Abstract
Geologic carbon sequestration is the capture of anthropogenic carbon dioxide (CO2) and its storage in deep geologic formations. The processes of CO2 seepage into surface water after migration through water-saturated sediments are reviewed. Natural CO2 and CH4 fluxes are pervasive in surface-water environments and are good analogues to potential leakage and seepage of CO2. Buoyancy-driven bubble rise in surface water reaches a maximum velocity of approximately 30 cm s−1. CO2 rise in saturated porous media tends to occur as channel flow rather than bubble flow. A comparison of ebullition versus dispersive gas transport for CO2 and CH4 shows that bubble flow will dominate over dispersion in surface water. Gaseous CO2 solubility in variable-salinity waters decreases as pressure decreases leading to greater likelihood of ebullition and bubble flow in surface water as CO2 migrates upward.






Similar content being viewed by others
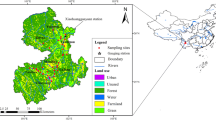
Abbreviations
- A :
-
Empirical fit parameter
- A d :
-
Additional mass
- C M :
-
Mass factor
- C :
-
Concentration (mole fraction)
- d :
-
Radius in Stoke's Law (m)
- d p :
-
Porous media particle diameter (m)
- D :
-
Diffusivity and dispersivity (m2 s−1)
- E :
-
Total ebullition rate (mol cm−2 s−1)
- F b :
-
Buoyancy force (N)
- F D :
-
Diffusive flux (mol cm−2 s−1)
- F E :
-
Bubble (ebullition) flux (mol cm−2 s−1)
- F d :
-
Drag force (N)
- F E :
-
Fraction ebullition flux
- g :
-
Gravitational acceleration (m s−2)
- H :
-
Henry’s Law coefficient (Pa)
- K :
-
Henry’s Law coefficient (mol cm−3 atm−1)
- k :
-
Permeability (m2)
- m :
-
Mole flux (mol cm−2 s−1)
- n :
-
Porosity
- N i :
-
Molar content of gas species i (mol)
- P :
-
Pressure (Pa, atm)
- \(P_{{\rm CO_{2}}}\) :
-
Partial pressure of CO2 (Pa, atm)
- P st :
-
Surface tension pressure (N m−2)
- P z :
-
Hydrostatic pressure (Pa, atm)
- \(q_{{\rm B}_{i}}\) :
-
Bubble gas transfer rate of species i (mol cm−2s−1)
- r :
-
Bubble radius (m)
- r p :
-
Characteristic length scale of pore (m)
- R′:
-
Equivalent pore throat radius (m)
- R b :
-
Bubble radius (m)
- T :
-
Temperature (°C)
- u b :
-
Bubble velocity (m s−1)
- v:
-
Stoke's velocity (m s−1)
- V B :
-
Bubble velocity in surface water (m s−1)
- z :
-
Vertical coordinate (m)
- μ w :
-
Water viscosity (kg m−1 s−1)
- ρ g :
-
Gas-phase density (kg m−3)
- ρ f :
-
Fluid density (kg m−3)
- ρw :
-
Water density (kg m−3)
- σ:
-
Surface tension (N m−1)
- θ:
-
Contact angle (degrees)
- [ ]:
-
Concentration (mol cm−3)
References
Anderson AL, Bryant WR (1990) Gassy sediment occurrence and properties: northern Gulf of Mexico. Geo-Marine Lett 10:209–220
Baldocchi DD, Wilson KB (2001) Modeling CO2 and water vapor exchange of a temperate broadleaved forest across hourly to decadal time scales. Ecol Modell 142:155–184
Boles JR, Clark JF, Leifer I, Washburn L (2001) Temporal variation in natural methane seep rate due to tides, Coal Oil Point area, California. J Geophys Res 106:27077–27086
Brooks MC, Zise WR, Annable MD (1999) Fundamental changes in in-situ airsparging flow patterns. Ground Water Monit Rem 19(2):105–113
Casper P, Maberly SC, Hall GH, Finlay BJ (2000) Fluxes of methane and carbon dioxide from a small productive lake to the atmosphere. Biogeochemistry 49:1–19
Chiodini G, Frondini F, Kerrick DM, Rogie J, Parello F, Peruzzi L, Zanzari AR (1999) Quantification of deep CO2 fluxes from Central Italy. Examples of carbon balance for regional aquifers and of soil diffuse degassing. Chem Geol 159:205–222
Chiodini G, Frondini F, Cardellini C, Parello F, Peruzzi L (2000) Rate of diffuse carbon dioxide Earth degassing estimated from carbon balance of regional aquifers: The case of the central Apennine, Italy. J Geophys Res 105:8423–8424
Christensen TR, Panikov N, Mastepanov M, Joabsson A, Stewart A, Oquist M, Sommerkorn M, Reynaud S, Svensson B (2003) Biotic controls on CO2 and CH4 exchange in wetlands–a closed environment study. Biogeochem 64:337–354
Corapcioglu MY, Cihan A, Drazenovic M (2004) Rise velocity of an air bubble in porous media: Theoretical studies. Water Resour Res 40:W04214. DOI 10.1029/2003WR002618
