-Imaging-System, Upland, CA, USA). Imaging signals were digitized and analyzed, and then the ratio of band intensity to β-actin was obtained to quantify the relative protein expression level.
Data analysis and statistics
Data were expressed as the mean±s.e.m. Significance (P<0.05) was determined by t-test or two-way analysis of variance (ANOVA) followed by a post hoc test, where appropriate. Curve fitting was performed with SigmaPlot 11 (Systat Software, Inc., Chicago, IL, USA) and GraphPad Prism 5 (GraphPad Software, Inc., San Diego, CA, USA).
Results
Blood glucose and body weight
As Table 1 shows, the fetal body weight was heavier in the HS group (P<0.05), whereas no significant difference in body weight was found between the control and HS offspring. Both the fetal and maternal blood glucose levels were higher in the HS group (P<0.05). However, the blood glucose level of the HS offspring was not significantly different from that of the control.
Ang II-induced BP responses
The basal mean arterial pressure showed no significant difference with measurements of 103.3±1.9 mm Hg in the HS offspring and 102.7±1.7 mm Hg in the control. Immediately following intravenous administration of Ang II, mean arterial pressure in the HS offspring was significantly higher than that of the control (P<0.05, Figure 1a).
(a) Ang II-mediated pressor responses in the offspring. Arrow: time of injection of intravenous Ang II. Control (n=10 from five dams), HS: high sucrose (n=12 from five dams). (b) Ang II-mediated vasoconstriction in mesenteric arteries of the offspring (n=5, each group). (c, d) the effect of losartan or PD123319 on Ang II-mediated vasoconstriction in mesenteric arteries of the offspring (n=5, each group). *P<0.05.
Ang II-mediated vasoconstrictions in the mesenteric arteries
Ang II-induced maximum contraction was 3.82±0.59% in the control and 9.52±0.69% in the HS group (P<0.05, Figure 1b). Pretreating vessel rings with losartan completely inhibited Ang II-mediated vascular contractions in both the control and the HS group, whereas PD123319 had no effect (Figures 1c and d). Ang II-induced vasoconstrictions were lower in the presence of GF109203X. GF109203X-inhibited contractile response to Ang II was greater in the HS offspring than in the control (Figure 2a). Nifedipine depressed the Ang II-induced contractile response, and the decreased level in the HS offspring was significantly more than that of the control (Figure 2b). PDBu produced stronger contractions in the HS offspring than the control, and the PDBu-caused difference could be removed by nifediping (Figure 2c). Cumulative concentrations of KCl also caused a significantly higher contractile response in mesenteric arteries of the HS offspring compared with the control, which was reversed by nifedipine (Figure 2d).
(a, b) The effect of GF109203X or nifedipine on Ang II-mediated vasoconstrictions in mesenteric arteries of the offspring (n=5, each group). (c, d), PDBu- and KCl-induced vasoconstriction in the absence and presence of nifedipine in mesenteric arteries of the offspring (n=5, each group). Data in d were expressed as a percentage of the maximum response of that vessel to KCl. *P<0.05; #P<0.05.
The effect of prenatal HS on Cav1.2
Figure 3 shows the peak current–voltage relation for Cav1.2 in myocytes from the offspring. The control or HS traces show that the peak current increased with each voltage step from –50 to +20 mV. The current amplitude was significantly higher in the HS myocytes than in the control, and the maximum current density calculated at +20 mV in the HS myocytes showed a striking increase of 42.83% compared with the control (P<0.05, Figure 3).
Voltage dependence of activation and inactivation of Cav1.2
Figure 4 shows the steady-state activation and inactivation of Cav1.2 in myocytes. The V1/2 of activation occurred at 1.06±0.69 mV for the control and −1.78±0.56 mV for the HS cells (P>0.05). The V1/2 of inactivation occurred at −9.00±1.29 mV for the control and −6.24±1.13 mV for the HS cells (P>0.05). There was no significant difference in voltage dependence of Cav1.2 activation and inactivation between the control and HS cells (Figure 4).
