ABSTRACT
Spontaneous Ca2+ oscillations in vascular smooth muscle cells have been modeled using a single Ca2+ pool. This report describes spontaneous Ca2+ oscillations dependent on two separate Ca2+ sources for the nuclear versus cytoplasmic compartments. Changes in free intracellular Ca2+ were monitored with ratiometric Ca2+- fluorophores using confocal microscopy. On average, spontaneous oscillations developed in 79% of rat aortic smooth muscle cells that were synchronous between the cytoplasm and nucleus. Reduction of extracellular Ca2+ (< 1 μM) decreased the frequency and amplitude of the cytoplasmic oscillations with 48% of the oscillations asynchronous between the nuclear and cytoplasmic compartments. Similar results were obtained with the Ca2+ channel blockers, nimodipine and diltiazem. Arg-vasopressin (AVP) induced a rapid release of intracellular Ca2+ stores that was greater in the nuclear compartment (4.20 ± 0.23 ratio units, n = 56) than cytoplasm (2.54 ± 0.28) in cells that had spontaneously developed prior oscillations. Conversely, cells in the same conditions lacking oscillations had a greater AVP-induced Ca2+ transient in the cytoplasm (4.99 ± 0.66, n = 17) than in the nucleus (2.67 ± 0.29). Pre-treatment with Ca2+ channel blockers depressed the AVP responses in both compartments with the cytoplasmic Ca2+ most diminished. Depletion of internal Ca2+ stores prior to AVP exposure blunted the nuclear response, mimicking the response of cells that lacked prior oscillations. Spontaneous oscillating cells had a greater sarcoplasmic reticulum network than cells that did not oscillate. We propose that spontaneous nuclear oscillations rely on perinuclear sarcoplasmic reticulum stores, while the cytoplasmic oscillations rely on Ca2+ influx.
Similar content being viewed by others
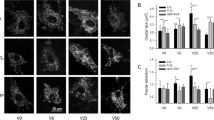
INTRODUCTION
In vascular smooth muscle, as in other types of muscle, an increase in intracellular Ca2+ is the immediate trigger for contraction, which ultimately determines vascular tone and peripheral resistance. In the past 12 years, investigators have characterized repetitive spontaneous Ca2+ changes in vascular smooth muscle cells, often referred to as spikes or oscillations, depending on their frequency 1, 2, 3. These transient elevations in intracellular Ca2+ correlate with oscillations in the electrical potential of the plasma membrane 3, 4, which are responsible for the rhythmic dilation and constriction noted in blood vessels 4. The oscillatory electrical activity is not dependent on neuronal input in vivo 4, and can be measured in cultured arterial smooth muscle cells 5. This spontaneous vasomotion is thought to assist in maintaining perfusion pressure and inhibiting tissue ischemia 6, 7.
Whole-cell techniques such as patch-clamp and fluorimetry have done much to characterize the spontaneous oscillations and ascribe a physiological function. Subcellular characterization of the oscillations has described the pattern of individual Ca2+ waves as they travel across the cell 8. Previous reports determined that the spontaneous oscillations in vascular smooth muscle cells were dependent on extracellular Ca2+ 3, 9, while increasing intracellular Ca2+ through release of internal stores initiated oscillations or increased their frequency 2, 9. Plasma membrane voltage-gated Ca2+ channels were shown to be responsible for the spontaneously oscillating free intracellular Ca2+ levels in cultured smooth muscle cells 2. Furthermore, the oscillations were shown to be highly variable even within the same cell 10.
Confluent monolayers of the rat aortic smooth muscle cell line, A7r5, exhibit a variety of structural and functional properties of freshly dispersed smooth muscle cells including active Ca2+ channels 11 and pumps 12, 13. In particular, these cells provide an excellent model for studying the spontaneous Ca2+ oscillations, because confluent cells electrically couple, and show evidence of spontaneous oscillations that are synchronized among cells 2, 3, 5, 14. This accounts for the ability of single-cell oscillations to produce small, repetitive, constrictive and dilatory vasomotion in vessels, which are in contrast to other oscillations in vessels that appear to be more random and do not propagate between cells 7. Under certain conditions 90% of all confluent cells have demonstrated spontaneous, repetitive Ca2+ spikes in the presence of 1.5 mM extracellular Ca2+ 3. These oscillations are different events from the Ca2+ sparks described in vascular smooth muscle that are localized within specific areas of cells with total durations of 100 ms 15. In contrast, spontaneous Ca2+ oscillations cover entire regions of cells and may have a duration of 30 s 14.
Ten years ago, we demonstrated significant non-uniformity in the distribution of Ca2+ in quiescent vascular smooth muscle cells using patch-clamp and microscopy techniques 16, 17. Since then, several reports have demonstrated that non-uniform Ca2+ distribution exists in smooth muscle cells in a variety of conditions 1, 18, 19. In the current report, we measured the subcellular patterns of spontaneous Ca2+ oscillations, using confocal microscopy and a variety of single and dual-wavelength Ca2+- sensitive fluorophores. While such spontaneous oscillations have been reported in smooth muscle cells previously, this report describes nuclear oscillations dependent on intracellular Ca2+ stores, strikingly different from previous reports, which showed little or no difference in the oscillation pattern following depletion of intracellular Ca2+ stores 9, 14. Data presented here illustrates a correlation between oscillations and subsequent agonist-induced Ca2+ responses. Finally, a model is proposed describing a possible mechanism for the oscillations and their effect on agonist-induced cell signaling.