Ennis-King J, Paterson L (2003) Rate of dissolution due to convective mixing in the underground storage of carbon dioxide. In: Gale J, Kaya Y (eds) Proceedings of the 6th international conference on greenhouse gas control technologies, vol 1. pp 507–510
Evans WC, Sorey ML, Cook AC, Kennedy BM, Shuster DL, Colvard EM, White LD, Huebner MA. (2002) Tracing and quantifying magmatic carbon discharge in cold groundwaters: lessons learned from Mammoth Mountain, USA. J Volcanol Geotherm Res 114:291–312
Fischer HB, List EJ, Imberger J, Brooks NH (1979) Mixing in Inland and Coastal Waters. Academic Press, New York
Giggenbach WF (1990) Water and gas chemistry of Lake Nyos and its bearing on the eruptive process. J Volcanol Geotherm Res 42:337–362
Giggenbach WF, Sano Y, Schmincke HU (1991) CO2-rich gases from Lakes Nyos and Monoun, Cameroon; Laacher See, Germany, Dieng, Indonesia, and Mt. Gambier, Australia - variations on a common theme. J Volcanol Geotherm Res 45:311–323
Goldman CR, Horne AJ (1983) Limnology. McGraw-Hill, New York
Halbwachs M, Sabroux J-C, Grangeon J, Kayser G, Tochon-Danguy J-C, Felix A, Beard J-C, Veilleveille A, Vitter G, Rishon P, Wuest A, Hell J (2004) Degassing the “killer lakes” Nyos and Monoun, Cameroon. Trans Am Geophys Union EOS 85(3):281–285
Harriss RC, Sebacher D (1981) Methane flux in forested freshwater swamps of the southeastern United States. Geophys Res Lett 8:1002–1004
Hornafius SJ, Quigley D, Luyendyk BP (1999) The world’s most spectacular marine hydrocarbon seeps (Coal Oil Point, Santa Barbara Channel, California): Quantification of emissions. J Geophys Res 104:20703–20711
Hovland M, Judd AG (1988) Seabed pockmarks and seepages: impact on geology, biology, and the marine environment. Graham & Trotman, London
Hovland M, Judd AG, Burke RA Jr (1993) The global flux of methane from shallow marine sediments. Chemosphere 26:559–578
Hovorka SD, Doughty C, Benson SM, Pruess K, Knox PR (2004) Assessment of the impact of geological heterogeneity on CO2 storage in brine formations: a case study from the Texas Gulf Coast. In: Baines SJ, Gale J, Worden RH (eds) Geological storage of carbon dioxide for emissions reduction: technology, vol 233. Geological Society of London Special Publication, pp 147–163
Iliuta I, Ortiz-Arroyo A, Larachi F, Grandjean BPA, Wild G (1999) Hydrodynamics and mass transfer in trickle-bed reactors: an overview. Chem Eng Sci 54(21):5329–5337
Ji W, Dahmani A, Ahlfied DP, Lin JD, Hill E III (1993) Laboratory study of air sparging: Air flow visualization, Ground Water Monit Rem 13(4):115–126
Joyce J, Jewell PW (2003) Physical controls on methane ebullition from reservoirs and lakes. Env Eng Geosc 9:167–178
Keller M, Stallard RF (1994) Methane emission by bubbling from Gatun Lake, Panama. J Geophys Res 99:8307–8319
Leifer I, Patro RK (2002) The bubble mechanism for methane transport from the shallow sea bed to the surface: A review and sensitivity study. Continental Shelf Res 22:2409–2428
Leifer I, Clark JF, Chen RF (2000) Modifications of the local environment by natural marine hydrocarbon seeps. Geophys Res Lett 27:3711–3714
Lemmon EW, McLinden MO, Friend DG (2003) Thermophysical properties of fluid systems. In: Linstrom PJ, Mallard WG (eds) NIST Chemistry WebBook, NIST Standard Reference Database Number 69, National Institute of Standards and Technology, Gaithersburg, Maryland (http://www.webbook.nist.gov)
Lewicki JL, Hilley GE, Oldenburg CM (2005) An improved strategy to detect CO2 leakage for verification of geologic carbon sequestration. Geophys Res Lett. 32(19):L19403. DOI 10.1029/2005GL024281
Macdonald JA, Fowler D, Hargreaves KJ, Skiba U, Leith ID, Murray MB (1998) Methane emission rates from a northern wetland—response to temperature, water table and transport. Atmos Environ 32:3219–3227
de Marsily G (1986) Quantitative hydrogeology. Academic Press, New York
Martens CS, Klump JV (1980) Biogeochemical cycling in an organic rich coastal marine basin. 1. Methane sediment-water exchange processes. Geochim Cosmochim Acta 44:471–490
Morel FMM, Hering JG (1993) Principles and applications of aquatic geochemistry. Wiley, New York