(a, b) Typical inactivation currents obtained by stepping to +20 mV for 200 ms following the application of a series of 1000-ms conditioning potentials from –60 to +60 mV. (c) Voltage dependence of activation curves of the Cav1.2 currents in the control (n=15) and HS cells (n=11) (G, conductance; Gmax, maximum conductance). (d) Voltage dependence of inactivation curves of the Cav1.2 currents in the control (n=22) and HS (n=19) groups (I, current; Imax, maximum current). The representative traces for activation currents are presented in Figure 3 (a) (b).
PKC α, δ isoforms and Cav1.2 α1c subunit expression in mesenteric arteries
Western blot analysis showed no significant difference in expression levels of Cav1.2 α1c subunits and PKCδ isoform in mesenteric arteries between the HS and control group. Higher expression of the PKCα isoform was detected in the HS offspring (Figure 5).
Discussion
In the present study, prenatal HS diet was shown to enhance the Ang II-mediated pressor response and vascular tone, acting through altered PKC/Cav1.2 signaling pathways. Blood glucose was higher in pregnant and fetal rats, and fetal weight was increased in the HS group. However, both blood glucose and body weight were the same for the control and HS groups at 5 months after birth, suggesting that mechanisms may exist for reversal in the offspring if abnormal dieting is corrected. Although baseline BP was not significantly changed, Ang II-stimulated pressor response was increased in the HS offspring. RAS has been suggested to have an important role in the programming of hypertension,9, 25 and PKC/Cav1.2 signal pathways are critical in vasoconstriction and pressor responses. Ang II binds to AT1R to activate phospholipase C, and then hydrolyzes membrane phosphoinositides into diacylglycerol to activate PKC.26, 27 PKC can activate Cav1.2 by direct phosphorylation28 or by altering membrane potential through the inhibition of BK channels.29 The activation of Cav1.2 could lead to extracellular Ca2+ influx and vasoconstriction.
Overconsumption of sugar during pregnancy may lead to an overexpression of AT1R in the offspring.12 In the present study, losartan, a specific antagonist of AT1R, abolished the enhanced vascular response to Ang II in the HS group, whereas the AT2R blocker PD123319 did not show any significant effects on Ang II-mediated contraction, suggesting that AT1R, not AT2R, mediated Ang II-increased vasoconstriction and pressor response in the HS offspring. A high-sugar diet during pregnancy could alter blood values and hormone levels, including glucose and insulin concentrations.7 Known interactions exist between RAS and other endocrine systems related to glucose homeostasis.30 In a previous study, hyperglycemia upregulated the RAS components necessary for the synthesis and actions of Ang II that may contribute to hypertension associated with metabolic syndrome.30 Thus, it is reasonable to propose that biochemical and endocrine changes following prenatal overconsumption of sugar may cause alterations in RAS in the offspring. We also found that GF109203X suppressed the Ang II-increased vasoconstriction, suggesting that the enhanced PKC functions had a role in Ang II-stimulated vasoconstriction in the HS offspring. As an upstream component of the Ang II pathway, overexpressed AT1R may contribute to enhanced PKC functions.
Gestational diabetes mellitus may result in increased activity and enhanced tissue distribution of several PKC isoforms in early organogenesis,31 and hyperglycemia could increase PKC activity in different issues in vitro.32, 33, 34 PKC isoforms, including PKCα, PKCγ, PKCδ, PKCɛ and PKCζ, were detected in the rat mesenteric arteries. Only α and δ isoforms appeared to be involved in PDBu-induced vasoconstriction.24 PKCα can strengthen Ca2+-dependent cellular contraction and its overexpression has been linked to hypertension.35 The present study measured the expression of PKCα and PKCδ to determine whether they were involved in enhanced PKC functions. In addition, PDBu was used to test PKC activity. Overexpression of PKCα, not PKCδ, was found in the HS offspring. It has been previously demonstrated that prenatal hypoxia induces epigenetic programming of the PKCɛ gene in rat heart.36 Thus, epigenetic factors may be one of the underlying mechanisms for the change of PKCα, an area that deserves further investigation. PDBu produced greater vasoconstriction in the HS offspring. In addition, nifedipine reversed the PDBu-enhanced vasoconstriction, indicating that an altered PKC/Cav1.2 pathway may contribute to Ang II-enhanced vessel tone.