MATERIALS AND METHODS
Cell culture
Rat thoracic aortic smooth muscle cells (A7r5, American Type Culture Collection, Manassas, VA) were grown in Dulbecco's modified Eagle's medium (DMEM), supplemented with 10% fetal bovine serum, 2 mM glutamine, 200 U/ml penicillin and 0.2 mg/ml streptomycin in 5% CO2 at 37°C. Cells were plated at a density of 104 cells/cm2 onto 25 mm glass cover slips in 35 mm dishes and used for experiments when the monolayers were near or at confluency (typically 2 d).
Calcium imaging with fluorescent and confocal microscopy
Dye loading and treatments
Cells were labeled with membrane-permeant, Ca2+-sensitive fluorophores by incubation in 5% CO2 at 37°C for 30 min in completed DMEM containing 1μM dye for all compounds. Experiments were replicated using more than one fluorophore to verify all results. Ratiometric Ca2+-sensitive indicators used in these experiments were fluo-4 and fura-red (Molecular Probes, Eugene, OR) 20, 21. While other indicators were tested (fluo-3, calcium orange, calcium green, calcium crimson, indo-1, and oregon Green; Molecular Probes), the ratiometric fluo-4/fura-red combination provided the most consistent results as fura-red fluorescence emission is quenched when Ca2+ increases, while fluo-4 fluorescence increases, thus artifacts due to movement or dye sequestration are avoided 31. Cells were incubated in 40 μM fura red and 20 μM fluo-4 in extracellular solution for 30 min at 37°C. Then cells were rinsed in extracellular solution consisting of (in mM): 137 NaCl, 2.7 KCl, 4.3 Na2HPO4 and 1.4 KH2PO4, pH 7.4. During experiments cells were bathed in the same solution with 2 mM Ca2+. Other conditions (noted in the body of the results section) consisted of one of the following: 1) 0 Ca2+ (1 mM EGTA); or 2) low Ca2+ (1 μM). The Arg8-vasopressin (AVP; Sigma, St. Louis, MO) concentration was 10 nM, because previous dose/response studies showed that maximal Ca2+ responses were obtained with AVP concentrations of 10-25 nM 22. The diltiazem (Sigma) concentration was 10 μM, nimodipine (Sigma) was 50 nM, and thapsigargin (Sigma) was 5 μM. Endoplasmic reticulum morphology was measured using ER Tracker Blue White DPX (Molecular Probes) using DAPI filters, which has been shown to be highly sensitive for ER 23. Cells were preloaded with Ca2+ Crimson and monitored for Ca2+ oscillations. Subsequently the chamber was washed and cells were incubated in ER Tracker for 15 min followed by another wash with extracellular solution prior to the capture of ER images. Great care was taken to ensure that the cells were exposed to the same conditions when loading and capturing images with ER Tracker, as the fluorophore is known to be highly sensitive to environmental conditions.
Microscopy and image analysis
Coverslips with cells were inserted into an Attofluor cell chamber (Molecular Probes) on the stage of an inverted Nikon Eclipse TE 300/Bio-Rad MicroRadiance Plus Laser Scanning Confocal Microscope (Bio-Rad Laboratories, Germany) or an Olympus FluoView 300 Confocal Microscope 24. Preliminary experiments were conducted on a Nikon Eclipse TE300 epi-fluorescent microscope (Nikon, Japan) attached to a SPOT32 camera (Diagnostic Instruments, Inc, Sterling Heights, MI) (results not shown). For imaging, Ca2+-sensitive fluorophores were excited at the recommended wavelengths (Molecular Probes). Sampling frequency varied depending on the experimental design, but was typically 1 scan every 1–10 s. The slow sampling frequency was chosen based on the slow frequency of the Ca2+ oscillations of interest, and the extended length of the experiments, often more than 20 min long.
Cells were analyzed for nuclear and cytoplasmic fluorescence using methods described by Goldman et al 25. Basically, the nucleus was identified easily by its spherical shape using simultaneously collected transmitted light images. Background fluorescence was subtracted from all fluorescence values. Due to the fact that these cells attached well to glass coverslips, several (10-15) cells could be optically dissected in a single field. Cells without clear nuclear compartments were excluded from data analysis. Fluorescence values from regions of interest within the cells were calculated as mean pixel values ± SE, and a ratio was calculated when using the Fluo-4/Fura Red combination. The delay of an agonist-induced response was measured from the time the agonist was added to the time when the ratio exceeded the baseline by 10%. This delay represented both the time required for the agonist to reach the cell surface, and the time required for the cell to respond. Similar results were obtained in at least three separate experiments for each condition. All experiments were performed at room temperature. Nested ANOVA was used to determine significant changes among different groups.
RESULTS
Spontaneous Ca2+ oscillations in the nucleus
The smooth muscle cell fluorescence emission was dynamic, even in unstimulated cells. Seventy-nine percent of all cells in physiological buffer with 2 mM Ca2+ demonstrated repetitive Ca2+ oscillations (n = 105). Oscillations were defined as repetitive fluctuations in fluorescence a minimum of 20% above the basal Ca2+ dependent fluorescent values. The presence of spontaneous oscillations in A7r5 cells was confirmed using different Ca2+-sensitive fluorophores, including Fluo-4, Fluo-3, Calcium Crimson, Calcium Orange, Calcium Green, Oregon Green, and ratiometric methods using Fluo-4 and Fura-Red 20, 21. The oscillations were synchronized among confluent cells. The field of cells illustrated in Fig. 1A shows the synchronized oscillations between different cells. Note that at least one cell did not follow the same pattern as the Ca2+ dropped between images while it was rising in the majority of other cells. The Ca2+-sensitive fluorescence emission was plotted in Fig. 1B. Each line represents the output obtained for a single cell, and is not normalized for different initial Ca2+concentrations. On average the spontaneous oscillations occurred with a frequency of one oscillation every 1-4 min. The frequency of spontaneous oscillations reported in the literature varies widely from 12/min 3 to 0.5-3/min 14, depending on the experimental conditions.