Oldenburg CM, Unger AJA (2003) On leakage and seepage from geologic carbon sequestration sites: unsaturated zone attenuation. Vadose Zone J 2:287–296
Oldenburg CM, Unger AJA (2004) Coupled vadose zone and atmospheric surface-layer transport of CO2 from geologic carbon sequestration sites. Vadose Zone Journal 3:848–857
Oldenburg CM, Lewicki JL, Hepple RP (2003) Near-surface monitoring strategies for geologic carbon dioxide storage verification. Lawrence Berkeley National Laboratory Report LBNL-54089
Oskarsson N (1990) Carbon dioxide bursts of lake Nyos, Cameroon, modeled as a periodic supersaturation in a countercurrent reactor. J Volcanol Geotherm Res 42:307–318
Pellicer, J, Garcia-Morales V, Hernandez MJ (2000) On the demonstration of the Young–Laplace equation in introductory physics courses. Phys Educ 35(2):126–129
Roosevelt SE, Corapcioglu MY (1998) Air bubble migration in a granular porous medium: Experimental studies. Water Resour Res 34(5):1131–1142
Rosenberry DO, Glaser PH, Siegle DI, Weeks EP (2003) Use of hydraulic head to estimate volumetric gas content and ebullition flux in northern peatlands. Water Resour Res 39:1066. DOI 10.1029/2002WR001377
Shipton ZK, Evans JP, Dockrill B, Heath J, Williams A, Kirschner D, Kolesar PT (2004a) Natural leaking CO2-charged systems as analogs for failed geologic sequestration reservoirs. In: Thomas D, Benson SM (eds) Carbon dioxide capture for storage in deep geologic formations—results from the CO2 capture project, vol 2. Geologic storage of carbon dioxide with monitoring and verification, Elsevier, Amsterdam
Shipton ZK, Evans JP, Kirschner D, Kolesar PT, Williams AP, Heath J (2004b) Analysis of CO2 leakage through “low-permeability” faults from natural reservoirs in the Colorado Plateau, east-central Utah. In: Baines SJ, Worden RH (eds) Geological storage of carbon dioxide, vol 233. Geological Society of London, Special Publications, pp 43–58
Sigurdsson H, Devine JD, Tchoua FM, Presser TS, Pringle MKW, Evans WC (1987) Origin of the lethal gas burst from Lake Monoun, Cameroun. J Volcanol Geotherm Res 31:1–16
Smith LK, Lewis WM, Chanton JP, Cronin G, Hamilton SK (2000) Methane emissions from the Orinoco River floodplain, Venezuela. Biogeochemistry 51:113–140
Spycher N, Pruess K (2004) CO2−H2O mixtures in the geological sequestration of CO2. II. Partitioning in chloride brines at 12–100°C and up to 600 bar. Lawrence Berkeley National Laboratory Report LBNL-56334
Spycher N, Ennis-King J, Pruess K (2003) CO2-H2O mixtures in the geological sequestration of CO2. I. Assessment and calculation of mutual solubilities from 12 to 100°C and up to 600 bar. Geochemica et Cosmochimica Acta 67:3015–3031
Torp TA, Gale J (2004) Demonstrating storage of CO2 in geological reservoirs: The sleipner and SACS projects. Energy 29(9–10):1361–1369
Walter BP, Heimann M (2000) A process-based, climate sensitive model to derive methane emissions from natural wetlands: Application of five wetland sites, sensitivity to model parameters, and climate. Global Biogeochem Cycles 14:745–765
Wan J, Veerapaneni S, Gadelle F, Tokunaga TK (2001) Generation of stable microbubbles and their transport through porous media. Water Resour Res 37(5):1173–1182
Washburn L, Johnson C, Gotschalk CC, Egland ET (2001) A gas-capture buoy for measuring bubbling gas flux in oceans and lakes. J Atm Ocean Technol 18:1411–1420
Wilson JO, Crill PM, Bartlett KB, Sebacher DI, Harriss RC, Sann RL (1989) Seasonal variation of methane emissions from a temperate swamp. Biogeochemistry 8:55–71
Acknowledgements
The authors thank Nic Spycher and Karsten Pruess (LBNL) for helpful discussions and constructive comments and reviews, and Scott Imbus and Dan Kieke (Chevron) for support and encouragement. This work was supported in part by a Cooperative Research and Development Agreement (CRADA) between BP Corporation North America, as part of the CO2 Capture Project (CCP) of the Joint Industry Program (JIP), and the U.S. Department of Energy through the National Energy Technologies Laboratory (NETL), and by the Ernest Lawrence Berkeley National Laboratory, managed for the U.S. Department of Energy under Contract No. DE-AC03-76SF00098.