To determine whether the altered Cav1.2 was related to the upregulated PKC/Cav1.2 pathway, we used cumulative concentrations of KCl to test the vascular response that was manipulated with the antagonist. High concentration of extracellular K+ depolarizes the cell membrane leading to activation of Cav1.2, resulting in increased influx of Ca2+ and in turn vasoconstriction.20 KCl produced greater vasoconstriction in the mesenteric arteries of the HS offspring in the present study, which was normalized by nifedipine, indicating increased activity of Cav1.2 in the HS offspring. However, there was no significant difference in the expression of Cav1.2 in the mesenteric arteries between the control and the HS offspring, suggesting that the anatomical density of Cav1.2 in the mesenteric arteries might not be altered. Rather, changes in sensitivity of Cav1.2 could be the cause of the increased activity. A consistent finding in the vascular smooth muscle cell from mesenteric arteries was an increase in currents of Cav1.2 in the HS offspring without changes in the voltage dependence of activation or inactivation of Cav1.2. Furthermore, nifedipine partially reduced the Ang II-enhanced vasoconstriction in mesenteric arteries, suggesting that the PKC/Cav1.2 signaling pathway may not be the only route for Ang II-increased vasoconstriction and pressor responses in the HS offspring.
In conclusion, microvessel and cellular experiments suggest potential pathways that may be linked to enhanced activity of the PKC/Cav1.2 route and are associated with alterations in AT1R, PKCα and Cav1.2. These observations may provide a novel insight into mechanisms underlying the Ang II-increased pressor response and vessel tone in the offspring exposed to high sugar prenatally. The information is an important contribution to our understanding of early prevention or treatments of cardiovascular diseases that are programmed in utero.
References
Samuelsson A-M, Matthews PA, Jansen E, Taylor PD, Poston L . Sucrose feeding in mouse pregnancy leads to hypertension, and sex-linked obesity and insulin resistance in female offspring. Front Physiol 2013; 4: 1–11.
Marco LJ, McCloskey K, Vuillermin PJ, Burgner D, Said J, Ponsonby A-L . Cardiovascular disease risk in the offspring of diabetic women: the impact of the intrauterine environment. Exp Diabetes Res 2012; 2012: 1–10.
Sherman RC, Langley-Evans SC . Early administration of angiotensin-converting enzyme inhibitor captopril, prevents the development of hypertension programmed by intrauterine exposure to a maternal low-protein diet in the rat. Clin Sci 1998; 94: 373–381.
Barker DJ, Godfrey KM, Gluckman PD, Harding JE, Owens JA, Robinson JS . Fetal nutrition and cardiovascular disease in adult life. Lancet 1993; 341: 938–941.
Tao H, Rui C, Zheng J, Tang J, Wu L, Shi A, Chen N, He R, Wu C, Li J, Yin X, Zhang P, Zhu Z, Tao J, Xiao J, Mao C, Xu Z . Angiotensin II-mediated vascular changes in aged offspring rats exposed to perinatal nicotine. Peptides 2013; 44: 111–119.
Zhang L . Prenatal hypoxia and cardiac programming. J Soc Gynecol Invest 2005; 12: 2–13.
Hwang IS, Ho H, Hoffman BB, Reaven GM . Fructose-induced insulin resistance and hypertension in rats. Hypertension 1987; 10: 512–516.
Wu L, Mao C, Liu Y, Shi A, Xu F, Zhang L, Xu Z . Altered dipsogenic responses and expression of angiotensin receptors in the offspring exposed to prenatal high sucrose. Peptides 2011; 32: 104–111.
Sherman RC, Langley-Evans SC . Antihypertensive treatment in early postnatal life modulates prenatal dietary in uences upon blood pressure in the rat. Clin Sci 2000; 98: 269–275.
Bauer J, Dau C, Cavarape A, Schaefer F, Ehmke H, Parekh. N . ANG II- and TxA2-induced mesenteric vasoconstriction in rats is mediated by separate cell signaling pathways. Am J Physiol Heart Circ Physiol 1999; 277: H1–H7.