Spontaneous Ca 2+ oscillations in confluent vascular smooth muscle cells. (A) A7r5 cells were incubated in medium containing 2 mM Ca2+ and changes in Fluo-4 fluorescence were recorded within a single field. Most cells in the field showed spontaneous oscillations that were similar between cells. The three images were collected at 20 s intervals. The arrow (left panel) identifies a cell that showed decreasing fluorescence when Ca2+ appeared to be rising in the surrounding cells. (B) A graphic representation of the Ca2+ changes is shown over time. Each colored line indicates the whole-cell Ca2+ changes in an individual confluent cell.
The effects of extracellular Ca2+ on subcellular oscillations
To determine whether Ca2+ in the nuclear and cytoplasmic compartments of cells oscillated in identical manners, we analyzed Ca2+ activity in each subcompartment separately. Fig. 2A illustrates the time course and magnitude of the spontaneous oscillations from a single cell with synchronous cytoplasmic and nuclear Ca2+ oscillations. While amplitudes varied between the compartments, the general time course of the spontaneous oscillations was the same. Cells bathed in 2 mM Ca2+ developed spontaneous intracellular Ca2+ oscillations that were synchronous between the nuclear and cytoplasmic compartments.
Independent Ca2+ oscillations in the nuclear and cytoplasmic compartments. (A) Typical nuclear (black line) and cytoplasmic (gray line) Ca2+ oscillations within the same cell bathed in 2 mM Ca2+. Although the amplitude of the oscillations between the compartments varied, the overall oscillatory pattern was similar. (B) When extracellular Ca2+ was lowered, nearly half of the cells developed asynchronous oscillations between the nuclear and cytoplasmic compartments as demonstrated in the representative cell with a greater nuclear oscillation. There was 20 s between the two images. (C) The asynchronous oscillations are illustrated with the line graph. Ca2+ changes in the two compartments were measured using the Fluo-4/Fura Red ratio.
The percentage of cells with spontaneous oscillations dropped to 61% when extracellular Ca2+ was lowered to 1 μM (low Ca2+). The amplitudes of the cytoplasmic oscillations were dramatically diminished when extracellular Ca2+ was decreased or when Ca2+ influx was blocked by Ca2+ channel blockers. Nuclear oscillations were not statistically different in low or high extracellular Ca2+ conditions, although there was a trend towards lower peak levels in low extracellular Ca2+. Interestingly, there was a disconnection in the frequency of oscillations between the cytoplasm and nucleus in low extracellular Ca2+ conditions. Forty-eight percent of the spontaneous oscillations that occurred in low Ca2+ arose in the nucleus alone (n = 192 cells). Fig. 2B illustrates a cell with asynchronous oscillations which were larger in the nuclear versus the cytoplasmic compartment. While the spontaneous oscillations in the nucleus were larger, they were also slower in frequency, as illustrated in Fig. 2C (mean nuclear oscillations = 2.2 oscillations/min, 2.8 cytoplasmic oscillations/min). Further decreasing extracellular Ca2+ with the addition of 1 mM EGTA suppressed the spontaneous cytoplasmic oscillations, while nuclear oscillations continued in 33% of the cells. The lingering nuclear oscillations faded with time and at 8 min in 0 Ca2+ there were no nuclear or cytoplasmic oscillations.
Likewise the addition of the Ca2+ channel blocker, diltiazem, to the extracellular media halted cytoplasmic oscillations in 95% of the cells and decreased the frequency of the nuclear oscillations from 0.67 to 0.11 oscillations/min (not shown). Fig. 3 illustrates that a 5 min exposure to diltiazem reduced the peak amplitude of the spontaneous cytoplasmic oscillation from a 68 ± 6% to 12 ± 3 5% in fluorescence, while cells in low Ca2+ had oscillations that were 23 ± 4% above baseline (P < 0.01; n = 26/group). Nimodipine, another Ca2+ channel blocker, produced similar results (n = 20, not shown). The magnitude of the nuclear oscillations fell steadily with extended exposure to diltiazem. With 10 min of diltiazem or nimodipine application both cytoplasmic and nuclear oscillations were completely blocked.
Effects of extracellular Ca2+ on spontaneous oscillation activity. The amplitude of the spontaneous cytoplasmic oscillations was dramatically diminished by exposure to either a Ca2+channel blocker (diltiazem) or to low extracellular Ca2+ conditions (1 μM Ca2+; n = 26 cells/group). * indicates P < 0.01.
Depolarization of the cells in the presence of Ca2+ (100 mM K+, 2 mM Ca2+) induced a slow rise in cyto-plasmic, and a subsequent increase in nuclear fluorescence in 83% of all cells (n = 28). The time from depolarization to peak Ca2+ was 111 ± 12 s in the cytoplasm and 304 ± 47 s in the nucleus. Continued monitoring over the next 20 min revealed few subsequent nuclear or cytoplasmic oscillations. This result is in accord with previous reports that depolarization of the cells with high K+ (140 mM) activated a sustained rise in Ca2+ and abolished subsequent Ca2+ oscillatory activity 2.