Author information
Authors and Affiliations
Corresponding author
Appendices
Appendix A
Equations for bubble rise in porous media from Corapcioglu et al. (2004). The equations governing bubble rise in coarse porous media are derived by balancing forces due to buoyancy given by
the surface tension force given by
where R′ is an equivalent pore throat radius, and the drag force given by
(variables are defined in Nomenclature). The first term in brackets in Eq. 9 is the Kozeny term, accounting for viscous drag in laminar flow, while the second term is the Burke–Plummer term, accounting for turbulent losses. Summing these three forces and allowing for acceleration of the bubble, we have the balance relation
where the A d term accounts for entrained liquid ahead of the bubble and is defined as
Substituting the individual force equations and grouping terms by the powers of bubble rise velocity (u b), we obtain
where
The rise velocity (u b) can be calculated using the coefficients of Eqs. 13–15 in the quadratic equation (12) for which we assume steady state and zero inertia, i.e., right-hand side of Eq. 12 is set to zero.
Appendix B
Equations for ebullition and diffusion rates are given from Morel and Herring (1993). For each species, the rate of ebullition E i (mol cm−2 s−1) is proportional to its partial pressure at the sediment surface:
where E is the total rate of ebullition of all species together. A steady-state mass balance equation is written for each species at the sediment surface where the sum of its transport by diffusion and ebullition is equal to its rate of formation at depth:
where the subscripts b and s refer to the bottom and surface concentrations (mol cm−3), respectively, and K i is the Henry’s law constant for each gas species (mol cm−3atm−1), and the bottom air flux is assumed to be zero. The diffusive flux is assumed to be driven by the concentration gradient across the entire depth of the surface-water body.
The aqueous concentrations of species at the surface are calculated to be in equilibrium with the atmosphere at 10°C, where \(P_{{\rm CO_{2}}}^{{\rm atm}}\), P atmair , and \(P_{{\rm CH_{4}}}^{{\rm atm}}\) are 3.12×10−4, 1.97×10−6, and 9.87×10−1 atm, respectively (see Table 4 for K i values):
Unknowns in the mass balance equations are now bottom concentrations and E, while the pressure condition at the sediment surface is:
\(P_{{\rm H_{2}O}}\) in Eq. 23 can be neglected relative to the other volatile components and substitute Henry’s law expressions to obtain
where K i values are for the P z considered (Table 4). With the approximations [CO2]s << [CO2]b and [CH4]s << [CH4]b, bottom concentrations from Eqs. 17–19 are substituted into Eq. 24 to yield:
By neglecting the first term in Eq. 25 and replacing \(K_{{\rm CH_{4}}}\) and K air with an average K value, an approximate solution for E can be obtained:
Substitution of Eq. 26 into Eqs. 17 –19 gives the bottom concentrations of species:
The diffusive and ebullition fluxes, F D and F E, respectively, of CO2 and CH4 can then be calculated:
Rights and permissions
About this article
Cite this article
Oldenburg, C.M., Lewicki, J.L. On leakage and seepage of CO2 from geologic storage sites into surface water. Environ Geol 50, 691–705 (2006). https://doi.org/10.1007/s00254-006-0242-0
Received:
Accepted:
Published:
Issue Date:
DOI: https://doi.org/10.1007/s00254-006-0242-0