Litten RZ, Suba EA, Roth BL . Effects of a phorbol ester on rat aortic contraction and calcium influx in the presence and absence of BAY k 8644. Eur J Pharmacol 1987; 144: 185–191.
Li S, Fang Q, Zhou A, Wu L, Shi A, Cao L, Zhu H, Liu Y, Mao C, Xu Z . Intake of high sucrose during pregnancy altered large-conductance Ca2+-activated K+ channels and vessel tone in offspring’s mesenteric arteries. Hypertens Res 2012; 36: 158–165.
White R, Carrier G . Enhanced vascular alpha-adrenergic neuroeffector system in diabetes: importance of calcium. Am J Physiol Heart C 1988; 255: H1036–H1042.
Pinho JF, Medeiros MAA, Capettini LSA, Rezende BA, Campos PP, Andrade SP, Cortes SF, Cruz JS, Lemos. VS . Phosphatidylinositol 3-kinase-δ up-regulates L-type Ca2+ currents and increases vascular contractility in a mouse model of type 1 diabetes. Br J Pharmacol 2010; 161: 1458–1471.
Massett MP, Lewis SJ, Stauss HM, Kregel KC . Vascular reactivity and baroreflex function during hyperthermia in conscious rats. Am J Physiol Regul Integr Comp Physiol 2000; 279: R1282–R1289.
Wong PC, Price WA, Chiu AT, Duncia JV, Carini DJ, Wexler RR, Johnson AL, Timmermans PB . Hypotensive action of DuP 753, an angiotensin II antagonist, in spontaneously hypertensive rats. Nonpeptide angiotensin II receptor antagonists: X. Hypertension 1990; 15: 459–468.
Stenman E . Cerebral ischemia enhances vascular angiotensin AT1 receptor-mediated contraction in rats. Stroke 2004; 35: 970–974.
Neidhold S, Eichhorn B, Kasper M, Ravens U, Kaumann A . The function of α- and β-adrenoceptors of the saphenous artery in caveolin-1 knockout and wild-type mice. Br J Pharmacol 2007; 150: 261–270.
Xiao D, Xu Z, Huang X, Longo LD, Yang S, Zhang L . Prenatal gender-related nicotine exposure increases blood pressure response to angiotensin II in adult offspring. Hypertension 2008; 51: 1239–1247.
Nerurkar PV, Leiria LO, Sollon C, Calixto MC, Lintomen L, Mónica FZ, Anhê GF, De Nucci G, Zanesco A, Grant AD, Antunes E . Role of PKC and CaV1.2 in detrusor overactivity in a model of obesity associated with insulin resistance in mice. PLoS ONE 2012; 7: e48507.
Goyal R, Papamatheakis DG, Loftin M, Vrancken K, Dawson AS, Osman NJ, Blood AB, Pearce WJ, Longo LD, Wilson SM . Long-term maternal hypoxia: the role of extracellular Ca2+ entry during serotonin-mediated contractility in fetal ovine pulmonary arteries. Reprod Sci 2011; 18: 948–962.
Eichhorn BM, Muller G, Leuner A, Sawamura T, Ravens U, Morawietz H . Impaired vascular function in small resistance arteries of LOX-1 overexpressing mice on high-fat diet. Cardiovasc res 2009; 82: 493–502.
Liu X, Rusch NJ, Striessnig J, Sarna SK . Down-regulation of L-type calcium channels in inflamed circular smooth muscle cells of the canine colon. Gastroenterology 2001; 120: 480–489.
Ohanian V, Ohanian J, Shaw L, Scarth S, Parker PJ, Heagerty AM . Identification of protein kinase C isoforms in rat mesenteric small arteries and their possible role in agonist-induced contraction. Circ res 1996; 78: 806–812.
Ashton N . Perinatal development and adult blood pressure. Braz J Med Biol Res 2000; 33: 731–740.
Ruan X, Arendshorst WJ . Role of protein kinase C in angiotensin II-induced renal vasoconstriction in genetically hypertensive rats. Am J Physiol 1996; 270: F945–F952.