The effects of intracellular Ca2+ stores on subcellular Ca2+ oscillations
To examine the effect of depletion of the intracellular Ca2+ store on spontaneous oscillations, cells were treated with thapsigargin, a selective inhibitor of the intracellular Ca2+-ATPase, which subsequently increased cytoplasmic Ca2+ in A7r5 cells 26. Application of thapsigargin (5 μM) induced a relatively rapid increase in intracellular Ca2+ (Fig. 4). Exposure to thapsigargin halted subsequent nuclear Ca2+ oscillations in 54% of the cells (n = 133 cells). Likewise, cytoplasmic oscillations were halted post thapsigargin in 62% of the cells tested. Most importantly, thapsigargin blocked any subsequent asynchronous oscillations between the nucleus and cytoplasm. Thus, 100% of the nuclear oscillations measured after thapsigargin exposure were synchronous with cytoplasmic oscillations as shown in Fig. 4. These oscillations had a mean frequency of 3.2 oscillations/min. When both intracellular Ca2+ release and extracellular Ca2+ influx were prevented by the combination of EGTA (1 mM) and thapsigargin (5 mM), there was an initial increase in fluorescence, which subsided by 1 min in both compartments. Subsequent Ca2+ elevations were completely abolished in both the cytoplasm and nucleus (n = 25).
Response of compartmental Ca2+ during SR Ca2+ release Stimulation of SR Ca2+ release with thapsigargin inhibited spontaneous oscillations in over half of all cells. In the remaining cells, all oscillations were synchronous between the nuclear and cytoplasmic compartments. The response of the two compartments from a typical cell is illustrated. Thapsigargin was added at time 0.
Spontaneous oscillations alter the Arg8-vasopressin stimulated-response
AVP is a potent InsP3-producing agonist for vascular smooth muscle cells, causing release of Ca2+ from intracellular stores followed by influx of Ca2+ from the extracellular environment 27. Exposure to AVP (10 nM) initiated a large transient in both the nuclear and cytoplasmic compartments in over 80% of all cells tested (n = 189). In general, cytoplasmic and nuclear Ca2+ increased at approximately the same rate during the initiation of the AVP response (Fig. 5A). The AVP-induced responses were significantly greater than spontaneous oscillations in either the nucleus or cytoplasm, as has been reported previously 3.
Influence of spontaneous Ca2+ oscillations on subsequent AVP response. (A) In a cell with spontaneous nuclear and cytoplasmic oscillations, the addition of AVP (at the arrow) induced a greater amplitude response in the nuclear compartment (black) than in the cytoplasm (gray). (B) In a cell lacking prior oscillations, the cytoplasmic AVP-induced transient tended to be larger than the nuclear. In both cases the nuclear compartment Ca2+ took longer to recover to baseline values.
As stated previously 79% of all cells in 2 mM Ca2+ had spontaneous oscillations. The remaining 21% failed to produce oscillations. These two populations of cells were analyzed as separate groups to decipher the characteristics of the oscillating cells. A clear difference was noted in the AVP response for cells that had prior oscillations from those that did not (Figs. 5A and 5B). The time from AVP exposure to the upstroke of the response (termed the delay) was not different in cells with or without prior oscillations (n = 50). The magnitude of the responses was significantly different, depending on whether the cells had prior spontaneous oscillations. The amplitude of the peak AVP response was greater in the nuclear compartment (4.20 ± 0.23, n = 51) than cytoplasmic (2.54 ± 0.28, p < 0.0002) in cells that had spontaneously developed prior oscillations (Fig. 6). Conversely, cells in the same environment that failed to produce oscillations had a greater AVP-induced Ca2+ transient in the cytoplasm (amplitude of transient = 4.99 ± 0.66, n = 50) than in the nucleus (2.67 ± 0.29, p < 0.0005). The duration of the AVP transient was longer in the nucleus than cytoplasm in both cell types (mean = 17 s. longer in nucleus; Figs. 5A and 5B). Cells without oscillations prior to the AVP exposure, often developed oscillations subsequent to the application of AVP (not shown), illustrating that the cells were in fact capable of oscillating when prompted.
Characterization of the AVP response in cellular subcompartments. Cells with spontaneous oscillations had greater AVP responses in the nuclear compartment than in the cytoplasm (n = 51, p < 0.0002). The reverse is true for cells that lacked spontaneous Ca2+ oscillations (n = 50, p < 0.0005). Exposure of cells to a plasma membrane Ca2+ channel blocker (diltiazem) reduced the amplitude of the cytoplasmic compartment Ca2+ response, but there was no statistical difference between the nuclear and cytoplasmic response. Pre-treatment of cells with thapsigargin resulted in an AVP response with a greater cytoplasmic than nuclear transient (n = 21, p < 0.005). * indicates statistical difference between nuclear and cytoplasmic compartments for each group. # indicates the difference in the nuclear and cytoplasmic Ca2+ peaks between cells with and without spontaneous oscillations (p < 0.001).
The addition of AVP in the presence of the Ca2+ channel blocker diltiazem prevented the sustained cytoplasmic oscillations normally seen after AVP exposure. There was no statistical difference in the AVP transient between the nuclear compartment (3.33 ± 0.26, n = 18) and the cytoplasmic compartment (1.96 ± 0.36) following pre-treatment with diltiazem (Fig. 6). Pre-treatment with thapsigargin (5 min) depleted the intracellular Ca2+ stores and decreased the subsequent AVP-induced nuclear Ca2+ transient (Fig. 6). The peak AVP-induced nuclear transient was reduced in the presence of thapsigargin (2.21 ± 0.33, n = 21), while the cytoplasmic transient was not affected by the pre-treatment with thapsigargin (3.79 ± 0.37, p < 0.005). The duration of the AVP responses in both the nuclear and cytoplasmic compartments was significantly lengthened by exposure to thapsigargin. In the presence of thapsigargin, post-AVP oscillations appeared in the cytoplasm that were absent in nearly all of the nuclei.
Nuclear Ca2+ oscillations and sarcoplasmic reticulum morphology
Cells that demonstrated spontaneous cytoplasmic Ca2+ oscillations (measured with Calcium Crimson) were subsequently labeled with the fluorescent SR-specific fluorophore, ER Tracker (Fig. 7A). The presence of cellular Ca2+ oscillations was correlated with the level and characteristics of the SR (n = 28 cells). Cellular SR-derived fluorescence was significantly greater in the cells that had demonstrated spontaneous Ca2+ oscillations compared to cells in the same field that failed to elicit spontaneous oscillations (Fig. 7B). Interestingly, there was no difference in the perinuclear SR in groups of cells with or without spontaneous cytoplasmic Ca2+ oscillations (Fig. 7C).
Correlation of SR morphology and spontaneous Ca2+ oscillations. (A) Following the monitoring of subcellular oscillations with the Ca2+ fluorophore, Ca 2+ Crimson, cells were tagged as either spontaneous oscillators or cells lacking oscillations. All cells were exposed to the SR-specific fluorescent probe, ER Tracker, which resulted in illustrations of both the distribution and amount of SR in each cell. The field of cells on the left did not demonstrate spontaneous oscillations, while the cells on the right were oscillatory. (B) Analysis of the levels of SR in the cells demonstrated that cells with oscillations had significantly more SR fluorescence (p< 0.01). (C) In contrast, analysis of the levels of perinuclear SR demonstrated no difference between the two groups.
DISCUSSION
Spontaneous oscillatory behavior has been noted previously in vascular smooth muscle cells, first as electrical oscillations 28, 29. Later, spontaneous Ca2+ oscillations were measured in smooth muscle cells with extensive structural and electrical contact 2, 3, 5, 9, 14. These investigators established the need for extracellular Ca2+, since its removal 3, or the addition of nimodipine 14, a common L-type channel blocker, abolished the spontaneous Ca2+ oscillations. Prolonged incubation with thapsigargin, which depletes intracellular Ca2+ stores, did not block subsequent spikes in previously published reports 14. Further, caffeine, a ryanodine receptor agonist, did not suppress the oscillations 9. All these results indicated that Ca2+ entry through L-type Ca2+ channels was a requirement for spontaneous oscillations, and that mobilization of intracellular Ca2+ stores was not necessary 5, 14. However, the initial electrical event at the plasma membrane that launches the oscillation is still unknown 7. In contrast, the data presented here identified Ca2+ oscillations within nuclei of smooth muscle cells that were dependent on intracellular Ca2+ stores. Furthermore, reduction of extracellular Ca2+ blocked cytoplasmic oscillations, while nuclear oscillations were maintained in nearly half of all cells. We categorized these cells as having asynchronous Ca2+ oscillations. Such a term may be confusing, as asynchrony commonly represents the unique Ca2+ behavior between cells 30 but not within cells.
The Ca2+ oscillations identified in this report are qualitatively different from the common Ca2+ oscillations measured in vascular smooth muscle cells, as these oscillations have a much lower frequency with an average of one oscillation every 0.5-4 min. In asynchronous conditions, the nuclear oscillations occurred more frequently than those in the cytoplasm. The frequency of spontaneous oscillations reported in the literature varies widely with most reports focusing on the higher frequency oscillations 28. The low frequency oscillations have been reported previously by other groups 14, but are less well-studied, which may explain why the phenomenon of asynchronous oscillations had not been uncovered previously.
Smooth muscle cells certainly possess mechanisms for passive diffusion of Ca2+ across the nuclear envelope, and many signaling cascades use the passive flow of ions and small molecules across the envelope to influence nuclear events 31. However, there is a growing appreciation for the role of the nuclear envelope and perinuclear SR in regulating nuclear Ca2+ levels independent of cytoplasmic concentrations 32, 33, 34, 35. In fact, AVP has already been reported to increase Ca2+ in the nuclear and cytoplasmic regions of rat aortic smooth muscle cells with the suggestion that the perinuclear region was likely an important Ca2+ storage site 36. The source responsible for the independent spontaneous nuclear oscillations appears to be the perinuclear SR, which acts as a Ca2+ sink, initiating nuclear and perinuclear transients. In addition, the nuclear envelope itself may be involved in Ca2+ regulation of the nucleus as it acts as a Ca2+ storage site 37, 38, 39. Multiple publications have confirmed extensive Ca2+ signaling into the nucleus. Functional endothelin-1 receptors have been located on the nuclear membrane in vascular smooth muscle cells to increase nuclear Ca2+ when stimulated 40. Furthermore, exposure to extracellular low-density lipoproteins induced a rise in Ca2+ that was localized to the nuclear region 41. Just as researchers have ascribed unique contributions by the superficial SR, the perinuclear SR and nuclear envelope could be modeled as deep SR with its own unique functions 7.