Sathishkumar K, Yallampalli U, Elkins R, Yallampalli C . Raf-1 kinase regulates smooth muscle contraction in the rat mesenteric arteries. J Vasc Res 2010; 47: 384–398.
Webb RC . Smooth muscle contraction and relaxation. Adv Physiol Educ 2003; 27: 201–206.
Minami K, Fukuzawa K, Nakaya Y . Protein kinase c inhibits the Ca2+-activated K+ channel of cultured porcine coronary artery smooth muscle cells. Biochem Biophys Res Commun 1993; 190: 263–269.
Putnam K, Shoemaker R, Yiannikouris F, Cassis LA . The renin-angiotensin system: a target of and contributor to dyslipidemias, altered glucose homeostasis, and hypertension of the metabolic syndrome. Am J Physiol Heart Circ Physiol 2012; 302: H1219–H1230.
Gäreskog M, Wentzel P . Altered protein kinase C activation associated with rat embryonic dysmorphogenesis. Pediatr Res 2004; 56: 849–857.
Park SH, Woo CH, Kim JH, Lee JH, Yang IS, Park KM, Han HJ . High glucose down-regulates angiotensin II binding via the PKC-MAPK-cPLA2 signal cascade in renal proximal tubule cells. Kidney Int 2002; 61: 913–925.
Hua KF, Wang SH, Dong WC, Lin CY, Ho CL, Wu TH . High glucose increases nitric oxide generation in lipopolysaccharide-activated macrophages by enhancing activity of protein kinase C-alpha/delta and NF-kB. Inflamm Res 2012; 61: 1107–1116.
Park JY, Ha SW, King GL . The role of protein kinase C activation in the pathogenesis of diabetic vascular complications. Perit Dial Int 1999; 19 (Suppl 2): S222–S227.
Salamanca DA, Khalil RA . Protein kinase C isoforms as specific targets for modulation of vascular smooth muscle function in hypertension. Biochem Pharmacol 2005; 70: 1537–1547.
Patterson AJ, Chen M, Xue Q, Xiao D, Zhang L . Chronic prenatal hypoxia induces epigenetic programming of PKC gene repression in rat hearts. Circ Res 2010; 107: 365–373.
Acknowledgements
This study was supported partly by 2012CB947600, NSFC grants (81030006, 30973211, 81070540 and 30902018); grants (BK2009122, CX09B-031Z and 2011BAZ03151) and Jiangsu Province’s Key Discipline/Laboratory of Medicine and ‘ChuangXin TuanDui’ grant, China. We would like to thank technical assistance from Encode Genomics Bio-Technology, Suzhou.
Author information
Authors and Affiliations
Corresponding author
Rights and permissions
About this article
Cite this article
Wu, C., Li, J., Bo, L. et al. High-sucrose diets in pregnancy alter angiotensin II-mediated pressor response and microvessel tone via the PKC/Cav1.2 pathway in rat offspring. Hypertens Res 37, 818–823 (2014). https://doi.org/10.1038/hr.2014.94
Received:
Revised:
Accepted:
Published:
Issue Date:
DOI: https://doi.org/10.1038/hr.2014.94
Keywords
This article is cited by
-
Prenatal hypoxia affected endothelium-dependent vasodilation in mesenteric arteries of aged offspring via increased oxidative stress
Hypertension Research (2019)
-
The angiotensin II receptor antagonist, losartan, enhances regulator of G protein signaling 2 mRNA expression in vascular smooth muscle cells of Wistar rats
Hypertension Research (2016)
-
Chronic fetal exposure to caffeine altered resistance vessel functions via RyRs-BKCa down-regulation in rat offspring
Scientific Reports (2015)
-
Chronic hypoxia in pregnancy affected vascular tone of renal interlobar arteries in the offspring
Scientific Reports (2015)
-
Fetal programming by high-sucrose diet during pregnancy affects the vascular angiotensin II receptor–PKC–L-type Ca2+ channels (Cav1.2) axis to enhance pressor responses
Hypertension Research (2014)