The data presented here documented the presence of oscillations in the nucleus without concurrent oscillations in the cytoplasm and vice versa. The study of subcellular Ca2+ events during spontaneous oscillations is an important topic with few, if any, previous publications 7. It provides compelling evidence that, under certain conditions, nuclear Ca2+ is independently regulated. We propose a model in which spontaneous Ca2+ oscillations within the cytoplasm are dependent on extracellular Ca2+ influx and the plasma membrane Ca2+ pumps. This is in agreement with earlier reports indicating that Ca2+ influx is needed for spontaneous smooth muscle cell oscillations 2, 3, 5, 9, 14, 42 . However, these reports monitored oscillations by means of electrophysiology or Ca2+ fluorescence without analysis of separate intracellular compartments. Our data showing independent nuclear oscillations in low extracellular Ca2+ suggest a two-pool model for Ca2+ oscillations for the cytoplasmic versus nuclear compartments. We propose that nuclear Ca2+ oscillations are governed principally by Ca2+ stores in the nuclear envelope and in the perinuclear SR. In this model it is possible, and quite likely, to have asynchronous nuclear and cytoplasmic oscillations. A cytoplasmic oscillation might not immediately diffuse into the nucleus through the nuclear pore complexes, because an extensive network of perinuclear SR or Ca2+ binding proteins would buffer the Ca2+ before it could near the pore. Freshly dispersed vascular smooth muscle cells have a transnuclear SR that pierces the nucleoplasm, described by Hill et al 43 and observed in our laboratory (Y Searls and L Stehno-Bittel, unpublished observations), which would act as strong nuclear Ca2+ buffers.
We hypothesize that cells with more extensive SR would be more capable of sustaining spontaneous nuclear Ca2+ oscillations. In experiments described here attempting to statistically correlate the amount of SR and the frequency of oscillations we had mixed results. We were able to statistically link spontaneous cellular oscillations with an extended cellular SR network, yet there were no differences in the perinuclear SR. We are not the first to suggest a relationship between the intracellular Ca2+ buffer capacity and the presence of spontaneous Ca2+ oscillations. Wu et al suggested such a correlation based on power spectrum analysis of oscillations 9. They compared experimental data to a one pool non-oscillatory model of Ca2+ regenerative oscillations measured after AVP exposure, and proposed that when spontaneous oscillations occurred, the refilling of the internal Ca2+ stores was facilitated by the periodic change in membrane potential 9.
The mechanism responsible for the diversity of cells containing or lacking spontaneous oscillations is unknown, but could occur through changes in Ca2+ release channels or Ca2+ pumps. Both InsP3 receptors and SERCA pumps have been identified previously on the nuclear envelope or in the perinuclear region of A7r5 cells 39. We have evidence that the distribution of the SERCA2 isoform of the Ca2+ pump changes from a general SR pattern to a perinuclear distribution when cells are grown in high glucose. This appears to occur without a change in the ultrastructure of the SR (Y Searls and L Stehno-Bittel, unpublished observations). In the experiments described here, all cells were grown in identical conditions, yet only a certain population of cells developed spontaneous oscillations. Perhaps those cells contained a greater intracellular Ca2+ store that could participate and even initiate the spontaneous oscillations. Additionally, it is known that AVP-induced Ca2+ oscillations are dependent on the activation of protein kinase C (PKC) 44, thus Ca2+ oscillations and the changes in AVP-stimulated Ca2+ transients may be due to different activity levels of PKC. Whatever the exact mechanism is, two separate sources of Ca2+ appear to be responsible for the subcellular Ca2+ oscillations. The nuclear oscillations are dependent on a perinuclear source and cytoplasmic oscillations require the influx of Ca2+ from outside the cell.
Evidence for this model is provided by the following observations: 1) the magnitude of the nuclear AVP-induced peak was approximately 50% less in cells without oscillations; 2) blockers of plasma membrane Ca2+ channels attenuated the cytoplasmic oscillations first, followed by a slow attenuation of the nuclear oscillations; 3) depletion of the internal Ca2+ store halted asynchronous nuclear oscillations; 4) more extensive SR was associated with cells that exhibited spontaneous oscillations. The model refines a previously published model for Ca2+ oscillations in tracheal smooth muscle cells in which the oscillation amplitude was proposed to be dependent on the amount of Ca2+ in the SR, while the oscillation frequency and propagation velocity reflected the active state of the ryanodine receptors 45.
Ca2+ oscillations are a nearly universal mode of signaling in excitable and non-excitable cells 46. Local frequency and amplitude modulation may allow for rapid adjustments in the signaling process, not available to whole-cell changes in Ca2+ 1. Localized changes in Ca2+ regulation and release may play an important role in normal signaling and in disease states of the vascular system 47. It was suggested that a lack of nuclear Ca2+ buffering is a feature of pathological changes in vascular smooth muscle cells 43. We are currently undertaking experiments to determine whether nuclear Ca2+ buffering is altered in advanced diabetes-induced vascular conditions. Identifying the mechanisms involved may lead to new approaches to pharmacological interventions for vascular disease.
References
Pabelick CM, Sieck GC, Prakash YS . Invited review: significance of spatial and temporal heterogeneity of calcium transients in smooth muscle. J Appl Physiol 2001; 91(1):488–96.
Otun H, Gillespie JI, Nicholls JA, Greenwell JR, Dunlop W . Transients in intracellular free calcium in subconfluent and confluent cultures of a rat smooth muscle cell line. Exp Physiol 1992; 77(5):749–56.
Missiaen L, Oike M, Bootman MD, et al. Vasopressin responses in electrically coupled A7r5 cells. Pflugers Arch 1994; 428(3–4):283–7.
Bartlett IS, Crane GJ, Neild TO, Segal SS . Electrophysiological basis of arteriolar vasomotion in vivo. J Vasc Res 2000; 37(6):568–75.
Marks TN, Dubyak GR, Jones SW . Calcium currents in the A7r5 smooth muscle-derived cell line. Pflugers Arch 1990; 417(4):433–9.
Intaglietta M . Arteriolar vasomotion: implications for tissue ischemia. Blood Vessels 1991; 28(Suppl 1): 1–7.
Lee CH, Poburko D, Kuo KH, Seow CY, van Breemen C . Ca2+ oscillations, gradients, and homeostasis in vascular smooth muscle. Am J Physiol Heart Circ Physiol 2002; 282(5):H1571–83.
Neylon CB, Hoyland J, Mason WT, Irvine RF . Spatial dynamics of intracellular calcium in agonist-stimulated vascular smooth muscle cells. Am J Physiol 1990; 259(4 Pt 1):C675–86.
Wu SN, Yu HS, Seyama Y . Analytical studies of spontaneous and vasopressin-induced calcium oscillations in cultured vascular smooth muscle cells. J Biochem (Tokyo) 1996; 119(1):42–8.
Mahoney MG, Slakey LL, Hepler PK, Gross DJ . Independent modes of propagation of calcium waves in smooth muscle cells. J Cell Sci 1993; 104(Pt 4):1101–7.
Parys JB, Missiaen L, De Smedt H, Droogmans G, Casteels R . Bell-shaped activation of inositol-1,4,5-trisphosphate-induced Ca2+ release by thimerosal in permeabilized A7r5 smooth-muscle cells. Pflugers Arch 1993; 424(5–6):516–22.
Roullet JB, Le Quan Sang KH, Luft U, et al. Inhibition of Ca2+ uptake into A7r5 vascular smooth muscle cells by farnesol: lack of effect on membrane fluidity and Ca2+-ATPase activities. J Hypertens 1997; 15(12 Pt 2):1723–8.
Wu KD, Bungard D, Lytton J . Regulation of SERCA Ca2+ pump expression by cytoplasmic Ca2+ in vascular smooth muscle cells. Am J Physiol Cell Physiol 2001; 280(4):C843–51.
Byron KL, Taylor CW . Spontaneous Ca2+ spiking in a vascular smooth muscle cell line is independent of the release of intracellular Ca2+ stores. J Biol Chem 1993; 268(10):6945–52.
White C, McGeown JG . Inositol 1,4,5-trisphosphate receptors modulate Ca2+ sparks and Ca2+ store content in vas deferens myocytes. Am J Physiol Cell Physiol 2003; 285(1):C195–204.
Stehno-Bittel L, Sturek M . Spontaneous sarcoplasmic reticulum calcium release and extrusion from bovine, not porcine, coronary artery smooth muscle. J Physiol (Lond) 1992; 451: 49–78.
Stehno-Bittel L, Laughlin MH, Sturek M . Exercise training depletes sarcoplasmic reticulum calcium in coronary smooth muscle. J Appl Physiol 1991; 71(5):1764–73.
Marsault R, Murgia M, Pozzan T, Rizzuto R . Domains of high Ca2+ beneath the plasma membrane of living A7r5 cells. Embo J 1997; 16(7):1575–81.
Zhang X, Wen Y, Xu X, Dong F, Ren J . Agonist-stimulated Ca2+ transport in mesenteric vascular smooth muscle cells of vitamin D(3)-induced calcium overload rats. Vascul Pharmacol 2003; 40(4):189–95.
Marie I, Beny JL . Calcium imaging of murine thoracic aorta endothelium by confocal microscopy reveals inhomogeneous distribution of endothelial cells responding to vasodilator agents. J Vasc Res 2002; 39(3):260–7.
Oishi H, Budel S, Schuster A, Stergiopulos N, Meister JJ, Beny JL . Cytosolic-free calcium in smooth-muscle and endothelial cells in an intact arterial wall from rat mesenteric artery in vitro. Cell Calcium 2001; 30(4):261–7.
Blatter LA, Wier WG . Agonist-induced [Ca2+]i waves and Ca2+-induced Ca2+ release in mammalian vascular smooth muscle cells. Am J Physiol 1992; 263(2 Pt 2):H576–86.
Cole L, Davies D, Hyde GJ, Ashford AE . ER-Tracker dye and BODIPY-brefeldin A differentiate the endoplasmic reticulum and golgi bodies from the tubular-vacuole system in living hyphae of Pisolithus tinctorius. J Microsc 2000; 197(Pt 3):239–49.
Bkaily G, Pothier P, D'Orleans-Juste P, et al. The use of confocal microscopy in the investigation of cell structure and function in the heart, vascular endothelium and smooth muscle cells. Mol Cell Biochem 1997; 172(1–2):171–94.
Goldman WF . Spatial and temporal resolution of serotonin-induced changes in intracellular calcium in a cultured arterial smooth muscle cell line. Blood Vessels 1991; 28(1–3):252–61.
Doyle VM, Ruegg UT . Vasopressin induced production of inositol trisphosphate and calcium efflux in a smooth muscle cell line. Biochem Biophys Res Commun 1985; 131(1):469–76.
Kaplan-Albuquerque N, Di Salvo J . Protein kinase C: modulation of vasopressin-induced calcium influx and release in A7r5 vascular smooth muscle cells. Arch Biochem Biophys 1998; 359(2):209–14.
Kimes BW, Brandt BL . Characterization of two putative smooth muscle cell lines from rat thoracic aorta. Exp Cell Res 1976; 98(2):349–66.
Van Renterghem C, Romey G, Lazdunski M . Vasopressin modulates the spontaneous electrical activity in aortic cells (line A7r5) by acting on three different types of ionic channels. Proc Natl Acad Sci U S A 1988; 85(23):9365–9.
Iino M, Kasai H, Yamazawa T . Visualization of neural control of intracellular Ca2+ concentration in single vascular smooth muscle cells in situ. Embo J 1994; 13(21):5026–31.
Haller H, Lindschau C, Quass P, Distler A, Luft FC . Nuclear calcium signaling is initiated by cytosolic calcium surges in vascular smooth muscle cells. Kidney Int 1994; 46(6):1653–62.
al-Mohanna FA, Caddy KW, Bolsover SR . The nucleus is insulated from large cytosolic calcium ion changes. Nature 1994; 367(6465):745–50.
Himpens B, De Smedt H, Casteels R . Subcellular Ca2+-gradients in A7r5 vascular smooth muscle. Cell Calcium 1994; 15(1):55–65.
Badminton MN, Kendall JM, Rembold CM, Campbell AK . Current evidence suggests independent regulation of nuclear calcium. Cell Calcium 1998; 23(2–3):79–86.
Stehno-Bittel L, Luckhoff A, Clapham DE . Calcium release from the nucleus by InsP3 receptor channels. Neuron 1995; 14(1):163–7.
Fujihara H, Fukuda S, Tanaka T, Kanazawa H, Fujiwara N, Shimoji K . Arginine vasopressin increases perinuclear [Ca2+] in single cultured vascular smooth muscle cells of rat aorta. J Vasc Res 1993; 30(4):231–8.
Stehno-Bittel L, Perez-Terzic C, Luckhoff A, Clapham DE . Nuclear ion channels and regulation of the nuclear pore. Soc Gen Physiol Ser 1996; 51: 195–207.
Gerasimenko OV, Gerasimenko JV, Tepikin AV, Petersen OH . ATP-dependent accumulation and inositol trisphosphate- or cyclic ADP-ribose-mediated release of Ca2+ from the nuclear envelope. Cell 1995; 80(3):439–44.
Humbert JP, Matter N, Artault JC, Koppler P, Malviya AN . Inositol 1,4,5-trisphosphate receptor is located to the inner nuclear membrane vindicating regulation of nuclear calcium signaling by inositol 1,4,5-trisphosphate. Discrete distribution of inositol phosphate receptors to inner and outer nuclear membranes. J Biol Chem 1996; 271(1):478–85.
Bkaily G, Choufani S, Hassan G, et al. Presence of functional endothelin-1 receptors in nuclear membranes of human aortic vascular smooth muscle cells. J Cardiovasc Pharmacol 2000; 36(5 Suppl 1): S414–7.
Massaeli H, Hurtado C, Austria JA, Pierce GN . Increase in nuclear calcium in smooth muscle cells exposed to oxidized low density lipoprotein. Free Radic Res 2001; 34(1):9–16.
Byron KL . Vasopressin stimulates Ca2+ spiking activity in A7r5 vascular smooth muscle cells via activation of phospholipase A2. Circ Res 1996; 78(5):813–20.
Hill BJ, Wamhoff BR, Sturek M . Functional nucleotide receptor expression and sarcoplasmic reticulum morphology in dedifferentiated porcine coronary smooth muscle cells. J Vasc Res 2001; 38(5):432–43.
Fan J, Byron KL . Ca2+ signalling in rat vascular smooth muscle cells: a role for protein kinase C at physiological vasoconstrictor concentrations of vasopressin. J Physiol (Lond) 2000; 524(Pt 3):821–31.
Prakash YS, Pabelick CM, Kannan MS, Sieck GC . Spatial and temporal aspects of ACh-induced [Ca2+]i oscillations in porcine tracheal smooth muscle. Cell Calcium 2000; 27(3):153–62.
Berridge MJ, Lipp P, Bootman MD . The versatility and universality of calcium signalling. Nat Rev Mol Cell Biol 2000; 1(1):11–21.
Missiaen L, Robberecht W, van den Bosch L, et al. Abnormal intracellular ca2+ homeostasis and disease. Cell Calcium 2000; 28(1):1–21.
Acknowledgements
We greatly appreciate the work of Mrs. Eileen Roach for assistance with data collection. This work was supported by NIH RO1 GMS and US Department of Commerce SABIT grants to Lisa Stehno-Bittel.
Author information
Authors and Affiliations
Corresponding author
Rights and permissions
About this article
Cite this article
FEDORYAK, O., SEARLS, Y., SMIRNOVA, I. et al. Spontaneous Ca2+ oscillations in subcellular compartments of vascular smooth muscle cells rely on different Ca2+ pools. Cell Res 14, 379–388 (2004). https://doi.org/10.1038/sj.cr.7290238
Received:
Revised:
Accepted:
Issue Date:
DOI: https://doi.org/10.1038/sj.cr.7290238
Keywords
This article is cited by
-
Nuclear pores enable sustained perinuclear calcium oscillations
BMC Systems Biology (2016)
-
Intracellular Ca2+ regulating proteins in vascular smooth muscle cells are altered with type 1 diabetes due to the direct effects of hyperglycemia
Cardiovascular Diabetology (2010)